NCBI Bookshelf. A service of the National Library of Medicine, National Institutes of Health.
Mobley HLT, Mendz GL, Hazell SL, editors. Helicobacter pylori: Physiology and Genetics. Washington (DC): ASM Press; 2001.
For many pathogenic bacteria, flagellum-dependent motility and chemotaxis are crucial factors in the process of colonization of the host organism and establishment of a successful infection (reviewed in references 36 and 39). The flagella of Helicobacter pylori have been extensively studied, and convincing evidence is available demonstrating the key role of these organelles in the colonization of the human gastric mucosa by this fastidious pathogen. Experiments with gnotobiotic piglets have established a correlation between the motility state of some H. pylori isolates and their ability to colonize the gastric epithelium (11, 12). The characterization of isogenic mutants deficient in specific flagellar proteins has confirmed the importance of an intact flagellar apparatus and the resulting motility for H. pylori pathogenicity (8, 13, 17, 21, 28, 29). Structural components of the flagellum as well as secretory and regulatory proteins involved in the synthesis of the flagellar apparatus and the control of chemotaxis have been analyzed in recent years, and our knowledge regarding the mechanisms of H. pylori motility is rapidly increasing. This chapter summarizes the available experimental and genomic data on flagellar function and provides an overview of our current knowledge of this field. The emphasis will be on the regulation of flagellar gene transcription and chemotaxis, while a more detailed description of the morphology of the H. pylori flagellum will be given in chapter 7.
The Flagella
H. pylori cells normally possess a unipolar bundle of two to six sheathed flagella that enable the bacteria to move in their ecological niche represented by the mucous layer of the gastric epithelium (45). Each flagellum is about 3 μm long and exhibits a typical bulb-like structure at its distal end that represents a dilation of the flagellar sheath (20). The sheath itself is an extension of the outer membrane and is thought to protect the acid-labile flagellar structure from the attack of the stomach acid (20).
The H. pylori flagella are composed of three structural elements like those of enteric bacteria: a basal body, which is embedded in the cell wall and contains the proteins required for rotation and chemotaxis; an external helically shaped filament that works as a propeller when rotated at its base; and a hook that serves as a joint between the basal body and the flagellar filament (Fig. 1). Table 1 shows that in H. pylori more than 50 putative proteins are predicted to be involved in expression, secretion, and assembly of this complex flagellar apparatus (3, 47). At least 20 of these proteins constitute the structural components of the flagellar basal body, hook, and filament (Table 1). To date, only components of the filament and the hook have been characterized in some detail. The filament is a copolymer of the flagellin subunits FlaA (21, 32) and FlaB (46). The FlaA is the predominant subtype and FlaB the minor subtype, localized close to the basis of the flagellum (30). Both flagellins have similar molecular mass (53 kDa) and share considerable amino acid homology (58% identity), but the respective genes are unlinked in the chromosome (46). Studies with isogenic mutants of either flaA or flaB revealed that both genes are necessary for full motility (28) and for establishment of a persistent infection in the gnotobiotic piglet model (13). The flgE gene encodes the structural protein of the hook, and the fliD gene encodes a hook-associated protein (HAP2), which is localized at the tip of the flagellar filament and which promotes the incorporation of the flagellin monomers into the growing flagellar filament (Fig. 1). These genes have been characterized; mutants in flgE are nonmotile and aflagellate (38), and mutants in fliD produce truncated flagella and are severely impaired in motility and their ability to colonize the gastric mucosae of mice (29). A protein of the flagellar sheath was also characterized and shown to be identical to HpaA, an N-acetylneuraminyllactose binding hemagglutinin (15, 27).
Table 1
Flagellar genes identified in the two published genome sequencesa.
Another structural gene identified on the basis of sequence homologies with characterized genes is fliF encoding the subunits of the MS ring (Fig. 1), the first complex to be assembled in the course of flagellar morphogenesis (26). This complex is formed on the cytoplasmic side of the inner membrane, where it serves as a construction base for the flagellar rod made of the FlgB, FlgC, and FlgG proteins (24) and as an anchor for the motor switch proteins FliM, FliN, and FliG (18, 19) and the motor rotation proteins MotA and MotB (10, 44) (Fig. 1). The genes encoding all of these proteins have been found in the H. pylori genome as well as the flgI and flgH genes encoding the subunits of the P and L rings, (26), which form in the periplasmic space and the outer membrane, respectively (Fig. 1). In addition to FliD, the hook-associated proteins FlgK and FlgL (22), the FlaG polar flagellins, and additional paralogs of the sheath protein HpaA are also encoded by the genome. All these proteins are likely to become incorporated into the H. pylori flagella.
Biosynthesis and Assembly
Most of the flagellar apparatus is localized beyond the cytoplasmic membrane and therefore many of the numerous flagellar proteins have to cross the membrane to reach their final destination. The proteins constituting the P and L rings, which anchor the flagellum in the periplasm and the outer membrane, respectively, are secreted via the conventional signal-peptide–dependent Sec pathway, whereas the axial components of the flagellar apparatus, including the structural proteins of the filament, the hook, and the rod, which connects the hook to the basal body, are believed to be secreted by a specialized flagellum-specific pathway. This pathway is constituted of a number of flagellar biosynthetic proteins that assemble into a structure at the MS ring of the flagellum that binds the flagellar proteins and transfers them actively into the growing flagellum (Fig. 1). The members of this system share homology to components of the widely distributed contact-dependent type III secretion systems, which in other organisms have been found to be involved also in the secretion of virulence factors (5, 9).
In H. pylori the export apparatus is assembled from the proteins encoded by the fliH, fliI, fliQ, fliL, fliP, fliR, flhA (flbA), and flhB genes (Table 1). To date, only the fliI, fliQ, flhA, and flhB genes have been analyzed to some extent. Both fliI and flhA (flbA) code for members of the LcrD/FlbF family of motility and virulence-associated proteins, and knockout mutants in both of these genes were shown to be nonmotile and aflagellate (25, 40, 41). Similarly, the FliQ and FlhB genes were shown to be essential for assembly of the flagellum and for motility of the bacteria (17).
Regulation of Flagellar Gene Transcription
In Salmonella and Caulobacter crescentus the transcriptional regulation of flagellar gene expression has been extensively studied (reviewed in references 34 and 50). In these organisms, expression of the genes required for flagellar biosynthesis is tightly regulated by alternative sigma factors and specific transcriptional activators. In H. pylori only a few flagellar genes have been analyzed regarding their transcriptional regulation. Table 2 shows those H. pylori genes and putative operons for which transcriptional start points have been mapped experimentally and for which a specific promoter sequence could be assigned; the table also shows those genes and operons for which a putative promoter has been deduced on the basis of homology with the consensus recognition sequence of one of the three sigma factors encoded by the H. pylori genome. The analysis shows that the three sigma factors σ80, σ54, and σ28, deduced from the genome sequence analysis, participate in the transcription of flagellar genes, indicating that transcription of H. pylori flagellar genes is highly regulated like in other organisms studied (Table 2).
Table 2
Putative flagellar genes and operons and associated promoter sequences.
Rod and hook functions: σ54-dependent genes
A closer inspection of the genes regulated by RNA polymerase containing the alternative sigma factor σ54 (Table 2) reveals that the proteins encoded by these genes are likely to be localized in the same region of the flagellum. The flgB, flgC, and flgG genes are predicted to code for structural proteins of the rod (24), which traverse the periplasmic space, connecting the hook to the rest of the basal body. flgE and its homolog flgE′ code for structural proteins of the hook (3, 38, 47), flgK and flgL encode the hook-associated proteins HAP1 and HAP3 (22), and flaB codes for the minor flagellin, which is localized at the basis of the flagellar filament and therefore close to the flagellar hook (30, 46). This colocalization suggests that the σ54-dependent genes represent a class of flagellar genes that is coordinately regulated in response to certain environmental factors and/or cell cycle signals.
Transcription of σ54-dependent genes depends on upstream enhancer-like sequences, which are bound by a class of transactivating proteins usually referred to as the NtrC (NR-1) family (31). The H. pylori genome contains the gene HP703/jhp643 encoding a homolog of this class of proteins (Table 1), and the role of this protein in transcription of the flagellar rod and hook genes has been analyzed (42). Isogenic mutants in HP703/jhp643, whose product is FlgR for flagellum regulatory protein, were shown to be nonmotile, and transcription of the σ54-dependent flgE and flaB genes as well as of the flgBC, flgK, and flgDE′ operons was completely abolished in the mutant (42). Thus, the FlgR protein represents the master activator of these hook and hook-associated genes and possibly also of the other putative σ54-dependent genes. To date, it is not known which is the signal that triggers FlgR-mediated activation of transcription. However, an in vitro phosphorylation study with a series of purified signal-transducing histidine-kinase sensor proteins and putative response regulators of H. pylori has shown that FlgR is specifically phosphorylated by the histidine-kinase encoded by HP244/jhp229, suggesting that this gene encodes the sensor kinase for FlgR (6). Interestingly, unlike most other sensor proteins that reside in the cytoplasmic membrane, the protein encoded by HP244 is predicted to be located in the cytoplasm, indicating that additional transmembrane proteins might be involved in signal reception and transduction.
Environmental regulation of σ54-dependent flagellar genes has been shown in the closely related organism Campylobacter coli. In this bacterium, which shares similarities with H. pylori not only in its ecological requirements but also at the level of its genome sequence, the σ54-dependent transcription of flaB can be modulated by growth medium pH, temperature, and the concentration of certain salts (2). It is possible that the modulation of the amount of FlaB and other σ54-controlled flagellar proteins in response to environmental stimuli allows C. coli, and possibly also H. pylori, to produce flagella that are particularly suited for a given environment.
Filament: σ28-dependent transcription
In contrast to flaB, the flaA gene coding for the major flagellin has been shown to be transcribed by RNA polymerase containing σ28 (32), indicating that this gene belongs to a different class of flagellar genes (Table 2). With the recent finding that the fliD operon containing the flaG, fliD, and fliS genes is also under the control of a σ28-dependent promoter (29), it has become clear that such a class exists in H. pylori (Table 2). Similar to the σ54-regulated flagellar proteins, it is likely that the σ28-dependent proteins also become localized to a distinct area in the flagellum: FlaA is the major structural component of the filament (21, 32), FliD is localized at the tip of the filament where it promotes incorporation of flagellin monomers into the growing filament (23, 29), FlaG is a polar flagellin (47), and FliS has been shown to be essential for flagellar elongation (51). Therefore, the σ28-dependent genes might be members of a class of flagellar genes that are coordinately regulated as a function of their colocalization in the flagellar structure.
Basal-body, biosynthetic, and chemotaxis proteins: σ80-dependent transcription?
In contrast to the hook and flagellin genes, whose transcription has been investigated to some extent, very little data are available regarding the transcriptional regulation of the genes encoding the nonaxial proteins of the basal body and the proteins necessary for flagellar biosynthesis and chemotaxis. Characterized loci include the fliI-fliQ operon coding for two flagellar export proteins and a stress-responsive operon containing the gene encoding the chemotaxis regulator CheY. Both of these operons have been shown to be regulated by a σ80-dependent transcription (7, 40), and sequence analysis of the H. pylori genome suggests that the expression of the other flagellar biosynthetic, basal-body, and chemotaxis genes also is regulated by the same sigma factor (Table 2). It is possible that these genes represent a third class of flagellar genes that are coordinately regulated by RNA polymerase containing σ80.
Hierarchy?
The subdivision of the H. pylori flagellar genes into three classes according to their regulation by the three different sigma factors suggests the existence of a regulatory hierarchy, in which the different classes are expressed sequentially in an order that reflects the spatial distribution of the respective gene products in the flagellum. In the two model organisms Salmonella enterica serovar Typhimurium and C. crescentus, such transcriptional hierarchies have been found and extensively studied.
In Salmonella (Fig. 2A), environmental signals trigger expression of class I (early) genes, which encode two master regulatory proteins called FlhC and FlhD. These regulatory proteins are necessary for σ70-dependent expression of class II (middle) genes, which encode the components of the basal body and the hook. Assembly of the basal body-hook complex then acts as a signal for E(α2ββ′)σ28-regulated expression of class III (late) genes, including the genes for chemo-reception and the flagellin gene, by allowing the release of the anti-sigma factor FlgM (34).
In Caulobacter sp. (Fig. 2B) an early signal in the cell cycle is assumed to trigger activation of the class I gene product, a transcriptional regulator (CtrA), which in turn activates σ70-dependent transcription of class II genes encoding structural components of the MS-ring/switch complex. Among the class II genes are also the alternative sigma factor σ54 and its cognate transcriptional regulator FlbD that activate expression of class III and class IV genes, encoding structural proteins of the basal body-hook complex and flagellar filament, respectively. In contrast to the situation in S. enterica serovar Typhimurium, two different checkpoints in the assembly of the flagellar structure control the activation of these late flagellar genes. Completion of the MS-ring/switch complex acts as a signal for transcription of class III genes, whereas completion of the basal body-hook complex is required for activation of class IV genes (50).
Available data suggest that the regulation of flagellar gene transcription in H. pylori shows similarities to both systems. As in Caulobacter sp., RNA polymerase containing the alternative sigma factor σ54 and a cognate transcriptional activator (FlgR) are required for transcription of basal body and hook genes. However, expression of the major flagellin FlaA and other filament-associated proteins appears to be regulated by RNA polymerase containing σ28, thus reflecting the situation found in Salmonella sp. In contrast to both systems, checkpoints in flagellar assembly regulating transcription of flagellar genes seem less obvious in H. pylori. In fact, disruption of the flgR gene, which abolishes synthesis of basal-body and hook proteins or the flgE gene itself, which codes for the structural protein of the hook (38, 42), does not abolish the production of the major flagellin FlaA. Thus, it seems unlikely that an anti-sigma factor protein blocks transcription of σ28-dependent genes in the absence of a functional basal body-hook complex in H. pylori. In support of such a hypothesis is the fact that no gene coding for a homolog of the anti-sigma factor FlgM can be found in the H. pylori genome (3, 47). However, the activator protein FlgR was shown to down-modulate transcription of the flaA gene (42), suggesting that the apparent lack of an anti-sigma factor is partially compensated by a repressive action of FlgR on σ28-transcribed genes. Moreover, mutations in the fliD gene, encoding the capping protein, which promotes polymerization of flagellin subunits at the tip of the growing filament, also cause a decrease in flaA transcription, indicating a feedback control of flagellin production by a functional filament elongation machinery (29).
Interestingly, mutations in the genes coding for components of the type III secretion apparatus, which is associated with the MS ring at the cytoplasmic membrane and involved in export and assembly of the flagellar proteins, have dramatic effects on the expression of the flagellin and hook genes. Isogenic mutants in flbA(flhA), fliI, or fliQ were shown to express drastically reduced levels of FlaA, FlaB, and FlgE, indicating a regulatory effect of the type III secretion system on the production of the exterior flagellar proteins (25, 40, 41). It is possible that in H. pylori the completion of the flagellar export apparatus together with the MS ring acts as a checkpoint for the activation of transcription of σ54- and σ28-dependent late flagellar genes. Such a checkpoint would avoid the wasteful expression of axial proteins of the flagellum in conditions where the specific export apparatus is not present.
Figure 2C represents a simplified model for the mechanisms of flagellar gene regulation in H. pylori. In this model RNA polymerase containing σ80 directs transcription of the master regulator FlgR and of the genes encoding the export apparatus, the chemotaxis genes, and the nonaxial components of the basal body. FlgR in turn activates transcription of σ54-dependent genes encoding the structural proteins of the flagellar rod and hook and represses transcription of the σ28-dependent genes of the filament. Expression of both σ54- and σ28-dependent genes is controlled by a checkpoint in flagellar assembly represented by the export system/MS-ring assembly.
Chemotaxis
The colonization of the mucous layer overlaying the epithelial cells of the stomach is crucial for H. pylori to establish and maintain a persistent infection. The low pH of the stomach lumen and the constant shedding of the mucous layer represent a continuous threat for the bacteria that have to move toward the epithelial cell surface to avoid being washed out by the mucous flow toward the duodenum or killed by the stomach acid. Consequently, it has been postulated that H. pylori has the ability to swim actively toward the mucous layer. Experimental evidence is available showing that H. pylori exhibits chemotactic activity toward various compounds, including the amino acids glutamine, histidine, lysine, and alanine (49), as well as mucin (48), urea, sodium bicarbonate, and sodium chloride (35). The movement of H. pylori toward urea and bicarbonate was found to be markedly enhanced in viscous medium, a condition reflecting the ecological niche of H. pylori, and to be dependent on the presence of a functional urease enzyme (37). As the rotation of the H. pylori flagellum motor was shown to depend on a proton motive force, it has been suggested that the urease-driven hydrolysis of intracellular urea may supply this proton motive force and that chemotactic movement toward urea may provide the substrate for the hydrolysis (37). Urea as well as sodium and bicarbonate ions can be found in large amounts in the mucous layer of the epithelial cells, probably owing to passive diffusion from the bloodstream and active secretion from the mucus and parietal cells, respectively. The presence of a concentration gradient of these metabolites is consistent with a chemotactic response to these compounds that actually would lead to a net movement of the bacteria toward the gastric epithelium.
The regulation of chemotaxis has been extensively studied in the model organisms Escherichia coli and S. enterica serovar Typhimurium (reviewed in references 1, 4, 14, and 33). In these bacteria, sensing of chemoattractants or -repellents is mediated by methyl-accepting chemotaxis proteins (MCP), which possess a periplasmic ligand interaction domain and a large cytoplasmic signaling and adaptation domain. The transduction of the signal from the receptors to the flagellar motor is achieved by four different regulatory proteins: CheA, CheY, CheW, and CheZ (Fig. 1). CheA and CheY constitute a typical two-component system, with CheA being the sensor histidine kinase and CheY the response regulator; CheW is the receptor-coupling factor; and CheZ is an enhancer of CheY-P dephosphorylation. Binding of a ligand to an MCP causes a conformational change in the cytoplasmic domain of the protein, which is recognized by an associated CheA-CheW complex, bound to MCP via CheW. When a repellent is bound to the MCP, autophosphorylation of CheA is greatly stimulated and the phosphotransfer from CheA to CheY is enhanced. CheY-P in turn binds directly to FliM in the flagellar motor switch complex, thereby achieving clockwise rotation of the flagellum that leads to tumbling of the cell. In contrast, attractant-bound MCP causes suppression of CheA activity, which leads to lower levels of CheY-P and therefore to less frequent tumbling and more frequent running of the cell. The same effect is achieved by CheZ, which increases the conversion of CheY-P to CheY, thereby counteracting the clockwise signal.
The chemotactic movement toward attractants is achieved by an adaptation mechanism that involves the methyltransferase CheR and the methylesterase CheB. CheR transfers methyl groups from S-adenosylmethionine to four to six glutamate residues in the MCP, and CheB, after its phosphorylation by CheA, removes these methyl groups. In the absence of chemotactic stimuli MCPs are continuously methylated and demethylated, leading to a basal level of receptor methylation that maintains an intermediate run-tumble behavior. Binding of an attractant to an MCP leads to inhibition of CheA activity and a drop in the CheB-P levels, causing increased methylation of the receptor by CheR that counteracts the attractant-dependent inhibition of CheA activity. The net consequence of this pathway is an adaptation of the receptor, resulting in a return of its signaling state to prestimulus levels. In this way a continuous sensing of concentration gradients of attractants is possible, and the periodical running induced by dephosphorylated CheA (and CheY) leads to a net movement toward the source of attractant.
In the H. pylori genome about 10 genes have been identified that are assumed to be involved in the reception, transduction, and processing of chemotactic signals (3, 47) (Table 1). Homologs of the genes tlpA, tlpB, and tlpC coding for three methyl-accepting chemotaxis proteins have been found, as well as homologs of the regulatory genes cheY and cheW. Interestingly, a bifunctional gene encoding a CheAY fusion protein similar to FRZE of Myxococcus xanthus has also been identified as well as three copies of cheV, a homolog of a Bacillus subtilis gene coding for a protein with an N-terminal CheW domain linked to a response regulator domain of the CheY family. Knockout mutants of both the cheY gene and the bifunctional cheAY gene have been constructed and their motility and chemotactic behavior have been tested. The mutants affecting the bifunctional CheAY protein showed reduced tumbling with respect to the wild type while the CheY mutant showed a rapid-tumbling phenotype (16). All mutants were impaired in their swarming ability on agar plates and in their response to chemotactic signals, indicating the importance of both the CheY and the CheAY proteins in motility of H. pylori (7, 16).
Interestingly, no homologs of CheB or CheR could be identified in the genome of H. pylori, suggesting that the adaptation to chemotactic signals follows different pathways in H. pylori with respect to the ones observed in E. coli or Salmonella sp.
Perspectives
Although many components of the H. pylori flagella have been characterized and data regarding flagellar function and regulation are rapidly increasing, certain aspects of the H. pylori system, in particular those that differ from the well-studied model systems, are still poorly understood and require further investigation. One of these peculiarities is the production of two distinct flagellin subunits (FlaA and FlaB), which are present in different amounts in the flagellar filament and which are regulated differentially at the transcriptional level by a two-component system consisting of the NtrC-like regulator FlgR and a sensor kinase encoded by HP244/jhp229. It will be interesting to investigate the environmental signals that are sensed and transduced by this two-component system. It is tempting to speculate that this system functions as a modulator, which, in response to specific changes in the microenvironment, adapts the ultrastructure of the filament and perhaps other parts of the flagellum in a way that makes them optimally suited for a certain environmental condition. Possibly, the relative amounts of the two flagellin subunits and other hook and hook-associated proteins determine the degree of flexibility or stiffness of the flagellum, and the modulation of these amounts by the two-component system adapts the organelle to changes in the viscosity of the medium.
These regulatory mechanisms appear to act at the bottom of the putative transcriptional hierarchy that governs flagellar biosynthesis in H. pylori. In contrast, the mechanisms at the top of the hierarchy that actually trigger the initiation of flagellar gene transcription are completely unknown. In particular, it is not known whether cell cycle-specific signals (like in Caulobacter sp.) or environmental signals (like in Salmonella sp.) are responsible for the initiation of flagellar gene expression, and master regulators of flagellar gene transcription (like CtrA in Caulobacter sp. or FlhCD in Salmonella sp.) have not been identified in H. pylori. Nevertheless, recent work in our laboratory has provided some indications about the involvement of specific transcription factors in the regulation of flagellar biosynthesis. Mutants in the hspR gene, which codes for a transcriptional repressor of chaperone genes (43), show the same nonmotile phenotype on swarming agar plates as mutants in the flgR gene, which codes for the specific regulator of flagellar rod and hook genes (Fig. 3). Therefore, this regulator seems to have a role in the regulation of flagellar gene transcription in addition to its role in the transcriptional control of stress-responsive genes. It will be interesting to investigate this role and to explore possible interrelationships and cross talks between the regulatory mechanisms that control stress adaptation and those that control flagellar biosynthesis.
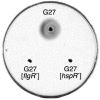
Figure 3
Bacterial motility assay. The indicated strains were stab-inoculated into semisolid (0.3%) agar plates and incubated at 37°C for 3 days.
Acknowledgments
We thank I. Delany for sharing unpublished data, C. Mallia for editing the manuscript, and G. Corsi for artwork.
References
- 1.
- Aizawa S.-I., Harwood C. S., Kadner R. J. Signaling components in bacterial locomotion and sensory reception. J. Bacteriol. 2000;182:1459–1471. [PMC free article: PMC94441] [PubMed: 10692349]
- 2.
- Alm R. A., Guerry P., Trust T. J. The Campylobacter σ54 flaB flagellin promoter is subject to environmental regulation. J. Bacteriol. 1993;175:4448–4455. [PMC free article: PMC204885] [PubMed: 8331072]
- 3.
- Alm R. A., Lee L.-S., Moir D. T., King B. L., Brown E. D., Doig P. C., Smith D. R., Noonan B., Guild B. C., deJonge B. L., Carmel G., Tummino P. J., Caruso A., Uria-Nickelsen M., Mills D. M., Ives C., Gibson R., Merberg D., Mills S. D., Jiang Q., Taylor D. E., Vovis G. F., Trust T. J. Genomic sequence comparison of two unrelated isolates of the human gastric pathogen Helicobacter pylori. Nature. 1999;397:176–180. [PubMed: 9923682]
- 4.
- Amsler, C. D., and P. Matsumura. 1995. Chemotactic signal transduction in Escherichia coli and Salmonella typhimurium, p. 89–103. In A. Hoch and T. J. Silhavy (ed.), Two-Component Signal Transduction. ASM Press, Washington, D.C.
- 5.
- Barinaga M. A shared strategy for virulence. Science. 1996;272:1261–1263. [PubMed: 8650535]
- 6.
- Beier D., Frank R. Molecular characterization of two-component systems of Helicobacter pylori. J. Bacteriol. 2000;182:2068–2076. [PMC free article: PMC111253] [PubMed: 10735847]
- 7.
- Beier D., Spohn G., Rappuoli R., Scarlato V. Identification and characterization of an operon of Helicobacter pylori that is involved in motility and stress adaptation. J. Bacteriol. 1997;179:4676–4683. [PMC free article: PMC179311] [PubMed: 9244252]
- 8.
- Clyne M., Ocroinin T., Suerbaum S., Josenhans C., Drumm B. Adherence of isogenic flagellum-negative mutants of Helicobacter pylori and Helicobacter mustelae to human and ferret gastric epithelial cells. Infect. Immun. 2000;68:4335–4339. [PMC free article: PMC101762] [PubMed: 10858255]
- 9.
- Covacci, A. 1996. Mobilis in mobile: unexpected flexibility and quantum leaps in the Helicobacter pylori genome, p. 40–49. In R. H. Hunt and G. N. J. Tytgat (ed.), Helicobacter pylori—Basic Mechanisms to Clinical Cure. Kluwer Academic Publishers, Dordrecht, The Netherlands.
- 10.
- Dean G. E., Macnab R. M., Stader J., Matsumura P., Burks C. Gene sequence and predicted amino acid sequence of the MotA protein, a membrane-associated protein required for flagellar rotation in Escherichia coli. J. Bacteriol. 1984;159:991–999. [PMC free article: PMC215758] [PubMed: 6090403]
- 11.
- Eaton K. A., Morgan D. R., Krakowka S. Campylobacter pylori virulence factors in gnotobiotic piglets. Infect. Immun. 1989;57:1119–1125. [PMC free article: PMC313239] [PubMed: 2925243]
- 12.
- Eaton K. A., Morgan D. R., Krakowka S. Motility as a factor in the colonisation of gnotobiotic piglets by Helicobacter pylori. J. Med. Microbiol. 1992;37:123–127. [PubMed: 1629897]
- 13.
- Eaton K. A., Suerbaum S., Josenhans C., Krakowka S. Colonization of gnotobiotic piglets by Helicobacter pylori deficient in two flagellin genes. Infect. Immun. 1996;64:2445–2448. [PMC free article: PMC174096] [PubMed: 8698465]
- 14.
- Eisenbach M. Control of chemotaxis. Mol. Microbiol. 1996;20:903–910. [PubMed: 8809743]
- 15.
- Evans D. G., Karjalainen R. K., Evans D. J. J., Graham D. Y., Lee C. H. Cloning, nucleotide sequence and expression of a gene encoding an adhesin subunit protein of Helicobacter pylori. J. Bacteriol. 1993;175:674–683. [PMC free article: PMC196205] [PubMed: 7678592]
- 16.
- Foynes S., Dorrell N., Ward S. J., Stabler R. A., McColm A. A., Rycroft A. N., Wren B. W. Helicobacter pylori possesses two CheY response regulators and a histidine kinase sensor, CheA, which are essential for chemotaxis and colonization of the gastric mucosa. Infect. Immun. 2000;68:2016–2023. [PMC free article: PMC97381] [PubMed: 10722597]
- 17.
- Foynes S., Dorrell N., Ward S. J., Zhang Z. W., McColm A. A., Farthing M. J. G., Wren B. W. Functional analysis of the roles of FliQ and FlhB in flagellar expression in Helicobacter pylori. FEMS Microbiol. Lett. 1999;174:33–39. [PubMed: 10234819]
- 18.
- Francis N. R., Irikura V. M., Yamaguchi S., DeRosier D. J., Macnab R. M. Localization of the S. typhimurium flagellar switch protein FliG in the cytoplasmic M-ring face of the basal-body. Proc. Natl. Acad. Sci. USA. 1992;89:6304–6308. [PMC free article: PMC49489] [PubMed: 1631122]
- 19.
- Francis N. R., Sosinsky G. E., Thomas D., DeRosier D. J. Isolation, characterization and structure of bacterial flagellar motors containing the switch complex. J. Mol. Biol. 1994;235:1261–1270. [PubMed: 8308888]
- 20.
- Geis G., Suerbaum S., Forsthoff B., Leying H., Opferkuch W. Ultrastructure and biochemical studies of the flagellar sheath of Helicobacter pylori. J. Med. Microbiol. 1993;38:371–377. [PubMed: 8487294]
- 21.
- Haas R., Meyer T. F., van Putten J. P. M. Aflagellated mutants of Helicobacter pylori generated by genetic transformation of naturally competent strains using shuttle mutagenesis. Mol. Microbiol. 1993;8:753–760. [PubMed: 8332066]
- 22.
- Homma M., DeRosier D. J., Macnab R. M. Flagellar hook and hook-associated proteins of Salmonella typhimurium and their relationship to other axial components of the flagellum. J. Mol. Biol. 1990;213:819–832. [PubMed: 2193164]
- 23.
- Homma M., Iino T. Locations of hook-associated proteins in flagellar structure of Salmonella typhimurium. J. Bacteriol. 1985;162:183–189. [PMC free article: PMC218972] [PubMed: 3884587]
- 24.
- Homma M., Kutsukake K., Hasebe M., Iino T., Macnab R. M. FlgB, FlgC, FlgF and FlgG. A family of structurally related proteins in the flagellar basal body of Salmonella typhimurium. J. Mol. Biol. 1990;211:465–477. [PubMed: 2129540]
- 25.
- Jenks P. J., Foynes S., Ward S. J., Constantinidou C., Penn C. W., Wren B. W. A flagellar-specific ATPase (FliI) is necessary for flagellar export in Helicobacter pylori. FEMS Microbiol. Lett. 1997;152:205–211. [PubMed: 9231413]
- 26.
- Jones C. J., Homma M., Macnab R. M. L-, P-, and M-ring proteins of the flagellar basal body of Salmonella typhimurium: gene sequences and deduced protein sequences. J. Bacteriol. 1989;171:3890–3900. [PMC free article: PMC210140] [PubMed: 2544561]
- 27.
- Jones A. C., Logan R. P. H., Foynes S., Cockayne A., Wren B. W., Penn C. W. A flagellar sheath protein of Helicobacter pylori is identical to HpaA, a putative N-acetylneuraminidyllactose-binding hemagglutinin, but is not an adhesin for AGS cells. J. Bacteriol. 1997;179:5643–5647. [PMC free article: PMC179448] [PubMed: 9287032]
- 28.
- Josenhans C., Labigne A., Suerbaum S. Comparative ultrastructural and functional studies of Helicobacter pylori and Helicobacter mustelae flagellin mutants: both flagellin subunits, FlaA and FlaB, are necessary for full motility in Helicobacter species. J. Bacteriol. 1995;177:3010–3020. [PMC free article: PMC176987] [PubMed: 7768796]
- 29.
- Kim J. S., Chang J. H., Chung S. I., Yum J. S. Molecular cloning and characterization of the Helicobacter pylori fliD gene, an essential factor in flagellar structure and motility. J. Bacteriol. 1999;181:6969–6976. [PMC free article: PMC94171] [PubMed: 10559162]
- 30.
- Kostrzynska M., Betts J. D., Austin J. W., Trust T. J. Identification, characterization, and spatial localization of two flagellin species in Helicobacter pylori flagella. J. Bacteriol. 1991;173:937–946. [PMC free article: PMC207209] [PubMed: 1704004]
- 31.
- Kustu S., North K., Weiss D. Prokaryotic transcriptional enhancers and enhancer-binding proteins. Trends Biochem. Sci. 1991;16:397–402. [PubMed: 1776167]
- 32.
- Leying H., Suerbaum S., Geis G., Haas R. Cloning and genetic characterization of a Helicobacter pylori flagellin gene. Mol. Microbiol. 1992;6:2863–2874. [PubMed: 1435261]
- 33.
- Macnab, R. M. 1995. Flagellar switch, p. 181–199. In J. A. Hoch and T. J. Silhavy (ed.), Two-Component Signal Transduction. ASM Press, Washington, D.C.
- 34.
- Macnab, R. M. 1996. Flagella and motility, p. 123–145. In F. C. Neidhardt, R. Curtiss III, J. L. Ingraham, E. C. C. Lin, K. B. Low, B. Magasanik, W. S. Reznikoff, M. Riley, M. Schaechter, and H. E. Umbarger (ed.), Escherichia coli and Salmonella: Cellular and Molecular Biology, 2nd ed. ASM Press, Washington, D.C.
- 35.
- Mizote T., Yoshiyama H., Nakazawa T. Urease-independent chemotactic responses of Helicobacter pylori to urea, urease inhibitors, and sodium bicarbonate. Infect. Immun. 1997;65:1519–1521. [PMC free article: PMC175162] [PubMed: 9119496]
- 36.
- Moens S., Vanderleyden J. Functions of bacterial flagella. Crit. Rev. Microbiol. 1996;22:67–100. [PubMed: 8817078]
- 37.
- Nakamura H., Yoshiyama H., Takeuchi H., Mizote T., Okita K., Nakazawa T. Urease plays an important role in the chemotactic motility of Helicobacter pylori in a viscous environment. Infect. Immun. 1998;66:4832–4837. [PMC free article: PMC108597] [PubMed: 9746586]
- 38.
- O'Toole P. W., Kostrzynska M., Trust T. J. Nonmotile mutants of Helicobacter pylori and Helicobacter mustelae defective in flagellar hook production. Mol. Microbiol. 1994;14:691–703. [PubMed: 7891557]
- 39.
- Ottemann K. M., Miller J. F. Roles for motility in bacterial-host interactions. Mol. Microbiol. 1997;24:1109–1117. [PubMed: 9218761]
- 40.
- Porwollik S., Noonan B., O'Toole P. W. Molecular characterization of a flagellar export locus of Helicobacter pylori. Infect. Immun. 1999;67:2060–2070. [PMC free article: PMC115938] [PubMed: 10225855]
- 41.
- Schmitz A., Josenhans C., Suerbaum S. 1997Cloning and characterization of the Helicobacter pylori flbA gene, which codes for a membrane protein involved in coordinated expression of flagellar genes J. Bacteriol 179987–997., 42. [PMC free article: PMC178789] [PubMed: 9023175]
- 42.
- Spohn G., Scarlato V. Motility of Helicobacter pylori is coordinately regulated by the transcriptional activator FlgR, an NtrC homolog. J. Bacteriol. 1999;181:593–599. [PMC free article: PMC93415] [PubMed: 9882675]
- 43.
- Spohn G., Scarlato V. The autoregulatory HspR repressor protein governs chaperone gene transcription in Helicobacter pylori. Mol. Microbiol. 1999;34:663–674. [PubMed: 10564507]
- 44.
- Stader J., Matsumura P., Vacante D., Dean G. E., Macnab R. M. Nucleotide sequence of the Escherichia coli motB gene and site-limited incorporation of its product into the cytoplasmic membrane. J. Bacteriol. 1986;166:244–252. [PMC free article: PMC214583] [PubMed: 3007435]
- 45.
- Suerbaum S. The complex flagella of gastric Helicobacter species. Trends Microbiol. 1995;3:168–170. [PubMed: 7627454]
- 46.
- Suerbaum S., Josenbaus C., Labigne A. Cloning and genetic characterization of the Helicobacter pylori and Helicobacter mustelae flagellin genes and construction of H. pylori flaA- and flaB-negative mutants by electroporation-mediated allelic exchange. J. Bacteriol. 1993;175:3278–3288. [PMC free article: PMC204724] [PubMed: 8501031]
- 47.
- Tomb J.-F., White O., Kerlavage A. R., Clayton R. A., Sutton G. G., Fleischmann R. D., Ketchum K. A., Klenk H. P., Gill S., Dougherty B. A., Nelson K., Quackenbush J., Zhou L., Kirkness E. F., Peterson S., Loftus B., Richardson D., Dodson R., Khalak H. G., Glodek A., McKenney K., Fitzegerald L. M., Lee N., Adams M. D., Hickey E. K., Berg D. E., Gocayne J. D., Utterback T. R., Peterson J. D., Kelley J. M., Cotton M. D., Weidman J. M., Fujii C., Bowman C., Watthey L., Wallin E., Hayes W. S., Borodovsky M., Karp P. D., Smith H. O., Fraser C. M., Venter J. C. The complete genome sequence of the gastric pathogen Helicobacter pylori. Nature. 1997;388:539–547. [PubMed: 9252185]
- 48.
- Turner G. A., Logan R. P. H., Chinnery R., Cockayne A., Hawkey C. J., Borriello S. P. Trefoil peptides are unique chemotaxins for Helicobacter pylori. Gastroenterology. 1997;112:A1107.
- 49.
- Worku M., Sidebotham R. L., Wren B. W., Karim Q. N. 1997Chemotaxis of H. pylori in presence of human plasma Gut 41A25 (abstract).
- 50.
- Wu J., Newton A. Regulation of the Caulobacter flagellar gene hierarchy; not just for motility. Mol. Microbiol. 1997;24:233–239. [PubMed: 9159510]
- 51.
- Yokoseki T., Kutsukake K., Ohnishi K., Iino T. Functional analysis of the flagellar genes in the fliD operon of Salmonella typhimurium. Microbiology. 1995;141:1715–1722. [PubMed: 7551038]
- ChePep controls Helicobacter pylori Infection of the gastric glands and chemotaxis in the Epsilonproteobacteria.[mBio. 2011]ChePep controls Helicobacter pylori Infection of the gastric glands and chemotaxis in the Epsilonproteobacteria.Howitt MR, Lee JY, Lertsethtakarn P, Vogelmann R, Joubert LM, Ottemann KM, Amieva MR. mBio. 2011; 2(4). Epub 2011 Jul 26.
- Imaging the motility and chemotaxis machineries in Helicobacter pylori by cryo-electron tomography.[J Bacteriol. 2017]Imaging the motility and chemotaxis machineries in Helicobacter pylori by cryo-electron tomography.Qin Z, Lin WT, Zhu S, Franco AT, Liu J. J Bacteriol. 2017 Feb; 199(3):e00695-16. Epub 2016 Nov 14.
- Loss of a Cardiolipin Synthase in Helicobacter pylori G27 Blocks Flagellum Assembly.[J Bacteriol. 2019]Loss of a Cardiolipin Synthase in Helicobacter pylori G27 Blocks Flagellum Assembly.Chu JK, Zhu S, Herrera CM, Henderson JC, Liu J, Trent MS, Hoover TR. J Bacteriol. 2019 Nov 1; 201(21). Epub 2019 Oct 4.
- Review Dual flagellar systems enable motility under different circumstances.[J Mol Microbiol Biotechnol. 2004]Review Dual flagellar systems enable motility under different circumstances.McCarter LL. J Mol Microbiol Biotechnol. 2004; 7(1-2):18-29.
- Review Motility of Different Gastric Helicobacter spp.[Microorganisms. 2023]Review Motility of Different Gastric Helicobacter spp.Bansil R, Constantino MA, Su-Arcaro C, Liao W, Shen Z, Fox JG. Microorganisms. 2023 Mar 1; 11(3). Epub 2023 Mar 1.
- Motility, Chemotaxis, and Flagella - Helicobacter pyloriMotility, Chemotaxis, and Flagella - Helicobacter pylori
Your browsing activity is empty.
Activity recording is turned off.
See more...