The content of this book is licensed under a Creative Commons Attribution-NonCommercial-NoDerivs 4.0 Unported license. To view the terms and conditions of this license, visit https://creativecommons.org/licenses/by-nc-nd/4.0/
NCBI Bookshelf. A service of the National Library of Medicine, National Institutes of Health.
Varki A, Cummings RD, Esko JD, et al., editors. Essentials of Glycobiology [Internet]. 4th edition. Cold Spring Harbor (NY): Cold Spring Harbor Laboratory Press; 2022. doi: 10.1101/glycobiology.4e.37
Symbiotic, commensal, and pathogenic microorganisms employ cell-surface glycans as targets for interaction with the host. Surface proteins (adhesins or agglutinins) mediate binding to such glycan “receptors,” and many microbes depend on these interactions for success in the host. In addition, antagonistic interactions are mediated by secreted toxins that use surface glycan targets for internalization by various mechanisms. This chapter highlights examples of microbial lectins and their roles in pathogenicity. In addition, the modes of action of glycan-dependent toxins is discussed.
BACKGROUND
During the last 70 years, an enormous array of glycan-binding proteins (lectins) of viruses, bacteria, fungi, and protozoa have been discovered and characterized. Many of these microbial lectins were originally detected based on their ability to aggregate or induce the hemagglutination of red blood cells (erythrocytes). The influenza virus hemagglutinin was the first identified pathogen glycan-binding protein, shown by Alfred Gottschalk in the early 1950s to bind erythrocytes and other cells via the sialic acid component of host cell-surface glycoconjugates. Don Wiley and associates crystallized the influenza hemagglutinin and determined its structure in 1981. Later, they solved the structure of hemagglutinin cocrystals bound to sialyllactose, providing molecular insight into the affinity and specificity of the receptor-ligand binding sites.
Nathan Sharon and colleagues first described bacterial surface lectins in the 1970s. Their primary function is to facilitate the attachment or adherence of bacteria to host cells, a prerequisite for bacterial colonization and infection (Chapter 42). Thus, bacterial lectins are often called adhesins, and these bind corresponding glycan receptors on the surface of the host cells via carbohydrate-recognition domains (CRDs) (“receptor” in this case is equivalent to “ligand” for animal cell lectins). Microbial adhesins can bind terminal sugar residues or internal sequences found in linear or branched oligosaccharide chains. These interactions are often an important determinant of host and tissue tropism. Detailed studies of the specificity of such microbial lectins have led to the identification and synthesis of powerful inhibitors of adhesion that may form the basis for novel therapeutic agents to combat infectious disease (Chapter 42). In fact, mammals often produce their own glycan decoys such as secreted mucins and milk oligosaccharides that help prevent binding of pathogen adhesins to their host cell targets.
Glycan-dependent interactions are important for both antagonistic and mutualistic interactions. For example, bacteria produce multimeric soluble toxins, whose glycan-binding subunits target and deliver the toxic cargo to the cell. In contrast, the normal flora of the lower gastrointestinal tract is determined by appropriate and desirable colonization by beneficial bacteria that engage in binding and digestion of host and dietary glycans. Likewise, the initial formation of nitrogen-fixing nodules in leguminous root tips by species of Rhizobium involves lectins on the root tip binding to glycan-containing Nod factors generated by the bacterium (Chapter 24).
VIRAL GLYCAN-BINDING PROTEINS
The first discovered and most well-studied example of a viral glycan-binding protein is the influenza virus hemagglutinin. Similar to most other lectin–glycan interactions, the affinity of this sialic acid–binding lectin is low. Avidity is increased by hemagglutinin trimerization and a high density of host cell surface glycan receptors. Binding is required for viral endocytosis and the subsequent pH-dependent fusion of the viral envelope with the endosomal membrane, ultimately triggering release of the viral RNA into the cytosol. The specificity of the host glycan–hemagglutinin interaction varies considerably for different subtypes of influenza. For example, human strains of influenza-A and -B viruses bind primarily to cells containing N-acetylneuraminic acid (Neu5Acα)2–6Gal-containing receptors. However, avian influenza viruses preferentially bind to receptors expressing Neu5Acα2–3Gal-, and porcine strains bind to both Neu5Acα2–6Gal- and -3Gal-containing receptors (Table 37.1). This linkage preference is a result of structural differences in the hemagglutinin (Figure 37.1). Viral adherence also depends on receptor abundance, such that tracheal epithelial cells in humans express glycans with a preponderance of Neu5Acα2–6Gal linkages, whereas other deeper airway surfaces contain many more Neu5Acα2–3Gal-terminated glycans. Thus, the specificity of the hemagglutinin determines the tropism of the virus with respect to species and target cells. As a result, avian-derived strains have a greater likelihood of producing lower respiratory tract infection (pneumonia) than common human strains because of the high abundance of Neu5Acα2-3Gal linkages deep in the lung. Conversely, avian-derived strains have a reduced likelihood of human-to-human transmission compared to human strains because of the paucity of Neu5Acα2-3Gal in the upper respiratory tract. The hemagglutinin is the major antigen against which neutralizing antibodies are produced and influenza strains continuously acquire genetic changes that affect both glycan binding and antigenicity. Such changes are responsible in part for new outbreaks and are considered during formulation of the annual influenza vaccine.
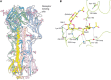
FIGURE 37.1.
Structure of the influenza virus hemagglutinin (HA) ectodomain. (A) A schematic diagram of the trimeric ectodomain of the H3 avian HA from A/duck/Ukr/63 showing residues HA1 9–326 and HA2 1–172. Modeled carbohydrate side chains (gray, (more...)
TABLE 37.1.
Examples of viral lectins and hemagglutinins
In addition to the hemagglutinin “H,” influenza-A and -B virions express a sialidase (traditionally called neuraminidase “N”, Chapter 15) that cleaves sialic acids from glycoconjugates. Its functions may include (1) prevention of viral aggregation by removal of sialic acid residues from virion envelope glycoproteins; (2) dissociation of newly synthesized virions inside the cell or as they bud from the cell surface; and (3) desialylation of soluble mucins at sites of infection to improve access to cell surface sialic acids. Inhibitors have been designed based on the crystal structure of the sialidase from influenza-A virus. Some of these (e.g., oseltamivir) inhibit the enzyme activity at nanomolar concentrations and are used clinically as antiviral agents (Chapter 57). Influenza-C virions (and many coronaviruses) contain a single “hemagglutinin-esterase” that possesses both hemagglutinin and receptor-destroying activities, which in this case is an esterase that cleaves the 9-O-acetyl group from the target O-acetylated sialic acid receptors (Table 37.1). Some coronaviruses have evolved to adapt the spike “S” proteins to target O-acetylated sialic acid receptors. The severe acute respiratory syndrome coronavirus 2 (SARS-CoV-2), causative agent of the COVID-19 pandemic, has completely lost its hemagglutinin-esterase protein, but encodes an S protein that has evolved further, to bind more robustly to heparan sulfate (HS) proteoglycans, which can also act as a cofactor facilitating binding to a high-affinity protein receptor ACE2.
Rotaviruses, the major killer of children worldwide, can also bind to sialic acid residues. These viruses only bind to the intestinal epithelium of newborn infants during a period that appears to correlate with the expression of specific types and arrangements of sialic acids on glycoproteins. Claims regarding “sialic acid–independent” rotaviruses may be explained by internal sialic acids resistant to bacterial sialidases. Many other viruses (e.g., adenovirus, reovirus, Sendai virus, and polyomavirus) also use sialic acids for infection, and crystal structures are now available for several of their sialic acid–binding domains.
A number of viruses, including herpes simplex virus (HSV), foot-and-mouth disease virus, human immunodeficiency virus (HIV), and dengue flavivirus, use heparan sulfate proteoglycans as adhesion receptors (Table 37.1). In many cases, the proteoglycans may be part of a coreceptor system in which viruses make initial contact with a cell-surface proteoglycan and later with another receptor. For example, HSV infection is thought to initially involve the binding of viral glycoproteins gB and/or gC to cell-surface heparan sulfate proteoglycans. Glycoprotein gB promotes virus-cell fusion, syncytium formation (cell–cell fusion), and adherence, whereas gC binds to the C3b component of complement and blocks complement-mediated inhibition of the virus. These events are followed by HSV glycoprotein gD binding to one of several cell-surface receptors, including protein receptors and heparan sulfate, ultimately leading to fusion of the viral envelope with the host-cell plasma membrane. Interestingly, the interaction of gD with heparan sulfate shows specificity for a particular substructure in heparan sulfate containing a 3-O-sulfated glucosamine residue, the formation of which is catalyzed by specific isozymes of the glucosaminyl 3-O-sulfotransferase gene family. Thus, the heparan sulfate–binding adhesins appear to recognize carbohydrate units within the polysaccharide chains as opposed to binding terminal sugars.
Dengue flavivirus, the causative agent of dengue hemorrhagic fever, also binds to heparan sulfate. Computational modeling to compare the primary dengue viral envelope protein sequence with the crystal structure of a related virion envelope protein suggests that the heparan sulfate–binding site may lie along a positively charged amino acid groove (Figure 37.2). The recently emerging Zika virus belongs to the same family. Furthermore, HIV can bind heparan sulfate and other sulfated polysaccharides by way of the V3 loop of its gp120 glycoprotein.
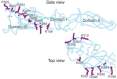
FIGURE 37.2.
Two views of a putative heparin sulfate–binding site on the dengue virus envelope protein. The envelope protein monomer is shown in ribbon form, displayed along its longitudinal axis and as an external side view. Note the alignment of positively (more...)
BACTERIAL ADHESION TO GLYCANS
Bacterial lectins occur commonly in the form of elongated multisubunit protein appendages, known as fimbriae (hairs) or pili (threads), which interact with glycoprotein and glycolipid receptors on host cells. Similar to viral glycan-binding proteins, adhesin-receptor binding is generally of low affinity. Because the adhesins and the receptors often cluster in the plane of the membrane, the resulting combinatorial avidity can be great. An analogy for adhesin–receptor binding is the interaction of the two faces of Velcro strips. The most well-characterized bacterial lectins include the mannose-specific type-1 fimbriae, the galabiose-specific P fimbriae, and the N-acetylglucosamine-binding F-17 fimbriae, which are produced by different strains of Escherichia coli. Fimbriated bacteria express 100 to 400 of these appendages, which typically have a diameter of 5–7 nm and can extend hundreds of nanometers in length (Figure 37.3) Thus, pili extend well beyond the bacterial glycocalyx comprised of lipopolysaccharide and capsular polysaccharides (see Chapter 21).
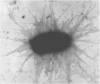
FIGURE 37.3.
Escherichia coli express hundreds of pili, as indicated by the fine filaments extending from the bacterium. (Reprinted, with permission of Elsevier, from Sharon N. 2006. Biochim Biophys Acta 1760: 527–537; courtesy of David L. Hasty, University (more...)
Examination of a high-resolution, three-dimensional structure of FimH bound to mannose revealed that, although mannose exists as a mixture of α and β anomers in solution, only the former was found in the complex. Mannose binds FimH at a deep and negatively charged site of FimH (Figure 37.4). FimH has “catch-bond” properties, such that the binding strength of individual FimH adhesins is augmented by increasing the shear forces on surface-bound E. coli cells. This is relevant for urinary tract infections, in which type-1 fimbriae mediate binding of the bacteria to the glycoprotein uroplakin Ia on the surface of bladder epithelial cells. Uroplakin Ia presents high levels of terminally exposed mannose residues that are capable of specifically interacting with FimH. Alternatively, type-1 fimbriae can bind to high mannose glycans on the soluble urinary Tamm–Horsfall glycoprotein/uromodulin, that forms long fibers. The resulting bacterial aggregates are flushed out of the urinary tract. Mice lacking the gene encoding the Tamm–Horsfall glycoprotein are considerably more susceptible to bladder colonization by type-1-fimbriated E. coli than normal mice.
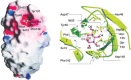
FIGURE 37.4.
The α anomer of mannose in the binding site of FimH. The mannose residue is buried in a unique site at the tip of the carbohydrate-recognition domain (left) in a deep and negatively charged pocket (right). FimH prefers to bind D-mannose in the (more...)
Most bacteria (and possibly other microorganisms) have multiple adhesins with diverse carbohydrate specificities. Many of these have been described (examples in Table 37.2), wherein the specificity of lectin binding can help define the range of susceptible tissues in the host (i.e., the microbe's ecological niche). The columnar epithelium that lines the large intestine expresses receptors with Galα1–4Gal-Cer residues, whereas cells lining the small intestine do not. Thus, Bacterioides, Clostridium, E. coli, and Lactobacillus only colonize the large intestine under normal conditions. P-fimbriated E. coli and some toxins bind specifically to galabiose (Galα1–4Gal) and galabiose-containing oligosaccharides, most commonly as constituents of glycolipids. Binding can occur to either internal (i.e., when the disaccharide is capped by other sugars) or terminal nonreducing galabiose units. P-fimbriated E. coli adhere mainly to the upper part of the kidney, where galabiose is abundant. The fine specificity of bacterial surface lectins and their relationship to the animal tropism of the bacteria can be further illustrated by E. coli K99. The K99 strain binds to glycolipids that contain N-glycolylneuraminic acid (Neu5Gc), in the form of Neu5Gcα2–3Galβ1–4Glc, but not to those that contain N-acetylneuraminic acid (Neu5Ac). These two sugars differ by a single hydroxyl group that is present only on Neu5Gc. Interestingly, Neu5Gc-containing receptors are expressed on the intestinal cells of newborn piglets, but disappear as the animals grow and develop. As Neu5Gc is not normally biosynthesized by humans, this may explain why E. coli K99 can cause often lethal diarrhea in piglets but not in adult pigs or humans.
TABLE 37.2.
Examples of interactions of bacterial adhesins with glycans
SECRETED TOXINS THAT BIND GLYCANS
A number of secreted bacterial toxins also bind glycans (Table 37.3). The toxin from Vibrio cholera (cholera toxin), which consists of A and B subunits in the ratio AB5, has been extensively studied. The crystal structure of cholera toxin shows that the carbohydrate-recognition domains are located at the base of the B subunits, which bind to the Galβ1–3GalNAc moiety of GM1 ganglioside (Chapter 11) receptors (Figure 37.5). On binding of the B subunits to membrane glycolipids, the AB5 complex is endocytosed to the Golgi apparatus and then undergoes retrograde transport to the endoplasmic reticulum (ER). The A1 and A2 chains are proteolytically cleaved on toxin secretion, but remain stably associated until arrival in the ER. There, the enzymatic A1 chain unfolds, dissociates from the A2-B5 complex, and retrotranslocates to the cytosol where it rapidly refolds, thereby avoiding degradation by the proteasome. Catalytically active A1 then ADP-ribosylates a regulatory homotrimeric G-protein to activate adenylyl cyclase, severely altering ion homeostasis of the infected cell.
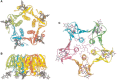
FIGURE 37.5.
Crystal structure of the cholera toxin B-subunit pentamer bound to GM1 pentasaccharide, shown from the bottom (A) and the side (B). (Redrawn, with permission, from Merritt EA, et al. 1994. Protein Sci 3: 166–175.) (C) Crystal structure of the (more...)
TABLE 37.3.
Examples of glycan receptors for bacterial toxins
Shiga toxin, produced by Shigella dysenteriae, will bind to Galα1-4Gal determinants on both glycolipids and glycoproteins. However, only binding to the glycosphingolipid receptor Gb3 results in cell death. Similar to cholera toxin, the AB5 complex is endocytosed and transported to the ER of the target cells, and then the A1 subunit is retrotranslocated to the cytosol. There, the catalytic A1 chain of Shiga toxin inactivates ribosomes, and thus the essential process of cytoplasmic protein synthesis, by an N-glycosidic cleavage event that depurinates 28S rRNA.
The most common class of bacterial toxins that are characterized by their pore-forming capability and a number of these toxins bind to glycans. For example, toxins produced by the soil-dwelling bacterium Bacillus thuringiensis (Bt) are used for crop protection by spraying plants or by genetically engineering crops to express the toxins. As such, these toxins function by binding glycolipids that line the insect gut and generating pores in the membrane. More specifically, the Bt toxin glycolipid receptors include in their structure the characteristic ceramide-linked, mannose-containing core tetrasaccharide GalNAcβ1-4GlcNAcβ1-3Manβ1-4GlcβCer. This structure is conserved between nematodes and insects (see Chapter 25) but is absent in vertebrates including humans. This explains why Bt can kill larval stages of insects but is harmless to humans.
Secondary glycan binding sites have been identified on some toxins. For example, cholera toxin can bind fucosylated glycans at a site distinct from the GM1 binding pocket, and Clostridium difficile toxin A (TcdA) binds sulfated glycosaminoglycans in addition to GalNAcβ1-3Galβ1-4GlcNAcβ1-3 Galβ1-4GlcβCer. Whereas these secondary interactions are lower affinity that those with the canonical receptors, the glycans recognized are abundant on the surface of host cells. Thus, glycan binding to secondary sites may serve as initial attachment events that capture and concentrate toxins on cell surfaces.
PARASITE LECTINS
In addition to viruses and bacteria, a number of parasites use glycans as receptors for adhesion (Table 37.4). Entamoeba histolytica expresses a 260-kDa heterodimeric lectin that binds to terminal Gal/GalNAc residues on glycoproteins and glycolipids via a cysteine-rich glycan-binding domain. This adhesin is essential for virulence, such that adhesin-glycan binding is required for parasitic attachment, invasion, and cytolysis of the intestinal epithelium. Furthermore, it may function in binding E. histolytica to bacteria as a food source. This adhesin elicits protective immunity, and is a potential target to manage E. histolytica infection.
TABLE 37.4.
Examples of glycan receptors for parasites
The initial interaction of Plasmodium falciparum (malaria) merozoites with red blood cells (erythrocytes) depends on sialic acid residues present on the host cell, in particular on the major erythrocyte membrane protein glycophorin. Parasite–host attachment is mediated by a family of sialic acid–binding adhesins on merozoites, the most prominent of which is called erythrocyte-binding antigen-175 (EBA-175). This adhesin preferentially binds Neu5Ac sialic acids, rather than 9-O-acetyl-Neu5Ac or Neu5Gc, and is sensitive to the linkage of the sialic acid to the underlying galactose. This is highlighted by the fact that Neu5Acα2–3Gal-containing oligosaccharides effectively inhibit the binding of EBA-175 to erythrocytes, whereas soluble Neu5Ac and Neu5Acα2–6Gal-containing oligosaccharides do not. Adhesin–glycan binding triggers invasion of the merozoites into red blood cells, where they develop into mature schizonts that rupture and release newly formed merozoites into the bloodstream. Many commonly used clinical antimalarial medications, such as chloroquine, target the parasite during this erythrocytic asexual reproduction stage.
THERAPEUTIC IMPLICATIONS
Various glycan-binding proteins mediate adhesion of microorganisms to host cells or tissues. These interactions are often a prerequisite for infection or symbiosis, with binding-deficient mutants being unable to initiate or maintain these relationships. Interestingly, glycans recognized by microbial surface lectins have been shown to block the adhesion of bacteria to animal cells in vitro and in vivo, and thus may protect animals against infection by such microorganisms. For example, coadministration of methyl α-mannoside with type-1-fimbriated E. coli into the bladder of mice can reduce microbial burden in a mouse model of urinary tract infection, whereas methyl α-glucoside, which does not bind to FimH, has no effect. Furthermore, lacto-N-neotetraose (LNnT) and its α2-3- and α2-6-sialylated derivatives block the adherence of Streptococcus pneumoniae to respiratory epithelial cells in vitro. In addition, these glycans prevent colonization of the nasopharynx and attenuate the course of pneumonia in rodent models of pneumococcal infection. Naturally occurring host glycans can also serve protective roles. For example, secreted mucins and human milk oligosaccharides (HMOs) act as natural receptor decoys, protecting the host from pathogens and shaping the microbiota composition.
Exogenous heparin and structurally related polysaccharides are known to inhibit viral replication, suggesting a potential approach for the development of polysaccharide-based antiviral pharmaceutical agents. For example, heparin octasaccharide decoy liposomes were recently shown to inhibit the replication of numerous viruses including HSV and respiratory syncytial virus. As more crystal structures are elucidated, the ability to design small molecule inhibitors that fit into the carbohydrate-recognition domains of adhesins should improve. Already, the structures of influenza hemagglutinin and sialidase have suggested numerous ways to modify sialic acid to fit better into the active sites. Some of these compounds are presently in clinical use to limit the spread of influenza.
ACKNOWLEDGMENTS
The authors acknowledge contributions to the previous version of this chapter from Jeffrey D. Esko, Victor Nizet, and the late Nathan Sharon and helpful comments and suggestions from Frederique Lisacek and Ajit Varki.
FURTHER READING
- Rostand KS, Esko JD. 1997. Microbial adherence to and invasion through proteoglycans. Infect Immun 65: 1–8. doi:10.1128/iai.65.1.1-8.1997 [PMC free article: PMC174549] [PubMed: 8975885] [CrossRef]
- Kitov PI, Sadowska JM, Mulvey G, Armstrong GD, Ling H, Pannu NS, Read RJ, Bundle DR. 2000. Shiga-like toxins are neutralized by tailored multivalent carbohydrate ligands. Nature 403: 669–672. doi:10.1038/35001095 [PubMed: 10688205] [CrossRef]
- Griffitts JS, Aroian RV. 2005. Many roads to resistance: how invertebrates adapt to Bt toxins. BioEssays 27: 614–624. doi:10.1002/bies.20239 [PubMed: 15892110] [CrossRef]
- Olofsson S, Bergstrom T. 2005. Glycoconjugate glycans as viral receptors. Ann Med 37: 154–172. doi:10.1080/07853890510007340 [PubMed: 16019714] [CrossRef]
- Mazmanian SK, Kasper DL. 2006. The love–hate relationship between bacterial polysaccharides and the host immune system. Nat Rev Immunol 6: 849–858. doi:10.1038/nri1956 [PubMed: 17024229] [CrossRef]
- Sinnis P, Coppi A. 2007. A long and winding road: the Plasmodium sporozoite's journey in the mammalian host. Parasitol Int 56: 171–178. doi:10.1016/j.parint.2007.04.002 [PMC free article: PMC1995443] [PubMed: 17513164] [CrossRef]
- Patsos G, Corfield A. 2009. Management of the human mucosal defensive barrier: evidence for glycan legislation. Biol Chem 390: 581–590. doi:10.1515/bc.2009.052 [PubMed: 19335202] [CrossRef]
- Krachler AM, Orth K. 2013. Targeting the bacteria–host interface: strategies in anti-adhesion therapy. Virulence 4: 284–294. doi:10.4161/viru.24606 [PMC free article: PMC3710331] [PubMed: 23799663] [CrossRef]
- Edinger TO, Pohl MO, Stertz S. 2014. Entry of influenza A virus: host factors and antiviral targets. J Gen Virol 95: 263–277. doi:10.1099/vir.0.059477-0 [PubMed: 24225499] [CrossRef]
- Stencel-Baerenwald JE, Reiss K, Reiter DM, Stehle T, Dermody TS. 2014. The sweet spot: defining virus–sialic acid interactions. Nat Rev Microbiol 12: 739–749. doi:10.1038/nrmicro3346 [PMC free article: PMC4791167] [PubMed: 25263223] [CrossRef]
- Bode L. 2015. The functional biology of human milk oligosaccharides. Early Hum Dev 91: 619–622. doi:10.1016/j.earlhumdev.2015.09.001 [PubMed: 26375354] [CrossRef]
- Zajonc DM, Girardi E. 2015. Recognition of microbial glycolipids by natural killer T cells. Front Immunol 6: 400. doi:10.3389/fimmu.2015.00400 [PMC free article: PMC4523824] [PubMed: 26300885] [CrossRef]
- Juge N, Tailford L, Owen CD. 2017. Sialidases from gut bacteria: a mini-review. Biochem Soc Trans 44: 166–175. doi:10.1042/bst20150226 [PMC free article: PMC4747158] [PubMed: 26862202] [CrossRef]
- Moonens K, Remaut H. 2017. Evolution and structural dynamics of bacterial glycan binding adhesins. Curr Opin Struct Biol 44: 48–58. doi:10.1016/j.sbi.2016.12.003 [PubMed: 28043017] [CrossRef]
- Raman R, Tharakaraman K, Sasisekharan V, Sasisekharan R. 2017. Glycan–protein interactions in viral pathogenesis. Curr Opin Struct Biol 40: 153–162. doi:10.1016/j.sbi.2016.10.003 [PMC free article: PMC5526076] [PubMed: 27792989] [CrossRef]
- Ramani S, Hu L, Venkataram Prasad BV, Estes MK. 2017. Diversity in rotavirus–host glycan interactions: a “sweet” spectrum. Cell Mol Gastroenterol Hepatol 2: 263–273. doi:10.1016/j.jcmgh.2016.03.002 [PMC free article: PMC5042371] [PubMed: 28090561] [CrossRef]
- Thomas GH. 2017. Sialic acid acquisition in bacteria—one substrate, many transporters. Biochem Soc Trans 44: 760–765. doi:10.1042/bst20160056 [PubMed: 27284039] [CrossRef]
- Tytgat HL, de Vos WM. 2017. Sugar coating the envelope: glycoconjugates for microbe–host crosstalk. Trends Microbiol 24: 853–861. doi:10.1016/j.tim.2016.06.004 [PubMed: 27374775] [CrossRef]
- Valguarnera E, Kinsella RL, Feldman MF. 2017. Sugar and spice make bacteria not nice: protein glycosylation and its influence in pathogenesis. J Mol Biol 428: 3206–3220. doi:10.1016/j.jmb.2016.04.013 [PubMed: 27107636] [CrossRef]
- Wasik BR, Barnard KN, Parrish CR. 2017. Effects of sialic acid modifications on virus binding and infection. Trends Microbiol 24: 991–1001. doi:10.1016/j.tim.2016.07.005 [PMC free article: PMC5123965] [PubMed: 27491885] [CrossRef]
- Poole J, Day CJ, von Itzstein M, Paton JC, Jennings MP. 2018. Glycointeractions in bacterial pathogenesis. Nat Rev Microbiol 16: 440–452. doi:10.1038/s41579-018-0007-2 [PubMed: 29674747] [CrossRef]
- Thompson AJ, de Vries RP, Paulson JC. 2019. Virus recognition of glycan receptors. Curr Opin Virol 34: 117–129. doi:10.1016/j.coviro.2019.01.004 [PMC free article: PMC6476673] [PubMed: 30849709] [CrossRef]
- Review Microbial Lectins: Hemagglutinins, Adhesins, and Toxins.[Essentials of Glycobiology. 2015]Review Microbial Lectins: Hemagglutinins, Adhesins, and Toxins.Nizet V, Varki A, Aebi M. Essentials of Glycobiology. 2015
- Review Microbial Lectins: Hemagglutinins, Adhesins, and Toxins.[Essentials of Glycobiology. 2009]Review Microbial Lectins: Hemagglutinins, Adhesins, and Toxins.Esko JD, Sharon N. Essentials of Glycobiology. 2009
- Lectin-Glycan Interaction Network-Based Identification of Host Receptors of Microbial Pathogenic Adhesins.[mBio. 2016]Lectin-Glycan Interaction Network-Based Identification of Host Receptors of Microbial Pathogenic Adhesins.Ielasi FS, Alioscha-Perez M, Donohue D, Claes S, Sahli H, Schols D, Willaert RG. mBio. 2016 Jul 12; 7(4). Epub 2016 Jul 12.
- Review An overview of lectin-glycan interactions: a key event in initiating fungal infection and pathogenesis.[Arch Microbiol. 2018]Review An overview of lectin-glycan interactions: a key event in initiating fungal infection and pathogenesis.Ballal S, Inamdar SR. Arch Microbiol. 2018 Apr; 200(3):371-382. Epub 2018 Feb 13.
- Review Bitter-sweet symphony: glycan-lectin interactions in virus biology.[FEMS Microbiol Rev. 2014]Review Bitter-sweet symphony: glycan-lectin interactions in virus biology.Van Breedam W, Pöhlmann S, Favoreel HW, de Groot RJ, Nauwynck HJ. FEMS Microbiol Rev. 2014 Jul; 38(4):598-632. Epub 2013 Dec 6.
- Microbial Lectins: Hemagglutinins, Adhesins, and Toxins - Essentials of Glycobio...Microbial Lectins: Hemagglutinins, Adhesins, and Toxins - Essentials of Glycobiology
Your browsing activity is empty.
Activity recording is turned off.
See more...