NCBI Bookshelf. A service of the National Library of Medicine, National Institutes of Health.
Varki A, Cummings RD, Esko JD, et al., editors. Essentials of Glycobiology [Internet]. 3rd edition. Cold Spring Harbor (NY): Cold Spring Harbor Laboratory Press; 2015-2017. doi: 10.1101/glycobiology.3e.013
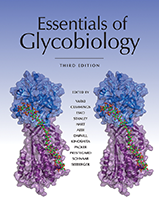
Essentials of Glycobiology [Internet]. 3rd edition.
Show detailsThis chapter focuses on several less easily categorized types of glycoprotein linkages that occur on specific proteins or domains, most of which have specific biological functions. O-linked modifications of epidermal growth factor (EGF)-like repeats (O-fucose, O-glucose, and O-GlcNAc) regulate the Notch signaling pathway. O-Fucosylation of thrombospondin type 1 repeats (TSRs) is required for folding of these domains in a number of secreted matricellular proteins. O-Mannosylation of α-dystroglycan is essential for interactions with several extracellular matrix proteins. Defects in the glycosyltransferases that add these glycans (O-fucose, O-glucose, O-GlcNAc, and O-mannose) result in human diseases. C-Mannosylation is a unique form of glycosylation in which mannose is linked through a carbon–carbon bond to tryptophan. O-Linked galactose disaccharides are added to hydroxyproline and hydroxylysine residues and play an important role in collagen fibril formation. Although the glycans described here are found on relatively few glycoproteins, they play specific and important roles in biology.
DISCOVERY OF NOVEL TYPES OF GLYCOSYLATION
In glycoproteins, the linkage between the first sugar of a glycan and the protein, defines its glycosylation class. In Chapter 1, Figure 1.7 shows 32 known types of glycan–protein linkages, including the common GlcNAc-N-Asn, GalNAc-O-Ser/Thr, and Xyl-O-Ser present in glycoproteins and proteoglycans. The first indication of the existence of novel, nonclassical types of glycosylation came from the analysis of human urine, which revealed unusual amino acid glycosides such as Glcβ1–3Fucα-Thr. This discovery garnered little interest at the time. However, finding O-fucose directly linked to various clotting proteins and signaling receptors, such as Notch, broadened this perspective. Monoclonal antibodies that detected glycans on specific proteins such as α-dystroglycan provided tools to identify other novel glycans. In addition, mass spectrometry of proteins revealed unusual modifications, such as mannose linked to protein as a C-glycoside. Table 13.1 describes many of the less common linkages synthesized in the endoplasmic reticulum (ER)–Golgi pathway. Chapters 18 and 19 describe the very few known glycosylation linkages synthesized in the nucleus and cytoplasm.
TABLE 13.1.
Other classes of eukaryotic glycoprotein glycosylation
O-LINKED MODIFICATIONS IN EPIDERMAL GROWTH FACTOR–LIKE REPEATS
Epidermal growth factor (EGF)-like repeats are small protein domains (∼40 amino acids) that are defined by six conserved cysteine residues, which form three disulfide bonds (Figure 13.1A). They are found in a few hundred cell-surface and secreted proteins in metazoans and, depending on their sequence, may be modified with the less common O-glycans described above. Proteins with these O-glycans attached to EGF-like repeats include several involved in blood clot formation and dissolution, and the Notch family of receptors and canonical Notch ligands (Delta and Serrate/Jagged) involved in embryogenesis and cell fate decisions. The glycan modifications are important because they regulate signal transduction during early development, cell differentiation, and the growth of several cancers.
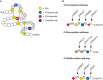
FIGURE 13.1.
Modifications of epidermal growth factor (EGF)-like repeats. (A) EGF-like repeats can be modified by O-fucose, O-glucose, and O-GlcNAc. A schematic representation of an EGF-like repeat is shown: (yellow) conserved cysteine residues; (gray lines between (more...)
O-Fucose Glycans
The α-linked O-fucose modification immediately precedes the third conserved cysteine of certain EGF-like repeats (Figure 13.1A). The consensus motif for O-fucosylation is C2X4(S/T)C3, in which C2 and C3 are the second and third conserved cysteines of the EGF-like repeat. Nearly 100 proteins in mouse or human databases contain this sequence. O-Fucose can be elongated to a tetrasaccharide (Siaα2–3/6Galβ1-4GlcNAcβ1-3Fuc-O-Ser/Thr) in certain contexts (e.g., EGF1 from human clotting factor IX and EGF12 from mouse NOTCH1) (Table 13.1), but not in others (e.g., EGF1 from human clotting factor VII and EGF5 from mouse NOTCH1).
Protein O-fucosyltransferase 1 (POFUT1) transfers fucose from GDP-Fuc to a properly folded EGF-like repeat containing the appropriate consensus sequence (Figure 13.1B). O-Fucose can be elongated by a β1-3 N-acetylglucosaminyltransferase (β3GnT) that is specific for O-fucose residues in properly folded EGF-like repeats. The gene for the O-fucose-specific β3GnT was originally identified in Drosophila as a modifier of Notch signaling called fringe (see below). There are three mammalian homologs: Manic fringe (MFNG), Lunatic fringe (LFNG), and Radical fringe (RFNG). Each of the Fringe proteins catalyzes the transfer of GlcNAc from UDP-GlcNAc to O-fucose on an EGF-like repeat. The GlcNAc can be further elongated by a β4GalT (e.g., B4GALT1) and capped with a sialic acid, probably using the same sialyltransferases as those that act on N- or O-glycans (Siaα2-3/6SiaTs). Elongation past the disaccharide has not been observed to date on Drosophila Notch, although a branched O-fucose trisaccharide (GlcUAβ1-4[GlcNAcβ1-3]Fucitol) has been detected in a glycomics analysis of O-glycans released from proteins extracted from Drosophila embryos.
The O-fucose glycans play important roles in the Notch signal transduction pathway. Notch was originally identified in Drosophila, and homologs have been found in all metazoans, with four Notch receptors in mammals. Activation of Notch signal transduction is controlled at numerous levels, and dysregulation of Notch signaling results in a number of human diseases, including several types of cancer and a variety of developmental disorders. Two classes of canonical ligands bind to and activate Notch in Drosophila: Delta and Serrate. Mammals have three Delta-like homologs (DLL1, DLL3, and DLL4), and two Serrate homologs called Jagged 1 and 2 (JAG1 and JAG2). These ligands are single-pass transmembrane glycoproteins that bind to and activate Notch receptors on an adjacent cell. The extracellular domain of Notch contains up to 36 tandem EGF-like repeats (Figure 13.2), many of which contain consensus sites for O-fucosylation (Figure 13.1A).
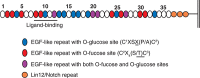
FIGURE 13.2.
Extracellular domain of Notch showing the numerous sites for O-fucose and O-glucose modification that are evolutionarily conserved in Drosophila Notch, mouse NOTCH1 and NOTCH2, and human NOTCH1 and NOTCH2. Epidermal growth factor (EGF)-like repeats directly (more...)
Elimination of POFUT1 in Drosophila or mice results in embryonic lethality with strong Notch developmental defects, indicating that O-fucose modification of Notch is essential for Notch function. Heterozygous mutations in the human POFUT1 gene cause a rare autosomal-dominant skin discoloration and symptoms of Dowling–Degos disease type 2 (DDD2; MIM 615327). POFUT1 is localized to the ER, where it only modifies properly folded EGF-like repeats, suggesting that O-fucosylation may have some role in quality control. The Drosophila homolog of POFUT1 has chaperone activity and is proposed to play a role in the proper folding of Notch. However, loss of POFUT1 has only minor effects, if any, on the trafficking of Notch receptors to the surface of mammalian cells. Structural studies reveal that the O-fucose on EGF12 directly participates in ligand binding, and elimination of this O-fucose glycosylation site by mutation in mouse NOTCH1 reduces Notch activity.
O-Fucose glycans also modulate Notch activity during development. The expression of O-fucose-specific Fringe enzymes is dynamic, increasing or decreasing depending on developmental stage or tissue. Mutations in Fringe show phenotypes in several, but not all, contexts in which Notch functions. Elimination of Fringe in Drosophila causes defects in wing, eye, and leg development, whereas ablation of Lfng in mice causes a severe defect in somite formation. Mutations in human LFNG result in malformed vertebrae and ribs (spondylocostal dysostosis type 3; MIM 609813). Fringe enzymes mediate their effects on Notch activity by transferring GlcNAc to O-fucose on Notch receptors (Figure 13.1B). Surprisingly, Fringe reduces Notch activation by Serrate/Jagged ligands, but it potentiates Notch activation by Delta ligands (Figure 13.3). A major question is how. Presumably the multiple Notch EGF-like repeats bearing O-fucose play a role (Figure 13.2). Fringe modification of some of these may activate signaling from Delta ligands, whereas modification of others may inhibit signaling from Serrate/Jagged. For instance, Fringe-mediated elongation of O-fucose in EGF12 of the ligand-binding domain clearly enhances the affinity between Notch and Delta ligands, but how Fringe inhibits activation from Serrate/Jagged ligands is less clear. One possibility is that the addition of GlcNAc by Fringe causes a conformational change in the extracellular domain of Notch that reduces activation by Serrate/Jagged. The fact that Fringe is a glycosyltransferase that modifies O-fucose residues on Notch provides one of the clearest examples to date of how a signal transduction pathway may be regulated by altering the glycosylation of a receptor.
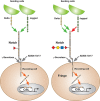
FIGURE 13.3.
Notch signaling pathway. Notch exists on the cell surface as a heterodimer in which the extracellular domain is tethered to the transmembrane and intracellular domain by noncovalent, calcium-dependent interactions. Notch is activated by ligand (members (more...)
O-Glucose Glycans
The β-linked O-glucose modification occurs between the first and second conserved cysteines of EGF-like repeats at the consensus sequence C1XSX(P/A)C2 (Figure 13.1A), which is found in approximately 50 proteins in mouse or human databases. The O-glucose glycan typically exists as the trisaccharide Xylα1–3Xylα1–3Glcβ-O-Ser, although mono- and disaccharide forms are also seen. The human gene encoding the protein O-glucosyltransferase is POGLUT1, rumi in flies. Mutations in POGLUT1/rumi cause Notch-deficient developmental defects in flies and mice, although in mice, O-glucosylation appears to be important for targets in addition to Notch. Similar to POFUT1, heterozygous mutations in human POGLUT1 cause an autosomal-dominant disease termed Dowling–Degos disease type 4 (DDD4; MIM 615696). O-Glucosylation does not appear to affect Notch binding to canonical ligands but is required for optimal Notch activation. Like POFUT1, POGLUT1 is localized to the ER and requires a properly folded EGF-like repeat as substrate. The genes encoding the xylosyltransferases are glucoside α1-3 xylososyltransferase (GXYLT1 and GXYLT2) and xyloside α1-3 xylosyltransferase (XXYLT1) (Figure 13.1B). Elongation of O-glucose with xylose has an inhibitory effect on Notch activity in flies.
O-GlcNAc Glycans
Although β-linked O-GlcNAc is very common in the nuclear and cytosolic compartments (Chapter 19), the same modification can be found in membrane and secreted proteins occurring between the fifth and sixth conserved cysteines of an EGF-like repeat at the putative consensus C5XXGX(S/T)GXXC6 (Figure 13.1A) in mouse and Drosophila Notch. However, sites have been mapped on only a few proteins. The O-GlcNAc can be elongated by Gal and Sia in mammals, putatively to form Siaα2/3-6Galβ1-4GlcNAc, a structure not yet confirmed. The gene encoding the EGF-specific O-GlcNAc transferase, EOGT, is distinct from OGT, the GlcNAc-transferase that modifies nuclear and cytoplasmic proteins (Chapter 19). Inactivation of Drosophila Eogt is pupal lethal and causes a wing-blistering defect if deleted in the wing. Although genetic interactions between Eogt and Notch pathway genes occur in Drosophila, no obvious Notch-deficient phenotypes are observed. However, mutations in human EOGT result in skin and skeletal problems in AOS (Adams–Oliver syndrome type 4; MIM 615297), a disorder with links to the Notch pathway.
MODIFICATIONS OF THROMBOSPONDIN TYPE 1 REPEATS
O-Fucose is also found in thrombospondin type 1 repeats (TSRs). Similar to EGF-like repeats, TSRs are small protein domains (50–60 amino acids) with six conserved cysteines that form three disulfide bonds (Figure 13.4). Like EGF repeats, TSRs are found in a subset of cell-surface and secreted proteins in metazoans, and they appear to function in protein–protein interactions. The O-fucose site occurs between the first and second conserved cysteine residues at the putative consensus sequence C1X2(S/T)C2X2G in TSRs in approximately 50 proteins (Table 13.1). The O-fucose on TSRs can be elongated to form the disaccharide Glcβ1-3Fucα-O-Ser/Thr first observed nearly 30 years ago in glycosides from human urine. The gene encoding the enzyme that fucosylates TSRs is POFUT2. It is expressed in many cells and tissues and is distinct from POFUT1. Elimination of Pofut2 in mice results in early embryonic lethality with defects in gastrulation. The gene encoding the β3-glucosyltransferase that elongates O-fucose on TSRs is B3GLCT. Mutations in B3GLCT in humans results in a multifaceted severe developmental disorder known as Peters-plus syndrome (MIM 261540). TSRs are also often modified with C-mannose (Figure 13.4; see below), although the relationship between the two modifications is not yet clear. A number of biologically interesting proteins are known or predicted to contain this modification, including thrombospondin-1 and -2, all ADAMTS (a disintegrin and metalloproteinase with thrombospondin motifs; ADAMTS 1–20) and ADAMTS-like proteins, properdin, and F-spondin (Table 13.1). O-Fucosylation of TSRs in several proteins including ADAMTS 13 and ADAMTS-like 1 is needed for their secretion, suggesting that O-fucosylation of TSRs may be involved in quality control or folding, similar to the predicted role of O-fucose on EGF-like repeats in Drosophila. Like POFUT1, POFUT2 is also an ER-localized enzyme that only fucosylates properly folded TSRs. Recent studies propose that both POFUT2 and B3GLCT function in a novel ER quality control pathway required for proper folding of TSRs. In thrombospondin-1, the region of the TSR modified with O-fucose mediates interactions between TSRs and several cell-surface receptors. Thus, the presence of O-fucose in this region could influence interactions between TSRs and adjacent cells, much as O-fucose on EGF-like repeats regulates Notch ligand binding. TSRs allow proteins like the thrombospondins to bind to cells and/or to other components in the extracellular matrix. Cell binding is an essential step in the biological roles of thrombospondins, including the antiangiogenic functions of these proteins.
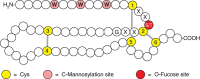
FIGURE 13.4.
Modifications of thrombospondin type-1 repeats (TSRs). These repeats have six conserved cysteines (yellow), three disulfide bonds (gray lines), and consensus sites for O-fucose (red) and C-mannose (pink) addition. W, tryptophan; S, serine; T, threonine; (more...)
O-MANNOSE GLYCANS
O-Mannose α-linked to protein was first identified in yeast in the 1950s (Chapter 23). Fungi elongate this mannose with a battery of mannosyltransferases to form extensions similar to those on their N-glycans. O-Mannose was first identified in mammals in 1979, linked to a rat brain proteoglycan. O-Mannose glycans account for up to one-third of all O-glycans in some mammalian tissues, including brain. O-Mannose glycans in mammals are quite varied and belong to three core classes (Figure 13.5). Defects in O-mannosylation of the protein α-dystroglycan, the major extracellular glycoprotein component of the dystrophin–glycoprotein complex, result in multiple forms of congenital muscular dystrophy (CMD), known as the secondary dystroglycanopathies that include Walker–Warburg syndrome, muscle–eye–brain disease, Fukuyama congenital muscular dystrophy, and limb-girdle muscular dystrophy (Chapter 45). Some of the O-mannose glycans on α-dystroglycan serve as binding sites for extracellular matrix proteins that contain laminin globular domains. These same O-mannose glycans function as a receptor for certain arenaviruses, and the loss of these glycans is also associated with metastasis in multiple cancers.
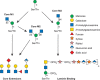
FIGURE 13.5.
Biosynthetic pathway for O-mannose glycans. The O-mannose glycans belong to three core classes depending on extension from the protein-linked mannose by a sole β1-2GlcNAc (core M1), by two GlcNAcs in β1-2 and β1-6 linkages (core (more...)
The initiating O-mannosyltransferase unique to this pathway is common to all three core classes (Figure 13.5). A heterodimer composed of protein O-mannosyltransferase 1 and 2 (POMT1 and POMT2) catalyzes the addition of O-Man to Ser/Thr residues of proteins in the ER using dolichol-P-mannose as the donor. Core M1 O-mannose glycans (Figure 13.5) are then generated by the action of a Golgi-localized N-acetylglucosaminyltransferase (POMGNT1) that adds β1-2GlcNAc to O-mannose on glycoproteins. Core M1 glycans can be further elaborated by enzymes that also act in other pathways, including galactosyltransferases, fucosyltransferases, glucuronyltransferases, and sialyltransferases. Core M1 glycans can also be converted to Core M2 O-mannose glycans by the addition of a β1-6GlcNAc to the O-Man, catalzyed by the N-acetylglucosaminyltansferase MGAT5B. This new branch on core M2 glycans can also be extended by the glycosyltransferases that act on core M1 (Figure 13.5 core extensions). Core M1 and M2 O-Man glycans have been identified on mammalian proteins besides α-dystroglycan, including cadherins.
The core M3 glycans (Figure 13.5) possess key functional properties and have to date been identified only on the mammalian protein α-dystroglycan. Core 3 O-mannose is extended in the ER by an N-acetylglucosaminyltransferase (POMGNT2) that adds a β1-4GlcNAc. This disaccharide is a substrate for an N-acetylgalactosaminyltransferase (B3GALNT2) that adds β1-3GalNAc to the GlcNAc. This trisaccharide is a substrate for a secretory pathway kinase (POMK) that adds a 6-linked phosphate to the core Man. The immediate next steps are the addition of a ribitol-5-P in a phosphodiester linkage to the GalNAc catalyzed by fukutin (FKTN) followed by the addition of another ribitol-5-P in a phosphodiester linkage to the first ribitol by fukutin-related protein (FKRP). Isoprenic synthase domain protein (ISPD) generates the sugar nucleotide CDP-ribitol that FKTN and FKRP use. TMEM5 and B4GAT1 glycosyltransferases add a xylose and glucuronic acid, respectively, to the outermost ribitol. This action serves to prime the core M3 glycan for the addition of a repeating disaccharide (Xyl-α3-GlcA-β3-)n that is catalyzed by the dual activity LARGE glycosyltransferase. This repeating disaccharide, termed matriglycan, appears to be the binding site for extracellular matrix proteins containing laminin globular domains, as well as the receptor for certain arenaviruses.
O-GLYCANS IN COLLAGENS
Proteins with collagen domains are modified by a disaccharide, Glcα1-2Galβ-, which is assembled on hydroxylysine or hydroxyproline residues. First recognized on collagen in the 1960s, this O-glycan has been observed in other proteins that have collagen-like modules, including adiponectin and pulmonary surfactants proteins. Primitive forms of collagen in sponges and sea anemones also have disaccharide-modified hydroxylysine. The first step in the pathway is the hydroxylation of either lysine or proline to hydroxylysine or hydroxyproline via the appropriate hydroxylases. The two-step glycosylation rapidly follows on most of the available acceptor sites. Interestingly, although the hydroxylation and addition of Gal are conserved in all animals species examined, and occur by the action of two separate enzymes, giant viruses have one bifunctional enzyme capable of hydroxylating lysine and adding Glc instead of Gal to collagen. Glycosylation is thought to proceed until the protein folds and assembles into the well-known collagen triple helix. Some studies suggest that the extent of glycosylation controls or influences the rate of triple-helix formation and, in turn, the size of collagen fibrils. Other studies suggest that excessive glycosylation of hydroxylysines makes them poor substrates for extracellular enzymatic deamination, the first step in cross-linking collagen. Mice lacking one of the lysyl hydroxylases (LH3) fail to glycosylate collagen IV properly, which causes embryonic lethality, and deposition of misfolded collagen in the ER.
C-MANNOSYLATION
Another novel type of glycosylation, first detected in human urine, is called C-mannosylation. The C-1 atom of a single Man residue is added in α-linkage to the C-2 atom of the indole moiety of tryptophan 7 (Trp7) in RNase 2 (Figure 13.6). Note that this is a rare glycosidic linkage; it is a C–C bond rather than a C–O or C–N bond. Structural analysis, biosynthetic studies in mammalian cell lines, and a specific antibody against the modification, show that C-mannosylation is widespread, but it appears to be absent in bacteria and yeast. Site-directed mutagenesis shows that the critical glycosylation sequence in RNase 2 is W-X-X-W-, with the first Trp being modified. Synthetic peptides with this motif can be C-mannosylated in vitro, and more than 300 mammalian cell proteins have this sequence. Several complement proteins contain TSRs with C-mannose (Figure 13.4). For instance, in properdin (which is a positive regulator of complement), 14 of 17 consensus sites are fully modified. Dolichol-P-mannose produced from GDP-Man (Chapter 5) is the biosynthetic donor for C-mannosylation in the ER, and a gene encoding a C-mannosyltransferase has been identified in Caenorhabditis elegans (C. elegans) (DPY-19). Mutants in DPY-19 cause a neuroblast migration defect. The modification appears to either compete with, or aid in, protein folding because already folded proteins are poor acceptor substrates in vitro, and expression of some proteins in C-mannosylation-disabled cells leads to their accumulation in the ER.
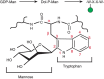
FIGURE 13.6.
Biosynthetic pathway for C-mannosylation and structural details of tryptophan-7 in RNase 2.
ACKNOWLEDGMENTS
The authors appreciate helpful comments and suggestions from Dustin Middleton, Ryan Porell, and Corinna Landig.
FURTHER READING
- Furmanek A, Hofsteenge J. 2000. Protein C-mannosylation: Facts and questions. Acta Biochim Pol 47: 781–789. [PubMed: 11310977]
- Martin PT. 2007. Congenital muscular dystrophies involving the O-mannose pathway. Curr Mol Med 7: 417–425. [PMC free article: PMC2855644] [PubMed: 17584082]
- Stanley P, Okajima T. 2010. Roles of glycosylation in Notch signaling. Curr Top Dev Biol 92: 131–164. [PubMed: 20816394]
- Ogawa M, Furukawa K, Okajima T. 2014. Extracellular O-linked β-N-acetylglucosamine: Its biology and relationship to human disease. World J Biol Chem 5: 224–230. [PMC free article: PMC4050115] [PubMed: 24921011]
- Praissman JL, Wells L. 2014. Mammalian O-mannosylation pathway: Glycan structures, enzymes, and protein substrates. Biochemistry 53: 3066–3078. [PMC free article: PMC4033628] [PubMed: 24786756]
- Takeuchi H, Haltiwanger RS. 2014. Significance of glycosylation in Notch signaling. Biochem Biophys Res Commun 453: 235–242. [PMC free article: PMC4254162] [PubMed: 24909690]
- Haltom AR, Jafar-Nejad H. 2015. The multiple roles of epidermal growth factor repeat O-glycans in animal development. Glycobiology 25: 1027–1042. [PMC free article: PMC4551148] [PubMed: 26175457]
- Vasudevan D, Takeuchi H, Johar SS, Majerus E, Haltiwanger RS. 2015. Peters plus syndrome mutations disrupt a noncanonical ER quality-control mechanism. Curr Biol 25: 286–295. [PMC free article: PMC4318717] [PubMed: 25544610]
- Yoshida-Moriguchi T, Campbell KP. 2015. Matriglycan: A novel polysaccharide that links dystroglycan to the basement membrane. Glycobiology 25: 702–713. [PMC free article: PMC4453867] [PubMed: 25882296]
- Review Other Classes of Eukaryotic Glycans.[Essentials of Glycobiology. 2022]Review Other Classes of Eukaryotic Glycans.Haltiwanger RS, Wells L, Freeze HH, Jafar-Nejad H, Okajima T, Stanley P. Essentials of Glycobiology. 2022
- Review Protein O-fucosylation: structure and function.[Curr Opin Struct Biol. 2019]Review Protein O-fucosylation: structure and function.Holdener BC, Haltiwanger RS. Curr Opin Struct Biol. 2019 Jun; 56:78-86. Epub 2019 Jan 26.
- O-Fucosylation of ADAMTSL2 is required for secretion and is impacted by geleophysic dysplasia-causing mutations.[J Biol Chem. 2020]O-Fucosylation of ADAMTSL2 is required for secretion and is impacted by geleophysic dysplasia-causing mutations.Zhang A, Berardinelli SJ, Leonhard-Melief C, Vasudevan D, Liu TW, Taibi A, Giannone S, Apte SS, Holdener BC, Haltiwanger RS. J Biol Chem. 2020 Nov 13; 295(46):15742-15753. Epub 2020 Sep 10.
- Review The multiple roles of epidermal growth factor repeat O-glycans in animal development.[Glycobiology. 2015]Review The multiple roles of epidermal growth factor repeat O-glycans in animal development.Haltom AR, Jafar-Nejad H. Glycobiology. 2015 Oct; 25(10):1027-42. Epub 2015 Jul 14.
- Two distinct pathways for O-fucosylation of epidermal growth factor-like or thrombospondin type 1 repeats.[J Biol Chem. 2006]Two distinct pathways for O-fucosylation of epidermal growth factor-like or thrombospondin type 1 repeats.Luo Y, Nita-Lazar A, Haltiwanger RS. J Biol Chem. 2006 Apr 7; 281(14):9385-92. Epub 2006 Feb 7.
- Other Classes of Eukaryotic Glycans - Essentials of GlycobiologyOther Classes of Eukaryotic Glycans - Essentials of Glycobiology
Your browsing activity is empty.
Activity recording is turned off.
See more...