The content of this book is licensed under a Creative Commons Attribution-NonCommercial-NoDerivs 4.0 Unported license. To view the terms and conditions of this license, visit https://creativecommons.org/licenses/by-nc-nd/4.0/
NCBI Bookshelf. A service of the National Library of Medicine, National Institutes of Health.
Varki A, Cummings RD, Esko JD, et al., editors. Essentials of Glycobiology [Internet]. 4th edition. Cold Spring Harbor (NY): Cold Spring Harbor Laboratory Press; 2022. doi: 10.1101/glycobiology.4e.27
This chapter discusses some general features of glycosylation and glycan-binding interactions in a few examples of species that belong to the deuterostome lineage, with particular emphasis on sea urchins, frogs, zebrafish, and mice. These organisms provide excellent models for studying the functions of glycans in development and physiology.
EVOLUTIONARY BACKGROUND
Animal evolution split into two major lineages ∼500–600 million years ago—the deuterostomes and the protostomes (Chapter 20). The superphylum Deuterostomia contains the major phyla of Echinodermata (sea urchins and starfish), Hemichordata (acorn worms), Urochordata (ascidians), Cephalochordata (amphioxus), and Vertebrata (fish, amphibia, reptiles, birds, and mammals). In deuterostomes, cell divisions of the zygote occur by radial cleavage, and cell fates are not precisely determined. Another characteristic feature is that the first opening to form in the blastula becomes the anus and the second opening becomes the mouth. The other superphylum, the Protostomia, contains the major phyla of Porifera (corals and sponges), Cnidara (anemones and hydra), Annelida (segmented worms), Mollusca (clams, oysters, snails, and slugs), and Arthropoda (insects, spiders, and crustacea). In protostomes, unlike deuterostomes, cell division during early development occurs in a highly organized manner and cell fate is precisely determined. Examples of well-studied protostome model organisms include the nematode Caenorhabditis elegans (Chapter 25) and the arthropod Drosophila melanogaster (Chapter 26).
Deuterostomes have been extensively studied because of their relatedness to humans and because they provide excellent model systems for understanding vertebrate biology. Sea urchins, frogs, zebrafish, and mice have received the most attention and each provides certain advantages for studying functions of glycans in reproduction, early development, or adult physiology. In numerous instances, these models have revealed aspects of glycobiology that were later confirmed in more evolutionarily distant organisms, and they often serve as excellent models for understanding human disease. Challenges exist, however, in describing the glycomes of the more ancient deuterostomes, mainly because of poor genome annotation, ignorance of individual glycosyltransferase substrates, and the presence of glycans with unusual structures. Each of these models is briefly described below with references to other sections of the book for additional details.
SEA URCHINS
Sea urchin glycans involved in fertilization have been studied extensively. In fact, much of what we know about the biochemistry of fertilization was first discovered in this organism (Figure 27.1), and the information subsequently applied to mammalian sperm–egg interactions. One of the advantages of studying fertilization in sea urchins is that eggs and sperm are easy to obtain in large quantities. Because fertilization occurs outside the adult body, it is also easy to experimentally manipulate sperm and eggs.
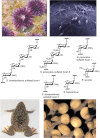
FIGURE 27.1.
(Top left) The purple sea urchin Strongylocentrotus purpuratus. (Reprinted, with permission, courtesy of Charles Hollahan, Santa Barbara Marine Biologicals.) (Top right) Sperm binding to a sea urchin egg. (Reprinted, with permission, courtesy of M. Tegner (more...)
Egg Glycans and Fertilization
Sea urchin eggs are covered by a hydrated jelly coat. About 80% of the weight of egg jelly is a high-molecular-weight linear fucose (Fuc) sulfate polymer (FSP) with a molecular mass of >106 Da. Receptor proteins on sperm bind to FSP, triggering the opening of two pharmacologically distinct calcium channels that induce the exocytosis of the sperm's acrosome vesicle (the “acrosome reaction”). The ionic mechanisms that trigger the acrosome reaction are conserved in mammals, but the nature of the sperm surface receptors varies. FSP is a species-selective inducer of the sea urchin sperm acrosomal reaction and most FSPs are sulfated α1-3Fuc-based linear polymers made of tri- or tetrasaccharide repeats. The number of fucoses per repeat, the linkage, and sulfation patterns all help ensure species selectivity for induction of the acrosome reaction. About 20% of the egg jelly mass is a large glycoprotein containing a unique polymer of sialic acid (Sia), which can be released from crude sea urchin egg jelly by treatment with mild base (β-elimination). The sialoglycan has a novel structure—[Neu5Gcα2-5-O-glycolylNeu5Gc]n. However, its receptor on the sperm membrane is unknown. When sea urchin sperm undergo the acrosome reaction, a protein named bindin is released from the acrosomal vesicle. Bindin cements the sperm to a large egg surface glycoprotein named egg bindin receptor 1 (EBR1). Bindin can agglutinate mammalian red blood cells much like plant lectins (Chapter 32), and glycopeptide fragments of unfertilized eggs can block bindin-induced red cell agglutination. Bindin is thought to recognize glycans on EBR1. EBR1 itself has lectin-like domains, but its glycan ligands are unknown.
Most studies of glycosylation in sea urchins have identified glycosyltransferase activities and the glycans they synthesize (e.g., including N-glycans with antennal β1-3-linked Gal, β1-4GalNAc, Neu5Gc, and sulphate residues as well as traces of the “invertebrate” feature of core α1-3/α1-6-difucosylation) (Figure 27.2). The functions of glycans and glycan-binding proteins (GBPs) can be investigated using genetic strategies in sea urchins, antisense morpholinos or short hairpin RNAs (shRNAs) to knock down gene expression have been the methods of choice, but these will likely be superseded by more precise gene editing techniques such as the clustered regularly interspaced short palindromic repeats/Cas9 (CRISPR/Cas9) strategy or transcription activator–like effector (TALE) nucleases (TALENs). A few echinoderm genomes have been sequenced (Echinobase), but as with most organisms, relating glycosylation activities to genes is not straightforward (Chapter 8).
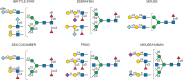
FIGURE 27.2.
N-glycan diversity in deuterostomes. Example N-glycan antennae from a brittle star and a sea cucumber (both echinoderms), zebrafish, the African clawed frog, and the mouse are shown as well as those shared between mouse and humans. ±S signifies (more...)
FROGS
The African clawed frog, Xenopus laevis, is a well-established model organism for studying fertilization and embryonic development (Figure 27.1). Adults are easy to maintain and can be induced to lay eggs up to three times a year by injection of human chorionic gonadotropin. The very large eggs allow easy microinjection experiments to express exogenous cDNAs encoding RNAs and proteins of interest. Although frog embryos develop quickly, the generation time for adults is long (1–2 yr), and the frog genome is tetraploid, making genetic studies difficult. Nevertheless, as for sea urchins, inhibition of gene expression has been accomplished by injection of antisense morpholinos or shRNAs into early embryos. These techniques suffer from off-target effects and the future will see a shift to newer gene editing strategies including CRISPR/Cas9 and TALENs. In addition, in situ hybridization and overexpression techniques have been used in Xenopus embryos. For example, the functions of two polypeptide N-acetylgalactosaminyltransferases in transforming growth factor-β/bone morphogenic protein signaling were distinguished, showing that this organism is useful for discovering glycan biosynthetic steps and mechanisms by which glycans regulate development. Indeed, developmental changes in the X. laevis N-glycome (Figure 27.2) have been reported, whereas the O-glycans of frog egg mucins can be extremely complicated in a species-dependent manner.
Lectin Family First Discovered in Xenopus
X. laevis oocyte cortical granules contain a lectin called XL35, which is released at fertilization and binds to glycoproteins expressing mucin-type O-glycans (Galα1-3GalNAc) in the jelly surrounding the egg. XL35 is an oligomer made up of monomers, each of which has a molecular weight of 35 kDa. Light scattering experiments showed that the oligomer exists primarily as a 12-mer, allowing it to cross-link its glycoprotein ligands in the egg jelly to form a relatively rigid layer on the fertilization envelope. This molecular complex functions to block polyspermy and also serves as a barrier against microbial infection. Homologs of XL35, known as “X-lectins,” exist in some of the most primitive deuterostomes, such as the sea squirt, but also in more complex vertebrates such as zebrafish, mice, and humans. Mice and humans have two orthologs termed intelectins-1 and -2 (Chapter 30) because of their initial discovery in the intestines. It is interesting to note that the sea squirt homolog (Halocynthia roretzi) shows a similar monomeric molecular weight to human intelectin 1 (Hint-1) and is 34% identical at the amino acid level. Hint-1 exists as a trimer when secreted from goblet cells in intestinal and respiratory epithelia. IL-13, a cytokine involved in the type TII innate immune response, induces a remarkable increase in Hint-1 transcripts. Constitutively expressed transcripts for Hint-1 are also found in heart and lung tissue. Hint-2, in contrast, is only expressed in Paneth cells that function in immune surveillance in the crypts of the small intestine. Glycan microarray results show that both Hints bind to the glycans of a unique set of human pathogenic bacteria that have in common the presence of particular cis-diols on their outer surface polysaccharides and include both Gram-positive and Gram-negative bacteria. Crystal structures of Hint-1 and the Xenopus embryonic epidermal lectin show a conserved mechanism of glycan recognition. But clearly, the carbohydrate binding determinants for each intelectin studied are unique. It is becoming clear that the X-lectin family plays key roles in deuterostome innate immune responses against pathogens.
ZEBRAFISH
Zebrafish (Danio rerio) provides an excellent model organism for understanding the functions of genes in early vertebrate development (Figure 27.3). Females lay several hundred eggs weekly, which undergo external fertilization. Embryos develop rapidly (in hours), and their translucence allows visualization of early development. Zebrafish are relatively easy to maintain compared with other vertebrate species. Furthermore, they can be mutagenized and outbred to produce mutant strains or manipulated using morpholinos, shRNAs, or CRISPR/Cas9 and TALEN methodologies to silence or delete genes permanently or transiently during early development (Figure 27.3).
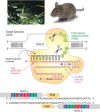
FIGURE 27.3.
(Top left) Zebrafish (Danio rerio). (Photograph from the Zebrafish Information Network [ZFIN], © University of Oregon; see also Sprague J, et al. 2006. Nucl Acids Res 34: D581–D585.) (Top right) Adult mouse (Mus musculus). (Middle) Gene (more...)
Zebrafish live in a nonterrestrial environment and widespread gene duplications and diversification occurred during teleost evolution. All major glycan classes present in mammals have been described in zebrafish and many of them have been manipulated genetically, yielding new insights into glycan functions. Indeed, the N-, O-, and lipid-linked glycomes of zebrafish organs have a range of typical vertebrate features such as β1-4-galactosylation and sialylation, but also a unique β1-4-galactose extension of the “sialyl-Lewis x” epitope (Figure 27.2).
Investigation of Glycosylation-Related Phenotypes
Sophisticated screening methods for the identification of novel mutants that affect specific physiological systems may be applied to zebrafish. For example, high-throughput screenings have identified groups of genes required for fin development and regeneration, which serve as models for cartilage and bone development in mammals. Mutants altered in vascular development and hemostasis have been identified as well. Moreover, the ability to identify novel genes in the system and manipulate glycan expression via gene silencing or mutation is making this model organism a focus of studies in vertebrate glycobiology. Functions of particular protein- and lipid-bound glycans have been uncovered; for example, the slytherin mutant that contains a missense mutation affecting GDP-fucose biosynthesis causes several phenotypes impacting neuronal differentiation. Knockdown of genes that regulate polysialylation show the critical function of polysialic acid for neuronal migration and plasticity, including those that may involve motor neurons in somite formation and differentiation. Significant contributions have also been made in the understanding of glycosaminoglycan (GAG) biosynthesis (Chapter 17) and functions during development. Furthermore, the modeling of several human congenital disorders of glycosylation in zebrafish has provided insights into the phenotypes associated with these disorders, including defects in the synthesis of O-mannose glycans (Chapter 13) that give rise to forms of muscular dystrophy (Chapter 45).
MICE
Laboratory mice (Mus musculus) are the most widely studied deuterostomes because they are the mammals that are most closely related to primates, reproduce quickly, and their genes can be readily manipulated. Inbred mouse strains are widespread, and spontaneous mutations occur that have specific morphological or pathophysiological phenotypes. Examples of the latter include brachymorphic mice, which are partially deficient in synthesis of PAPS, the universal sulfate donor (Chapter 5), resulting in shortened limbs because of defective chondroitin sulfate (CS) synthesis during endochondral ossification (Chapter 17). Another example is a mutation causing muscular dystrophy, which was traced to Largemyd, a glycosyltransferase that when defective in humans also causes muscular dystrophy (see Chapters 13 and 45). Much information about mouse glycobiology comes from the use of homologous recombination methods to knock out a specific gene systemically, in specific tissues or at selected times in development (Online Appendix 27A). In numerous cases, human pathological mutations have been engineered into conserved murine homologous genes to generate models for disease (Chapter 41). Notably, humans lack two glycan biosynthetic enzymes that are found in mice: the galactosyltransferase that synthesizes Galα1-3Gal that can terminate N- or O-glycans (Figure 27.2; Chapter 14), and the CMP-Neu5Ac hydroxylase that synthesizes CMP-N-glycolylneuraminic acid (Neu5Gc) from CMP-Neu5Ac (Chapter 15). Mice lack the ABH(O) blood group glycans of humans (Chapter 14).
Mutations That Affect Entire Pathways
Because gene deletion has been used to inactivate numerous genes involved in glycosylation, only a few mutants are mentioned here. Mutant lines have been created in early genes involved in the assembly of all the major subclasses of vertebrate glycans. In most cases, these mutations prevent initiation of the biosynthetic pathways and show embryonic lethality. These include the O-GlcNAc transferase OGT (Chapter 19), whose deletion is lethal even at the single-cell stage. Loss of the entire N-glycan pathway caused by ablation of phosphomannomutase 2 (which generates mannose-1-P and all mannose-containing glycans), and loss of GlcNAc-1-P transferase (which initiates the lipid-linked oligosaccharide precursor; Chapter 9) are lethal within a few days of fertilization. Loss of heparan sulfate (HS) biosynthesis due to deletion of Ext1 or Ext2 is also lethal at the E6–7 stage because of a failure to differentiate mesoderm. Systemic deletion of MGAT1 (Chapter 9) is lethal around midgestation (E9–10) showing that early development through gastrulation can proceed in the complete absence of hybrid and complex N-glycans. CS and hyaluronan synthesis are essential for embryonic development as well, and developmental arrest occurs during limb generation and organogenesis. Because there are more than 20 partially redundant polypeptide N-acetylgalactosaminyltransferases in mice, knocking out the O-GalNAc glycan pathway (Chapter 10) completely has not been possible. However, deletion of the single copy core-1 galactosyltransferase C1GALT1, which generates Galβ1-3GalNAcα-O-Ser/Thr, is lethal by stage E14 because of abnormal angiogenesis. Knocking out the protein O-fucosyltransferase POFUT1 that modifies epidermal growth factor (EGF) modules in Notch is lethal and causes developmental defects similar to those seen in global Notch pathway knockout mice. Absence of glycosylphosphatidylinositol (GPI) anchors (Chapter 12) or O-mannose glycans (Chapter 13) also result in embryonic lethality.
Mutations That Affect Specific Glycan Extensions or Modifications
Mutations in genes that encode glycosyltransferases that extend core glycans in each class of glycan usually have less severe phenotypes than those deleting entire pathways. For example, some glycosyltransferase gene knockouts affect the immune system, including leukocyte rolling, lymphocyte homing, and T-cell homeostasis. Knocking out sequential biosynthetic steps or multiple, seemingly redundant genes frequently reveals another layer of glycan-dependent functions, novel structures, and unrecognized biosynthetic pathways. Many mouse mutants serve as models of human genetic disorders (Chapter 41).
Cell Type–Specific Mutations
To study the function of glycans in specific tissues, tissue-specific lesions in a gene can be created by crossing strains bearing conditional null alleles to strains that selectively delete the gene only in those tissues of interest. The most common method is to flank a portion of the gene with loxP recombination sites and then mate these mice to a strain that produces bacteriophage Cre-recombinase under the control of a tissue-specific or inducible promoter (Figure 27.4). When activated, the Cre-recombinase recognizes the loxP sites and excises the DNA segment contained between them. These techniques can be combined with an environmental stress, dietary modification, or inflammatory challenge to delete genes in both a cell type–specific and temporally regulated fashion.
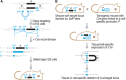
FIGURE 27.4.
Cre-loxP targeting for making conditional gene knockouts in the mouse.
Sometimes, altering a gene can have a highly specific effect on a given tissue without affecting other tissues because of the unforeseen presence of related isozymes. For example, Golgi α-mannosidase II (MAN2A1) was thought to have the dominant role in N-glycan processing (see Chapter 9), but mice lacking MAN2A1 survive and have a dyserythropoietic anemia. These mice produce many unusual glycans from the abnormally high levels of incomplete precursors that accumulate and drive the synthesis of atypical products. The mutant mice eventually develop a progressive autoimmune nephropathy, accumulating immune complexes in the kidney tubules, probably in response to the abnormal glycan antigens. Their erythrocytes lack complex N-glycans, but other tissues continue to produce them using a different isozyme called α-mannosidase IIx (MAN2A2). Mice lacking MAN2A2 are mostly unaffected, but male mutant mice are sterile because spermatocytes fail to make a glycan required for their binding to Sertoli cells in the testes, blocking spermatogenesis. Deletion of both α-mannosidases prevents synthesis of all complex N-glycans (Chapter 9), and most embryos die between E15 and E18, or shortly after birth from respiratory failure.
Strain Differences and Genetic Modifiers
Determining the effect of a mutant gene is usually done in highly inbred strains that are genetically identical except for the gene in question. Sometimes, the phenotype of a mutation changes according to the genetic background (mouse strain) in which the mutation is expressed. For instance, mice lacking N-acetylglucosaminyltransferase II (MGAT2), an enzyme required for complex N-glycan synthesis (Chapter 9), die after birth in one genetic background, but in another, rare survivors occur, with severe gastrointestinal, hematological, and osteogenic abnormalities. Interestingly, these mutants phenocopy the human disorder, CDG-IIa, in which patients lack the same enzyme (Chapter 45). As another example, lines deficient in global fucosylation because of mutations in the enzyme that converts GDP-mannose to GDP-fucose (Chapter 5) die as embryos, but when bred into a different background, they survive until birth and can then be maintained on a fucose-supplemented diet. A third example is the formation of bony outgrowths (exostoses) in the growth plates of endochondral bones. In one background, heterozygous mutations in the Ext-1 copolymerase involved in HS biosynthesis cause infrequent exostoses, whereas in another background, the incidence of tumors increases severalfold. These experiments suggest the existence of modifiers that alter the expression level of an enzyme or the assembly of substrate, that salvage sugars from the diet, or that alter glycan turnover.
Environmentally Driven Phenotypes
In some mutants, phenotypes are only evident under environmental challenge. For example, deletion of both enzymes responsible for polysialic acid synthesis produces “no-fear” mice that tend to be more aggressive and ignore normally stressful or anxiety-producing situations. Deletion of syndecan-1, a major HS core protein (Chapter 17), gives no obvious changes under normal laboratory conditions, but the mice are more resistant to bacterial lung infections. Apparently, bacteria exploit the shed syndecan in the lung to enhance their virulence and modulate host defenses.
Mice deficient in the synthesis of ganglioside GM3 appear to be normal except for enhanced insulin sensitivity, as well as deafness due to inner ear hair cell defects. These mice shunt glycosphingolipid synthesis toward more complex gangliosides that substitute for GM3 loss (Chapter 11). On the other hand, double-mutant mice that synthesize only ganglioside GM3 show tonic–clonic (epileptic) seizures, and 90% die because of seizures in response to sharp sounds. These examples show the importance of having the correct balance of glycans. They also show how phenotypes depend to a large extent on other genetic factors, diet, and environmental cues. Another major factor affecting the phenotype is now thought to be the microbiome, especially in the gut. Thus, mutations that appear silent in a controlled laboratory setting may elicit a strong phenotype in a more natural environment.
HUMANS AND OTHER PRIMATES
Studies of humans and other primates have been limited by cost, ethical controversies, and (in the case of humans) genetically outbred populations. However, there are increasing examples in which findings (especially therapeutic ones) in mice fail to translate to the human conditions. Even chimpanzees (our closest relatives) seem to have markedly different disease profiles, some explained by alterations in sialic acid biology. Although ethically and practically feasible studies on other primates should continue, the late Nobel laureate Sydney Brenner has suggested (Chapter 7) that we now have enough information about humans to consider ourselves to be a viable “model organism” for in-depth study.
ACKNOWLEDGMENTS
The authors acknowledge contributions to previous versions of this chapter by Victor Vacquier and appreciate helpful comments and suggestions from Ajit Varki and Vlad Panin.
FURTHER READING
- Lee JK, Baum LG, Moremen K, Pierce M. 2004. The X-lectins: a new family with homology to the Xenopus laevis oocyte lectin XL-35. Glycoconj J 21: 443–450. doi:10.1007/s10719-004-5534-6 [PubMed: 15750785] [CrossRef]
- Ohtsubo K, Marth JD. 2006. Glycosylation in cellular mechanisms of health and disease. Cell 126: 855–867. doi:10.1016/j.cell.2006.08.019 [PubMed: 16959566] [CrossRef]
- Bishop JR, Schuksz M, Esko JD. 2007. Heparan sulphate proteoglycans fine-tune mammalian physiology. Nature 446: 1030–1037. doi:10.1038/nature05817 [PubMed: 17460664] [CrossRef]
- Freeze HH, Sharma V. 2010. Metabolic manipulation of glycosylation disorders in humans and animal models. Semin Cell Dev Biol 21: 655–662. doi:10.1016/j.semcdb.2010.03.011 [PMC free article: PMC2917643] [PubMed: 20363348] [CrossRef]
- Varki NM, Strobert E, Dick EJ Jr, Benirschke K, Varki A. 2011. Biomedical differences between human and nonhuman hominids: potential roles for uniquely human aspects of sialic acid biology. Annu Rev Pathol 6: 365–393. doi:10.1146/annurev-pathol-011110-130315 [PubMed: 21073341] [CrossRef]
- Vacquier VD. 2012. The quest for the sea urchin egg receptor for sperm. Biochem Biophys Res Commun 425: 583–587. doi:10.1016/j.bbrc.2012.07.132 [PubMed: 22925678] [CrossRef]
- Flanagan-Steet HR, Steet R. 2013. “Casting” light on the role of glycosylation during embryonic development: insights from zebrafish. Glycoconj J 30: 33–40. doi:10.1007/s10719-012-9390-5 [PMC free article: PMC3718303] [PubMed: 22638861] [CrossRef]
- Bammens R, Mehta N, Race V, Foulquier F, Jaeken J, Tiemeyer M, Steet R, Matthijs G, Flanagan-Steet H. 2015. Abnormal cartilage development and altered N-glycosylation in Tmem165-deficient zebrafish mirrors the phenotypes associated with TMEM165-CDG. Glycobiology 25: 669–682. doi:10.1093/glycob/cwv009 [PMC free article: PMC4410832] [PubMed: 25609749] [CrossRef]
- Voglmeir J, Laurent N, Flitsch SL, Oelgeschlager M, Wilson IB. 2015. Biological and biochemical properties of two Xenopus laevis N-acetylgalactosaminyltransferases with contrasting roles in embryogenesis. Comp Biochem Physiol B Biochem Mol Biol 180: 40–47. doi:10.1016/j.cbpb.2014.10.003 [PMC free article: PMC4291152] [PubMed: 25447273] [CrossRef]
- Wesener DA, Wangkanont K, McBride R, Song X, Kraft MB, Hodges HL, Zarling LC, Splain RA, Smith DF, Cummings RD, et al. 2015. Recognition of microbial glycans by human intelectin-1. Nat Struct Mol Biol 22: 603–610. doi:10.1038/nsmb.3053 [PMC free article: PMC4526365] [PubMed: 26148048] [CrossRef]
- Wangkanont K, Wesener DA, Vidani JA, Kiessling LL, Forest KT. 2016. Structures of Xenopus embryonic epidermal lectin reveal of conserved mechanism of microbial glycan recognition. J Biol Chem 291: 5596–5610. doi:10.1074/jbc.m115.709212 [PMC free article: PMC4786701] [PubMed: 26755729] [CrossRef]
- Stanley P. 2017. What have we learned from glycosyltransferase knockouts in mice? J Mol Biol 428: 3166–3182. doi:10.1016/j.jmb.2016.03.025 [PMC free article: PMC5532804] [PubMed: 27040397] [CrossRef]
- Yamakawa N, Vanbeselaere J, Chang LY, Yu SY, Ducrocq L, Harduin-Lepers A, Kurata J, Aoki-Kinoshita KF, Sato C, Khoo KH, et al. 2018. Systems glycomics of adult zebrafish identifies organ-specific sialylation and glycosylation patterns. Nat Commun 9: 4647. doi:10.1038/s41467-018-06950-3 [PMC free article: PMC6220181] [PubMed: 30405127] [CrossRef]
- Sigal M, Del Mar Reinés M, Müllerké S, Fischer C, Kapalczynska M, Berger H, Bakker ERM, Mollenkopf H-J, Rothenberg ME, Wiedenmann B, et al. 2019. R-spondin-3 induces secretory, antimicrobial Lgr5+ cells in the stomach. Nat Cell Biol 21: 812–823. doi:10.1038/s41556-019-0339-9 [PubMed: 31235935] [CrossRef]
- Eckmair B, Jin C, Karlsson NG, Abed-Navandi D, Wilson IBH, Paschinger K. 2020. Glycosylation at an evolutionary nexus: the brittle star Ophiactis savignyi expresses both vertebrate and invertebrate N-glycomic features. J Biol Chem 295: 3173–3188. doi:10.1074/jbc.ra119.011703 [PMC free article: PMC7062179] [PubMed: 32001617] [CrossRef]
- Review Deuterostomes.[Essentials of Glycobiology. 2015]Review Deuterostomes.Pierce M, Stanley P. Essentials of Glycobiology. 2015
- Review Deuterostomes.[Essentials of Glycobiology. 2009]Review Deuterostomes.Freeze HH, Vacquier VD, Esko JD. Essentials of Glycobiology. 2009
- Review Nodal signaling and the evolution of deuterostome gastrulation.[Dev Dyn. 2005]Review Nodal signaling and the evolution of deuterostome gastrulation.Chea HK, Wright CV, Swalla BJ. Dev Dyn. 2005 Oct; 234(2):269-78.
- Investigation of glycan evolution based on a comprehensive analysis of glycosyltransferases using phylogenetic profiling.[Biophys Physicobiol. 2015]Investigation of glycan evolution based on a comprehensive analysis of glycosyltransferases using phylogenetic profiling.Tomono T, Kojima H, Fukuchi S, Tohsato Y, Ito M. Biophys Physicobiol. 2015; 12:57-68. Epub 2015 Nov 12.
- Review Heads or tails? Amphioxus and the evolution of anterior-posterior patterning in deuterostomes.[Dev Biol. 2002]Review Heads or tails? Amphioxus and the evolution of anterior-posterior patterning in deuterostomes.Holland LZ. Dev Biol. 2002 Jan 15; 241(2):209-28.
- Deuterostomes - Essentials of GlycobiologyDeuterostomes - Essentials of Glycobiology
- O-GalNAc Glycans - Essentials of GlycobiologyO-GalNAc Glycans - Essentials of Glycobiology
- Chemical Tools for Inhibiting Glycosylation - Essentials of GlycobiologyChemical Tools for Inhibiting Glycosylation - Essentials of Glycobiology
Your browsing activity is empty.
Activity recording is turned off.
See more...