NCBI Bookshelf. A service of the National Library of Medicine, National Institutes of Health.
Varki A, Cummings RD, Esko JD, et al., editors. Essentials of Glycobiology [Internet]. 3rd edition. Cold Spring Harbor (NY): Cold Spring Harbor Laboratory Press; 2015-2017. doi: 10.1101/glycobiology.3e.042
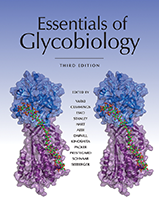
Essentials of Glycobiology [Internet]. 3rd edition.
Show detailsThis chapter illustrates and discusses some key mechanisms by which glycans influence the pathogenesis and progession of bacterial and viral infections and describes examples of opportunities for therapeutic intervention.
BACKGROUND
Infectious diseases remain a major cause of death, disability, and social and economic disorder for millions of people throughout the world. Poverty, poor access to health care, human migration, emerging disease agents, and antibiotic resistance all contribute to the expanding impact of these illnesses. Prevention and treatment strategies for infectious diseases are derived from a thorough understanding of the complex interactions between specific viral or bacterial pathogens and the human (or animal) host.
Just as glycans are major components of the outermost surface of all animal and plant cells, so too are oligosaccharides and polysaccharides found on the surfaces of all bacteria and viruses of eukaryotes. Thus, most (if not all) interactions of microbial pathogens with their hosts are influenced to an important degree by the pattern of glycans and glycan-binding receptors that each expresses. This holds true at all stages of infection, from initial colonization of host epithelial surfaces, to tissue spread, to the induction of inflammation or host-cell injury that results in clinical symptoms. The microbial molecules most responsible for disease manifestations are known as virulence factors.
BACTERIAL SURFACE GLYCANS AS VIRULENCE FACTORS
Polysaccharide Capsules
A human in good health is colonized by as many as 1013 bacteria on the skin and mucosal surfaces, particularly in the gastrointestinal tract, a number that equals the number of cells in our own body. Despite all these direct encounters, only a handful of bacterial species are known to spread into the body to produce serious infections. Although not restricted to pathogenic species, one feature that most of these disease-causing agents share in common is the presence of a polysaccharide capsule that covers the bacterial surface (Chapter 21). Capsule expression by the bacteria poses a particular challenge to immune detection and clearance.
Effective killing of bacteria by phagocytes, such as neutrophils or macrophages, requires opsonization, a process in which the bacterial surface is tagged with complement proteins or specific antibodies. Phagocytes express receptors for activated complement or antibody Fc domains, which allow host defense cells to bind, engulf, and kill the bacteria. Anionic host mimics, such as sialic acid present in bacterial surface capsules like those of the neonatal pathogens, group B streptococcus (GBS), and Escherichia coli K1, can bind the host regulatory protein factor H, thereby attenuating the activity of the alternative complement pathway. Through their surface polysaccharide capsules, bacteria also cloak protein structures on their surfaces to which antibodies might be directed.
Generally, humans can generate good antibody responses against nonsialylated bacterial polysaccharide capsules, but this ability is diminished early and late in life, so that infants and the elderly are particularly prone to invasive infection with encapsulated pathogens. Certain bacteria avoid antibody defenses through molecular mimicry of common host glycan structures, masquerading as “self” to avoid immune recognition. An example is the leading pathogen, group A streptococcus (GAS), which expresses a nonimmunogenic capsule of hyaluronan, identical to the nonsulfated glycosaminoglycan that is highly abundant in host skin and cartilage (Chapter 16). The contribution of capsule-based host mimicry to bacterial immune evasion is also well illustrated by the homopolymeric sialic acid capsules of Neisseria meningitidis (meningococcus), an important cause of sepsis and meningitis. Whereas the group C meningococcal capsule is composed of an α2-9-linked sialic acid polymer that is a unique bacterial structure, the group B meningococcal capsule is composed of an α2-8-linked sialic acid polymer that is identical to a motif present on neural cell adhesion molecules (NCAMs) found in human neural tissues (Chapter 15). The group C capsule has proven to be a successful vaccine antigen in human populations, whereas the group B capsule is essentially nonimmunogenic.
Another challenge posed to host immunity by certain pathogens is the great diversity of capsular structures, which is reflected in the different compositions and linkages of repeating sugar units that are produced by different strains of the same bacterial species. Often, these structures are immunologically distinct, allowing classification of different capsule “serotype” strains; for example, there are five major capsule serotypes of meningococcus (A, B, C, Y, and W-135), six different capsule serotypes of the respiratory pathogen Haemophilus influenzae (a–f), nine capsule serotypes of GBS (Ia, Ib, and II–VIII), and more than 90 different serotypes of Streptococcus pneumoniae (pneumococcus), which is a leading cause of bacterial pneumonia, sepsis, and meningitis. Antibodies generated by the host against the capsule of one serotype strain typically do not provide cross-protective immunity. Thus, individuals can be repeatedly infected over their lifetime by different serotypes of the same bacterial pathogen. Figuratively, although the strategy of capsular molecular mimicry used for example by GAS renders the pathogen invisible to immune surveillance, the strategy of antigenic diversity of capsule types presents a moving target to the immune system. Genetic exchange of capsule biosynthetic genes among serotype strains of an individual species (e.g., the polysialyltransferase gene of meningococcus) can lead to capsule switching in vivo, which provides another means of pathogen escape from protective immunity.
The key role of the capsule in the virulence of multiple bacterial pathogens has been shown through genetic mutagenesis of capsule biosynthesis genes and infectious challenge in small animal models of disease. Compared with the wild-type parent bacterial strains, isogenic capsule-deficient mutants of GAS, GBS, pneumococcus, H. influenzae, meningococcus, Salmonella typhi (typhoid fever), Bacillus anthracis (anthrax), and several other important human pathogens are rapidly cleared from the bloodstream by opsonophagocytosis and they are unable to establish systemic infections. Perhaps the most historically significant bacterial virulence factor of all is the pneumococcal capsule. Pioneering experiments by Frederick Griffith in 1928 showed the transfer of disease-causing capacity of virulent, encapsulated (smooth) strains to nonvirulent, nonencapsulated (rough) strains. Studies regarding immunogenicity of pneumococcal polysaccharides (Figure 42.1) provided the framework for the discoveries of Oswald Avery, Colin MacLeod, and Maclyn McCarty, which showed DNA to be the carrier of genetic information.

FIGURE 42.1.
Classical experiments on the role of the pneumococcal polysaccharide capsule in virulence. Streptococcus pneumoniae (SPN) strains can be identified with either a “rough” (R) or a “smooth” (S) phenotype, the latter being (more...)
Lipopolysaccharide
In addition to a polysaccharide capsule, Gram-negative bacteria have an outer membrane that is rich in lipopolysaccharide (LPS; Chapter 21). LPS contains a lipid A moiety (comprised of two glucosamines, acyl chains and phosphates), which is embedded in the outer membrane, and two additional glycan components that extend outward: a core oligosaccharide containing sugars not found in vertebrates (such as ketodeoxyoctulonate [Kdo] and heptose) and a repeating polysaccharide known as the O-antigen that can vary among strains within an individual species. Many mucosal pathogens such as H. influenzae, Campylobacter jejuni, and Neisseria gonorrhoeae lack O-antigens; instead, they produce lipooligosaccharides (LOSs) that contain only lipid A and an extended core structure.
LPS is a pathogen-associated molecular pattern (PAMP) that is recognized by the innate immune system and stimulates inflammatory responses to clear bacteria that have breeched the barrier defenses of the skin or mucosal epithelium. Soluble LPS released by invading bacteria, and particularly its lipid A component, interacts with the opsonic receptor CD14 and the membrane protein Toll-like receptor 4 (TLR4) to initiate distinct immune signaling processes (Figure 42.2). TLR4 belongs to an evolutionarily conserved family of receptors (TLRs) that can distinguish closely related microbe-derived ligands. For example, the TLR2 receptor can recognize peptidoglycan or lipoteichoic acid derived from the cell walls of Gram-positive bacteria that generally lack LPS. A signaling cascade ultimately leads to the activation of the transcription factor nuclear factor-κB (NF-κB) and its translocation to the nucleus, where it positively regulates the promoters for genes encoding several proinflammatory cytokines, including tumor necrosis factor-α (TNF-α) and Interleukin-1 (IL-1).

FIGURE 42.2.
Activation of immune signaling by bacterial lipopolysaccharide (LPS). LPS from the cell wall of Gram-negative bacteria is bound by the pattern-recognition molecule Toll-like receptor 4 (TLR4) in conjunction with the cell-surface receptor CD14. The binding (more...)
Although TLR-mediated detection of LPS and other microbial molecules is a critical element in triggering host innate immunity, a dangerous condition known as sepsis can develop in the setting of overwhelming bacterial infections that lead to dysregulated immune responses. Symptoms include fever, low blood pressure, rapid heart rate, abnormal white blood cell counts, and dysfunction of multiple organ systems that may lead to lung or kidney failure and death.
Many Gram-negative bacteria vary or modify their LPS to interfere with host immune defense mechanisms. For example, by incorporating modifications such as phosphoethanolamine that can now be attached through plasmid-mediated transferases to confer resistance to polymixins or 4-amino-4-deoxy-L-arabinose (L-Ara4N) that reduce the overall negative charge of LPS, bacteria can repel cationic host antimicrobial peptides, including defensins, away from their cell wall target of action. The plague bacillus Yersinia pestis changes the number and type of acyl groups on the lipid A of its LPS in response to temperature changes. At environmental temperatures (∼21°C), Y. pestis expresses predominantly hexa-acylated lipid A, whereas at the body temperature of the mammalian host (37°C), the pathogen expresses mostly tetra-acylated lipid A. The more complex hexa-acylated version of the LPS strongly induces cytokine release from host cells, which suggests that the production of a less immunostimulatory form of LPS on entry into the mammalian host might represent a virulence mechanism to avoid immune detection.
The foodborne pathogen, C. jejuni, is capable of expressing variable sialylated LOS structures that mimic human gangliosides (Chapters 11 and 46). In most instances, the LOS structures allow the pathogen to evade the immune system. In a small number of cases, however, self-tolerance is overcome and the host recognizes these antigens as foreign, mounting an immune response against the LOS. These self-reacting antibodies then bind to host gangliosides on nerve fibers, which results in a paralytic disorder known as Guillain–Barré syndrome (GBS). For most patients, GBS symptoms can be managed by removing cross-reactive antibodies (plasmapheresis) or immunomodulatory therapies such as intravenous immunoglobulin (IVIg).
MECHANISMS OF COLONIZATION AND INVASION
Adhesins and Receptors
Adherence to skin or mucosal surfaces is a fundamental characteristic of the normal human microflora and also an essential first step in the pathogenesis of many important infectious diseases (Chapter 34). Most microorganisms express more than one type of adherence factor or “adhesin.” A large fraction of microbial adhesins are lectins that bind directly to cell-surface glycoproteins, glycosphingolipids, or glycosaminoglycans; adhesion may be mediated through terminal sugars or internal carbohydrate motifs. In other cases, the bacteria express adhesins that bind matrix glycoproteins (e.g., fibronectin, collagen, or laminin) or mucin, mediating attachment to the mucosal surface. The specific carbohydrate ligands for bacterial attachment on the animal cell are often referred to as adhesin receptors and they are quite diverse in nature. The tropism of individual bacteria for particular host tissues (e.g., skin vs. respiratory tract vs. gastrointestinal tract) is effectively determined by the array of available adhesin-receptor pairs.
In a number of cases, the key adhesive factor is an assembly of protein subunits that project from the bacterial surface in hair-like threads known as pili or fimbriae (Figure 42.3A). Such pili are usually composed of a repeating structural subunit providing extension and a different “tip adhesin” responsible for binding to the host cell. The structural proteins for pilus assembly are often encoded in a bacterial operon. Lateral mobility of pili structures in the bacterial membrane provides a Velcro-like binding effect to epithelial surfaces. Certain strains of E. coli express pili that bind avidly to P-blood group-related glycosphingolipids in the bladder epithelium, leading to urinary tract infection. Pathogenic strains of Salmonella produce pili that facilitate adherence to human intestinal cell mucosa, thereby causing food poisoning and infectious diarrhea. In other cases, a surface-anchored protein (afimbrial adhesin) expressed by the bacteria represents a critical colonization factor (Figure 42.3B). For example, the filamentous hemagglutinin (FHA) of Bordetella pertussis promotes strong attachment of the bacteria to the ciliated epithelial cells of the bronchi and trachea, triggering local inflammation and tissue injury that results in the “whooping cough” disease. FHA is a component of modern pertussis vaccines given in infancy and early childhood to block infection.

FIGURE 42.3.
Examples of mechanisms of bacterial adherence to host-cell surfaces. (A) Pili or fimbriae are organelles that project from the cell surface. They are made up of a repeating structural subunit and a protein at their tip that mediates recognition of a specific (more...)
Adhesins can be glycoproteins as well. In Pasteurellaceae and some H. influenzae species, adhesins are N-glucosylated by a cytoplasmic N-glucosylation system that is homologous to the cytoplasmic O-GlcNAc transferase of eukaryotes. Similarly, transfer of heptose residues by the dodecameric bacterial autotransporter heptosyltransferase (BAHT) family of enzymes to autotransporter adhesins in several different Gram-negative pathogens is essential for the adhesion process.
Invasion Factors
Glycan–lectin interactions play pivotal roles in enabling certain pathogens to penetrate or invade through epithelial barriers, whereupon they may disseminate through the bloodstream to produce deep-seated infections. Salmonella enterica serovar Typhi causes typhoid fever in humans, a process that begins with intracellular invasion of intestinal epithelial cells. The outer core oligosaccharide structure of the LPS is required for internalization in epithelial cells. Removal of a key terminal sugar residue on the outer core markedly reduces the efficiency of bacterial uptake. Once invasion has occurred, the secreted A2B5 typhoid toxin mediates illness by first binding preferentially to the sialic acid Neu5Ac, which is enriched in humans. Streptococcus pyogenes, the common cause of strep throat but also an agent of serious invasive infections, attaches to human pharyngeal and skin epithelial cells through specific recognition of its hyaluronan capsular polysaccharide by the hyaluronan-binding protein CD44 (Chapter 16). This binding process induces marked cytoskeletal rearrangements manifested by membrane ruffling and opening of intercellular junctions that allow tissue penetration by GAS through a paracellular route.
Bacterial glycosylation systems are also involved in the manipulation of the host response: for example, injection of the NleB glycosyltransferase by enteropathogenic E. coli (EPEC) results in the N-GlcNAc modification of arginine residues of host defense proteins, whereas the injected GlcNAc transferase PaTox of Photorhabdus asymbiotica modifies the host Rho GTPase at tyrosine residues.
Biofilms
Biofilm formation is a mechanism that promotes bacterial attachment to host surfaces, often in the form of a polymicrobial community. For example, oral biofilms comprise, in total, approximately 1000 species, only one-half of which are culturable, and the remaining species can only be identified by nucleic acid detection methods. Streptococcus species predominate (60%–90%), but Eikenella, Haemophilus, Prevotella, and Priopionibacterium species can also be found abundantly. Dental plaque represents an oral biofilm in which dense, mushroom-like clumps of bacteria pop up from the surface of the tooth enamel, interspersed with bacteria-free channels filled with nucleic acids, proteins, lipids, and extracellular polysaccharide (EPS) produced by the bacteria that can serve as diffusion channels (Figure 42.4). Bacteria within biofilms communicate with one another through soluble signaling molecules in a process known as “quorum sensing” to optimize gene expression for survival. In biofilms, bacteria live under nutrient limitation and in a dormant state in which defense molecules (e.g., antimicrobial peptides) produced by the immune system and pharmacologic antibiotics are less effective. Moreover, the EPS matrix can bind and inactivate these same agents, contributing to the persistence of the biofilm and difficulty in medical treatment of biofilm infections, such as those that arise on catheters and other medical devices.

FIGURE 42.4.
Structure of a polymicrobial biofilm. Dental plaque is an example of a polymicrobial biofilm in which Streptococcus species and other bacteria secrete a thick exopolysaccharide matrix and exist within this matrix in a dormant or sessile state of low metabolic (more...)
The EPS synthesized by bacteria in biofilms varies greatly in their composition and in their chemical and physical properties. Many EPS types are polyanionic because of the presence of either uronic acids (D-glucuronic, D-galacturonic, or D-mannuronic acids) or ketal-linked pyruvate. Inorganic residues, such as phosphate or sulfate, also contribute to the negative charge and modifications such as O-acetylation or sugar epimerization further contribute to EPS complexity.
In several cases, EPS is a homoglycan composed of β-1,6-linked N-acetylglucosamine residues known as poly-N-acetylglucosamine (or PNAG), as exemplified by the adhesive polymer obtained from Staphylococcus epidermidis strains that produce biofilms on catheters. PNAG is common among many oral pathogens and multidrug-resistant bacteria resulting in concerted efforts to target this polymer, especially because antibodies raised against the deacetylated form of PNAG mediate opsonic killing. Interestingly, the periodontal pathogen Aggregatibacter actinomycetemcomitans secretes a PNAG hydrolase known as dispersin B (DspB) that has been shown to effectively disperse biofilms formed by PNAG-producing bacteria and is therefore also being developed as an adjuvant to antibiotic therapy.
VIRAL INFECTIONS
Viruses bind to host cells as a prerequisite for entry and intracellular replication. Because cell surfaces are especially enriched in glycans, numerous viruses target glycans for cell attachment and entry. Virus–glycan interactions are often responsible for species and tissue tropism (Table 37.1), as illustrated here with examples of three human pathogens: influenza virus, herpes simplex virus 1 (HSV-1), and human immunodeficiency virus (HIV), showing different modes of glycan-mediated viral interactions (Figure 42.5). Influenza virus uses a sialic acid–binding protein for viral binding and entry. HSV-1 has multiple envelope proteins that bind to heparan sulfate as the first step in recruitment of a multiprotein virus entry complex. HIV expresses glycans on its surface that co-opt host lectins to enhance cell dissemination.

FIGURE 42.5.
Mechanisms of viral entry into host cells. (A) Influenza virus initiates host cell contact and entry by binding to cell-surface sialic acid receptors through its surface glycoprotein hemagglutinin. After intracellular replication, a cell-surface neuraminidase (more...)
Influenza Virus
Influenza viruses are common human pathogens of the upper respiratory tract. Seasonal epidemics result in hundreds of thousands of deaths annually, with occasional, much more deadly pandemics. Influenza gains entry into cells of the human upper airway by binding to glycans terminated with α2-6-linked sialic acid (preferentially the human enriched one, Neu5Ac) (Chapter 15). The entry process is mediated by influenza hemagglutinin, named for the method of its discovery. When first isolated in the 1930s, influenza was found to cause clumping (agglutination) of human red blood cells in vitro. Upon continued incubation, the red cells disaggregated and were not re-agglutinated by fresh virus. It was hypothesized that a “receptor destroying enzyme” was responsible. Isolation of the virus-released receptor revealed sialic acid that had been released by a sialidase, also called viral neuraminidase. The familiar numbering system H1N1 represents hemagglutinin (H) and neuraminidase (N; technically a sialidase).
The life cycle of influenza starts with binding of the viral hemagglutinin to cell-surface sialic acids followed by hemagglutinin-mediated fusion with the host cell membrane, release of the virions intracellularly, replication, and then budding of newly assembled virions from the host cell surface. To disseminate to new cells, newly budded virus disengage from the cell surface and viral envelope sialic acids using the viral neuraminidase. The hemagglutinin directs species and tissue tropism, whereas the neuraminidase is essential for propagation of the infection.
Emergence of new human influenza strains occurs via transmission from other animal species, especially poultry, and a key to species tropism is sialic acid linkage. Glycan array screening revealed that avian influenza binds to glycans terminated with α2-3 Neu5Ac, whereas human isolates bind to glycans terminated with α2-6 Neu5Ac. Neu5Ac in α2-3 linkage is common in the intestinal tracts of birds but diminished in the human upper airway, whereas α2-6-linked Neu5Ac predominates in the human upper airway. The switch from α2-3 to α2-6 binding is thought to underlie the emergence of new human influenza strains. This switch can occur by mutation of one or two amino acids in the hemagglutinin sialic acid–binding pocket, and the emergence of α2-6 sialic acid binding is now monitored among animal influenza strains to detect potential new human influenza pathogens. Pigs are susceptible to both α2-3 (avian) and α2-6 (human) viruses, and act as a “mixing vessel” to produce recombinant viruses capable of transmission from birds to humans. Direct avian–human transmission is often associated with enhanced morbidity, but human-to-human spread of avian influenza is uncommon. Notably, ferrets are the most effective animal model for human influenza studies as they have similar upper airway glycans terminated with α2-6 Neu5Ac.
On completing its cellular replication cycle, the release of influenza viruses from infected cell surfaces relies on viral neuraminidase, which removes sialic acid from the surface of the host cells and the virion envelope. Without neuraminidase, newly formed virions stick together, form large rafts, and do not spread to other cells. Two rationally designed influenza virus neuraminidase inhibitor-based anti-influenza drugs, Relenza and Tamiflu, operate on this basis (Chapters 55 and 57).
Several nonenveloped viruses also have sialic acid–binding proteins on their icosahedral capsids including reovirus, adenovirus, parvovirus, and rotavirus, each of which binds to different sets of sialoglycans.
Herpes Simplex Virus
Heparan sulfate (HS) proteoglycans, which are widely distributed on vertebrate cells (Chapter 17), are implicated in the infective process of many pathogenic viruses including adeno-associated viruses, dengue viruses, hepatitis C virus, vaccinia virus, HIV, papillomavirus, and virtually all herpesviruses. In many cases HS is a coreceptor, initiating attachment before recruitment of other host receptor proteins that support viral entry. A prominent example is HSV-1, also known as human herpesvirus 1 (HHV-1), one of the eight currently known human herpesviruses that cause widespread disease.
HSV-1 establishes latent, recurrent infections of mucous membranes, particularly lesions of the mouth and lips (cold sores, fever blisters) but also of genital tract and cornea, the latter of which may cause blindness. Unlike most other viruses, in which host cell binding and entry are mediated by one or two viral proteins, herpesvirus entry requires several viral entry glycoproteins, some of which are shared among all herpesvirus family members. One shared viral glycoprotein, gB, initiates virus attachment by binding to cell-surface HS, as does gC in HSV-1. Once bound, the virus “surfs” the cell surface until it encounters other receptors, including a specific HS structure, 3-O-sulfation on GlcNAc. This relatively rare HS modification induces binding of gD, another glycoprotein shared in the herpesvirus family. Once gD binds, it recruits additional proteins required for fusion and host cell entry.
Removal of HS from cell surfaces enzymatically or by selection of mutant cells defective in HS expression renders the cells resistant to HSV-1 infection by reducing virus attachment. Soluble heparin and HS mimetics inhibit viral infection by masking the HS-binding domain on the virus envelope. Immobilized HS columns bind to the HIV-1 viral entry proteins gB and gC, and HSV-1 deletion mutants lacking gB and gC exhibit impaired virus binding. Genetic evidence of a role of 3-O-sulfation of HS GlcNAc in HSV–1 infection was obtained by altering the expression of 3-O-sulfotransferase genes in cells and living organisms. Recent evidence suggests that induced upregulation of the host's own heparanase helps newly budded HSV-1 to disseminate, analogous to the role of influenza neuraminidase. Although the herpesviruses have evolved much more complex systems for host cell binding and entry, some of which remain to be established, it is clear that HS plays important roles in viral pathogenesis.
Human Immunodeficiency Virus
HIV is a retrovirus and the etiologic agent of the acquired immunodeficiency syndrome (AIDS), a pandemic disease affecting tens of millions of people worldwide. HIV is an enveloped virus with a surface dominated by spikes made of two proteins, gp120 and gp41, of which gp120 mediates viral attachment to host cells, primarily CD4+ T cells, by binding to the host cell-surface receptor CD4 and a chemokine receptor coreceptor such as CCR5 or CXCR4. HIV gp120 is heavily glycosylated, with N-linked glycans comprising half of the spike mass and densely covering much of the spike surface. Dense glycosylation is thought to aid in immune evasion by masking the underlying polypeptide. However, gp120 glycans also actively support infection by co-opting host lectins, including C-type lectins on dendritic cells (Chapter 34).
Dendritic cells (DCs) are innate immune cells that capture and present antigens to T cells to initiate adaptive immunity. DCs capture antigens, in part, using C-type lectins that bind to glycan determinants common to pathogens but uncommon on host cells. Although DCs are not numerous, they are important in recognizing and presenting pathogen antigens to the adaptive immune system. DCs that reside in submucosal tissues of the vagina and rectum are early targets for HIV. Even with low levels of viral exposure, C-type lectins on DCs trap and concentrate HIV for subsequent presentation to T cells, the main site of HIV replication. DC-SIGN (dendritic cell–specific intercellular adhesion molecule-3-grabbing nonintegrin), mannose receptor, and Langerin are some of the C-type lectins that are important for this process. These lectins recognize dense arrays of mannose on pathogens including certain viruses (HIV, CMV [cytomegalovirus], hepatitis C virus, dengue virus), bacteria (Helicobacter, Klebsiella, Mycobacteria), fungi (Candida), and parasites (Leishmania, Schistosoma). Although T cells normally function to destroy pathogens and process their antigens for presentation, lectin-bound HIV evades destruction for extended periods. The natural role of DCs in presentation to T cells makes them ideal conduits for transmission of HIV to CD4+ T cells, where CD4 and cytokine receptors support binding, fusion, and viral replication. This process is termed trans-infection and facilitates early establishment of the HIV infection. DCs are not the only cells co-opted by HIV; macrophages express the same lectins and may also facilitate trans-infection. Other host lectins on DCs and macrophages, such as Siglec-1 (Chapter 35) play a similar role in facilitating uptake of viruses with heavily sialylated envelopes.
GLYCAN-BASED INTERACTIONS BETWEEN HOST AND GUT MICROBIOTA: COMMENSALS AND PATHOGENS
The nature of the relationship between microbes and the human host spans the spectrum from mutually beneficial (symbiotic), to benefiting the microbe without harming the host (commensal), to benefiting the microbe at the expense of the host (pathogenic). Key factors determining the placement of any particular microbe–host interaction along this continuum of potential outcomes are the repertoire of glycans expressed on the host cell surfaces, the mucosal barriers, and the glycan expressing and catabolizing capabilities of the bacteria.
Bacteroides thetaiotaomicron is an anaerobic bacterium that is one of the most abundant members of the normal colonic microbiota in mice and humans. This interesting microbe has evolved mechanisms to establish and maintain a nonpathogenic, mutually beneficial relationship with its mammalian host. A clue to this relationship came from examination of the gut epithelium of mice that are raised under germ-free conditions. Without bacterial exposure, the intestinal epithelium lacks expression of fucosylated glycoconjugates; when normal colonic bacteria are present, Fucα1-2Gal glycan expression is abundant on the surface of these host cells. B. thetaiotaomicron preferentially uses fucose both as an energy source and for incorporation into its own surface capsule and glycoproteins, phenotypes that are required for successful colonization and for proper immune development of the host. When dietary fucose is low, the bacterium induces the expression of host α1-2-fucosyltransferase, resulting in incorporation of fucose into surface Fucα1-2Gal glycoconjugates on the epithelial lining. B. thetaiotaomicron also expresses multiple fucosidases to cleave these terminal fucose residues and a fucose permease for uptake of the released sugar. Thus, the gut commensal has evolved a system for engineering the production of its own nutrient source from its host; but because the system is regulated for use only in times of need, the host in turn only has to synthesize enough fucosylated glycans to support the maintenance of this important member of its normal microbiota. Moreover, the well-adapted B. thetaiotaomicron has evolved additional elaborate systems for regulating its expression of particular polysaccharide-binding proteins and glycosidases to forage and consume sugars from the host's dietary intake when abundant or to switch over to glycans in the host mucus lining when sufficient polysaccharides are missing from the diet.
H. pylori colonizes nearly half the world's population, but it triggers chronic gastritis and stomach ulcers (conditions that are known to increase the risk of stomach cancer) in only a small subset of these individuals. Patterns of glycan expression in both host and microbe appear to help determine whether H. pylori persists as a benign commensal or triggers disease pathology. H. pylori expresses an adhesin (BabA) that can interact directly with gastric epithelium-expressing glycans that terminate with the Lewis b blood group antigen. Lewis b expression in human intestines is limited to mucus-producing pit cells in the gastric epithelium. Transgenic mice engineered to express Lewis b show enhanced binding of H. pylori to their gastric epithelium, which triggers an enhanced cellular immune response and more severe gastritis. This microenvironment of immune activation appears to set the stage for a glycan-based process of molecular mimicry that can promote further host-cell damage. H. pylori also expresses Lewis x–containing structures in the O-antigen of its own LPS, which resemble Lewis x–modified glycans on the surface of parietal cells in the gastric lining. This Lewis antigen mimicry can be varied through the expression of two variable α1,3-fucosyltransferases, FutA and FutB. Also, similar to B. thetaiotaomicron, H. pylori has developed mechanisms to obtain fucose from its host. The presence of H. pylori stimulates the host to secrete α-L-fucosidase 2 (FUCA2). This, in turn, increases the expression of the Lewis x–containing LPS O-antigen in H. pylori. Variation in both organism and host in the expression of Lewis x glycan structures and/or the adhesins may help explain the wide range of potential clinical outcomes following colonization by H. pylori.
This chapter provides only a glimpse of the diverse roles glycans play in the interactions between individual viruses and bacteria with their hosts. As scientists explore varying environments and their associated microbial communities (which also include parasites, fungi, and bacteriophages), it is becoming increasingly apparent that glycans influence every aspect of these interactions and there remains so much more to discover.
ACKNOWLEDGMENTS
The authors acknowledge contributions to the previous version of this chapter from Victor Nizet and Jeffrey D. Esko and helpful comments and suggestions from Felix Broecker, Natalie Silmon de Monerri, and Omai Garner.
FURTHER READING
- Hooper LV, Gordon JI. 2001. Glycans as legislators of host-microbial interactions: Spanning the spectrum from symbiosis to pathogenicity. Glycobiology 11: 1R–10R. [PubMed: 11287395]
- Spear PG. 2004. Herpes simplex virus: Receptors and ligands for cell entry. Cell Microbiol 6: 401–410. [PubMed: 15056211]
- Olofsson S, Bergstrom T. 2005. Glycoconjugate glycans as viral receptors. Ann Med 37: 154–172. [PubMed: 16019714]
- Comstock LE, Kasper DL. 2006. Bacterial glycans: Key mediators of diverse host immune responses. Cell 126: 847–850. [PubMed: 16959564]
- Ji X, Chen Y, Faro J, Gewurz H, Bremer J, Spear GT. 2006. Interaction of human immunodeficiency virus (HIV) glycans with lectins of the human immune system. Curr Protein Pept Sci 7: 317–324. [PubMed: 16918446]
- Munford RS, Varley AW. 2006. Shield as signal: Lipopolysaccharides and the evolution of immunity to gram-negative bacteria. PLoS Pathog 2: e67. [PMC free article: PMC1483240] [PubMed: 16846256]
- Wu L, KewalRamani VN. 2006. Dendritic-cell interactions with HIV: Infection and viral dissemination. Nat Rev Immunol 6: 859–868. [PMC free article: PMC1796806] [PubMed: 17063186]
- Akhtar J, Shukla D. 2009. Viral entry mechanisms: Cellular and viral mediators of herpes simplex virus entry. FEBS J 276: 7228–7236. [PMC free article: PMC2801626] [PubMed: 19878306]
- Schwarz F, Fan YY, Schubert M, Aebi M. 2011. Cytoplasmic N-glycosyltransferase of Actinobacillus pleuropneumoniae is an inverting enzyme and recognizes the NX(S/T) consensus sequence. J Biol Chem 286: 35267–35274. [PMC free article: PMC3186387] [PubMed: 21852240]
- Koropatkin NM, Cameron EA, Martens EC. 2012. How glycan metabolism shapes the human gut microbiota. Nat Rev Microbiol 10: 323–335. [PMC free article: PMC4005082] [PubMed: 22491358]
- Needham BD, Trent MS. 2013. Fortifying the barrier: The impact of lipid A remodelling on bacterial pathogenesis. Nat Rev Microbiol 11: 467–481. [PMC free article: PMC6913092] [PubMed: 23748343]
- Stencel-Baerenwald JE, Reiss K, Reiter DM, Stehle T, Dermody TS. 2014. The sweet spot: Defining virus–sialic acid interactions. Nat Rev Microbiol 12: 739–749. [PMC free article: PMC4791167] [PubMed: 25263223]
- Lu Q, Li S, Shao F. 2015. Sweet talk: Protein glycosylation in bacterial interaction with the host. Trends Microbiol 23: 630–641. [PubMed: 26433695]
- Peng W, de Vries RP, Grant OC, Thompson AJ, McBride R, Tsogtbaatar B, Lee PS, Razi N, Wilson IA, Woods RJ, Paulson JC. 2017. Recent H3N2 viruses have evolved specificity for extended, branched human-type receptors, conferring potential for increased avidity. Cell Host Microbe 21: 23–34. [PMC free article: PMC5233592] [PubMed: 28017661]
- Review Bacterial and Viral Infections.[Essentials of Glycobiology. 2022]Review Bacterial and Viral Infections.Lewis AL, Szymanski CM, Schnaar RL, Aebi M. Essentials of Glycobiology. 2022
- Review Bacterial and Viral Infections.[Essentials of Glycobiology. 2009]Review Bacterial and Viral Infections.Nizet V, Esko JD. Essentials of Glycobiology. 2009
- Review Polymicrobial Diseases[ 2002]Review Polymicrobial DiseasesBrogden KA, Guthmiller JM. 2002
- Review Glycans in Acquired Human Diseases.[Essentials of Glycobiology. 2009]Review Glycans in Acquired Human Diseases.Varki A, Freeze HH. Essentials of Glycobiology. 2009
- Review Infection's Sweet Tooth: How Glycans Mediate Infection and Disease Susceptibility.[Trends Microbiol. 2018]Review Infection's Sweet Tooth: How Glycans Mediate Infection and Disease Susceptibility.Taylor SL, McGuckin MA, Wesselingh S, Rogers GB. Trends Microbiol. 2018 Feb; 26(2):92-101. Epub 2017 Oct 24.
- Bacterial and Viral Infections - Essentials of GlycobiologyBacterial and Viral Infections - Essentials of Glycobiology
- PCK2 [Microcebus murinus]PCK2 [Microcebus murinus]Gene ID:105866581Gene
Your browsing activity is empty.
Activity recording is turned off.
See more...