NCBI Bookshelf. A service of the National Library of Medicine, National Institutes of Health.
Varki A, Cummings RD, Esko JD, et al., editors. Essentials of Glycobiology. 2nd edition. Cold Spring Harbor (NY): Cold Spring Harbor Laboratory Press; 2009.
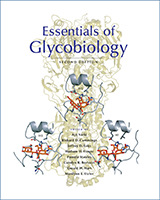
Essentials of Glycobiology. 2nd edition.
Show detailsThis chapter discusses various types of inhibitors, including natural products, substrate-based tight-binding inhibitors, glycoside primers, monosaccharide analogs for chemically tagging glycans, inhibitors found through screening chemical libraries, and examples of rationally designed inhibitors based on three-dimensional structures of enzymes (Table 50.1).
TABLE 50.1
Classes of inhibitors
ADVANTAGES OF INHIBITORS
Chapters 41, 42, and 46 describe various natural and induced mutants with defects in glycosylation. These mutants have helped to define genes that encode various transferases and glycosidases, and in some cases alternate biosynthetic pathways have been uncovered. Mutants also provide insights into the functions of glycosylation in cells and tissues as well as models for human inborn errors in metabolism and disease. However, one limitation of studying mutants is that the analyses are usually restricted to the cell or organism from which the mutant strain was isolated. Additionally, many mutations are lethal in animals, which makes the study of the gene in adult animals more difficult.
Inhibitors of glycosyltransferases and glycosidases provide another approach for studying glycosylation in cells, tissues, and whole organisms that avoids some of the problems associated with studying mutants. Many of these compounds are small molecules that are taken up readily by a variety of cell types and can be absorbed through the gut, which provides an opportunity for designing drugs to treat human diseases and disorders correlated with altered glycosylation (see Chapter 51). Because the field is quite large, only those inhibitors that act on specific enzymes or metabolic pathways and that illustrate certain basic concepts are discussed here (Table 50.1). Agents that block protein/carbohydrate interactions are surveyed in Chapter 27, and the aminoglycoside antibiotics are discussed briefly in Chapter 51.
INDIRECT INHIBITORS AND METABOLIC POISONS
A number of inhibitors have been described that block glycosylation by interfering with the metabolism of common precursors or intracellular transport activities. Some of these compounds act indirectly by impeding the transit of proteins between the endoplasmic reticulum (ER), Golgi, and trans-Golgi network. For example, the fungal metabolite brefeldin A causes retrograde transport of Golgi components located proximal to the trans-Golgi network back to the ER. Thus, treating cells with brefeldin A separates enzymes located in the trans-Golgi network from those found in the ER and Golgi and uncouples the assembly of the core structures of some glycans from later reactions, such as sialylation or sulfation. The drug can be used to examine if two pathways reside in the same compartment or share the same enzymes. Because the localization and array of the enzymes vary considerably in different cell types, extrapolating the effects of brefeldin A from one system to another is often difficult.
Some inhibitors act at key steps in intermediary metabolism where precursors involved in glycosylation are formed. For example, a glutamine analog, 6-diazo-5-oxo-L-norleucine, blocks glutamine:fructose-6-phosphate amidotransferase, the enzyme that forms glucosamine from fructose and glutamine (see Chapter 4). Depressing glucosamine production in this way has a pleiotropic effect on glycan assembly because all of the major families contain N-acetylglucosamine or N-acetylgalactosamine. Chlorate is another type of general inhibitor that blocks sulfation. The chlorate anion (ClO42−) is an analog of sulfate (SO42−) and it forms an abortive complex with the sulfurylase involved in the formation of phosphoadenosine-5′-phosphosulfate (PAPS), the active sulfate donor for all known sulfation reactions. Thus, treating cells with chlorate (usually 10–30 mM) inhibits sulfation by more than 90%, but the effect is not specific for any particular class of glycan or sulfation reaction (e.g., tyrosine sulfation is also affected).
A number of sugar analogs have been made with the hope that they might exhibit selective inhibition of glycosylation. 2-Deoxyglucose and fluorinated analogs of sugars (3-deoxy-3-fluoroglucosamine, 4-deoxy-4-fluoroglucosamine, 2-deoxy-2-fluoroglucose, and 2-deoxy-2-fluoromannose) inhibit glycoprotein biosynthesis as measured by decreased incorporation of radiolabeled glucosamine or fucose, but the mechanism underlying the inhibitory effect is unclear in many cases. Early studies of 2-deoxyglucose showed that the analog was converted to UDP-2-deoxyglucose as well as to GDP-2-deoxyglucose and dolichol-P-2-deoxyglucose. Inhibition of glycoprotein formation apparently occurs as a result of accumulation of various dolichol oligosaccharides containing 2-deoxyglucose, which cannot be elongated or transferred to glycoproteins normally. Care must be taken in interpreting the results of experiments employing these compounds because they may have effects due to metabolic conversion into other reactants.
TUNICAMYCIN: INHIBITION OF DOLICHOL-PP-GLCNAC ASSEMBLY
A number of natural products have been found to alter glycosylation. Tunicamycin belongs to a class of nucleoside antibiotics composed of uridine, an 11-carbon disaccharide called aminodeoxydialdose (tunicamine), and a fatty acid of variable length (13–17 carbons), branching, and unsaturation (Figure 50.1). Tunicamycin was first identified in Streptomyces lysosuperificus, and related compounds were found later in other microorganisms. It derives its name from its antiviral activity, which occurs by inhibiting viral coat (or “tunica”) formation.

FIGURE 50.1
Structure of tunicamycin, which consists of uridine conjugated to the disaccharide, tunicamine.
Tunicamycin inhibits N-glycosylation in eukaryotes by blocking the transfer of N-acetylglucosamine-1-phosphate (GlcNAc-1-P) from UDP-GlcNAc to dolichol-P (catalyzed by GlcNAc phosphotransferase; GPT), thereby decreasing the formation of dolichol-PP-GlcNAc (see Chapter 8). Other GlcNAc transferase reactions are not inhibited (e.g., GlcNAcTI–V), but the transfer of GlcNAc-1-P to undecaprenyl-P and the formation of undecaprenyl-PP-MurNAc pentapeptide (which is involved in bacterial peptidoglycan biosynthesis) are sensitive to tunicamycin (see Chapter 20). Tunicamycin acts as a tight-binding competitive inhibitor, presumably because it resembles the donor nucleotide sugar. The Ki value for tunicamycin is about 5 × 10−8 M, whereas the Km value for UDP-GlcNAc is approximately 3 × 10−6 M. The actual amount of tunicamycin needed to inhibit glycosylation varies in different cells (0.1–10 μg/ml), possibly because of variable uptake and culture conditions or differences in the level of expression of the phosphotransferase. Tunicamycin is cytotoxic to cells, and resistant mutants overproduce GPT. Similarly, transfection of cells with the cloned GPT confers resistance, suggesting that the variable dose of inhibitor required in different cells may reflect variation in enzyme levels.
Tunicamycin has been used extensively for studying the role of N-glycans in glycoprotein maturation, secretion, and function, as documented by thousands of papers citing its use since its first discovery in 1973. The drug was shown to induce apoptosis preferentially in cancer cells, presumably because of alterations in glycosylation of various cell-surface receptors and signaling molecules. Although the mechanism underlying its apoptosis-inducing activity may be complex, the observation suggests the possibility of a chemotherapeutic treatment based on inhibiting glycosylation. Inhibition of N-glycan formation could be useful for treating patients with naturally occurring mutations that create N-glycosylation sites in cell-surface receptors (gain-of-glycosylation mutants; see Chapter 42).
Another compound called amphomycin inhibits dolichol-P-mannose synthesis by forming a complex with dolichol-P. This compound is a lipopeptide and apparently forms complexes with the carrier lipid. Other lipophilic compounds have been described that bind lipid intermediates in bacterial cell wall synthesis (see Chapter 20).
PLANT ALKALOIDS: NATURAL INHIBITORS OF GLYCOSIDASES
Plant alkaloids block N-linked glycosylation by inhibiting the processing glycosidases involved in trimming nascent chains (Table 50.2). Unlike tunicamycin, which blocks glycosylation of glycoproteins entirely, the alkaloids inhibit the trimming reactions that occur after the Glc3Man9GlcNAc2 oligosaccharide is attached to a glycoprotein (see Chapter 8). Thus, the inhibitory alkaloids generally cause the appearance of glycoproteins on the cell surface lacking the characteristic termini found on mature N-glycans (see Chapter 13). One class of alkaloids inhibits the α-glucosidases involved in the initial processing of the N-glycans and in quality control of protein folding (see Chapters 29 and 36). This class includes castanospermine (from the seed of the Australian chestnut tree, Castanosperum australe), which inhibits both α-glucosidases I and II; australine (also from C. australe), which preferentially inhibits α-glucosidase I; and deoxynojirimycin (from Streptomyces species), which preferentially inhibits α-glucosidase II (Table 50.2). As expected, castanospermine and australine cause accumulation of fully glucosylated chains, whereas deoxynojirimycin results in chains containing one to two glucose residues. Treating cells with these inhibitors revealed that some trimming of the mannose residues could occur independently of removal of the glucose residues (see Chapter 8).
TABLE 50.2
Examples of alkaloids that inhibit glycosidases involved in N-linked glycan biosynthesis
Swainsonine was first discovered in plants from the western United States (Astragalus species; also known as locoweed) and Australia (Swainsona canescens), and it was later found in the fungus Rhizoctonia leguminocola that infects red clover. Consumption of these plants causes a severe abnormality called locoism and accumulation of glycoproteins in the lymph nodes. Thus, swainsonine is part of a chemical defense strategy used by plants against grazing animals (and probably insects). Swainsonine inhibits α-mannosidase II, causing the accumulation of high-mannose oligosaccharides (Man4GlcNAc2 and Man5GlcNAc2) and hybrid-type chains at the expense of complex oligosaccharides. It also inhibits the lysosomal α-mannosidase. Mannostatin A works in a similar way, but differs significantly in structure from swainsonine (Table 50.2). Other mannosidase inhibitors include deoxymannojirimycin and kifunensin, which selectively inhibit α-mannosidase I. As expected, these agents cause the accumulation of Man7–9GlcNAc2 oligosaccharides on glycoproteins.
All of these inhibitors have in common polyhydroxylated ring systems that mimic the orientation of hydroxyl groups in the natural substrates, but a strict correlation between stereochemistry and enzyme target (α-glucosidase vs. α-mannosidase) does not exist. The compounds contain nitrogen, usually in place of the ring oxygen. One idea is that the nitrogen in the protonated state may mimic the positive charge on the ring oxygen that arises from delocalization of charge from the tentative carbocation at C-1 generated during the hydrolysis reaction. Crystal structures for the α-mannosidase are available with bound inhibitors.
Alkylated and acylated analogs of the alkaloids have been made and shown to have interesting and useful properties. N-Butylation of deoxynojirimycin actually converts the glucosidase inhibitor into an inhibitor of glycolipid biosynthesis. Alkylation of the amino group or acylation of the hydroxyl groups can raise the potency of the compound, presumably by facilitating uptake across the plasma and Golgi membranes. Some of these compounds have shown positive effects as drugs for treating diabetes, lysosomal storage diseases, cancer, and HIV infection and for inducing male sterility (see Chapter 51).
INHIBITION OF O-GALNAC INITIATION OF MUCIN-TYPE GLYCANS
In contrast to the biosynthesis of N-linked glycans, comparatively few inhibitors are available that block O-linked glycans. Mucin-type O-linked glycan biosynthesis is initiated by polypeptidyl N-acetylgalactosaminyltransferases (ppGalNAcTs), a large family of enzymes that use UDP-GalNAc as a common donor and various glycoprotein acceptors (see Chapter 9). A synthetic library of uridine analogs was screened against members of the ppGalNAcT family, which led to the identification of two compounds that disrupt O-GalNAc addition (Figure 50.2). These compounds have Ki values of approximately 8μM with respect to UDP-GalNAc. Like tunicamycin, these inhibitors suppress glycosylation without selectivity for different glycoprotein targets. These compounds represent the first generation of enzyme inhibitors that work on O-linked glycans, and suggest the possibility of obtaining compounds that inhibit specific ppGalNAcT isoforms as well as other types of O-linked glycans, such as O-xylose (see Chapter 16), O-glucose (see Chapter 17), and O-GlcNAc (see Chapter 18).

FIGURE 50.2
Broad-spectrum inhibitors of the ppGalNAcTs identified from screening a uridine-based library.
INHIBITION OF O-GLCNAC MODIFICATION
With the realization of the importance of O-GlcNAc addition to many cytoplasmic and nuclear proteins (see Chapter 18), great interest exists in developing agents to inhibit its addition by O-GlcNAc transferase (OGT) or its removal by O-GlcNAc-specific β-hexosaminidase (O-GlcNAcase). Although alloxan and streptozotocin affect O-GlcNAc addition, these compounds lack specificity. The first potentially useful inhibitors of OGT have been obtained by screening chemical libraries for compounds that can displace a fluorescent derivative of the donor sugar, UDP-GlcNAc. The active compounds do not block other N-acetylglucosamine addition reactions, for example one involved in formation of the polysaccharide backbone of bacterial peptidoglycan (see Chapter 20).
Several inhibitors of the O-GlcNAcase have been devised based on the structure of N-acetylglucosamine. The first compound in this class, PUGNAc (O-[2-acetamido-2-deoxy-D-glucopyranosylidene]amino-N-phenylcarbamate; Figure 50.3) inhibits O-GlcNAcase at nanomolar concentrations, but also inhibits lysosomal β-hexosaminidases (HexA and HexB; see Chapter 41). N-Acetylglucosamine-thiazoline (NAG-thiazoline) is more specific and inhibits at even lower concentrations than PUGNAc. A new rationally designed glucoimidazole, GlcNAcstatin, inhibits O-GlcNAcase with a Ki of 4.6 pM and exhibits 105-fold selectivity over HexA and HexB. These compounds inhibit the enzyme in cultured cells, providing new tools to study the function of O-GlcNAc, and are potential candidates for drug therapy.

FIGURE 50.3
Inhibitors of O-GlcNAc-specific β-hexosaminidase (O-GlcNAcase).
SUBSTRATE ANALOGS: DIRECTED SYNTHESIS OF INHIBITORS
A number of inhibitors of specific transferases have been developed based on the concept that substrate analogs might act as tight-binding inhibitors. The general strategy is to modify the hydroxyl group that acts as the nucleophile during formation of the glycosidic bond or groups in its immediate vicinity (Table 50.3). About half of the compounds that have been made lack inhibitory activity, presumably because modification of the targeted hydroxyl group prevents binding of the analog to the enzyme by interfering with hydrogen bonding networks that hold the substrate in place. In other cases, the analogs exhibit Ki values in the approximate range of the Km values for the unmodified substrate. As one might expect, the analogs usually act competitively with respect to the unmodified substrate, but in a few cases the inhibition pattern is more complex, suggesting possible binding outside the active site.
TABLE 50.3
Synthetic substrate-based inhibitors of glycosyltransferases
Analogs of acceptors have the advantage that they should be selective for specific enzymes. However, analogs of nucleotide sugars provide opportunities for blocking classes of enzymes that utilize a common donor (e.g., all fucosyltransferases utilize GDP-fucose). A large number of nucleotide sugar derivatives have been made (e.g., N- and O-substituted analogs of UDP-GalNAc) and several exhibit inhibitory activity in vitro. “Bisubstrate” analogs consist of the nucleoside sugar donor or PAPS covalently linked to the acceptor substrate by way of a neutral bridging group. This arrangement has the potential for generating inhibitors whose binding characteristics reflect the product of the affinity constants for each substrate (approximated by the product of the individual Km values). Bisubstrates that have been made have Ki values in the range of the Km values for the nucleotide donors, suggesting that the correct geometry for the bridging group may have not been attained or that the analog binds in ways that differ from the natural substrates.
A certain amount of serendipity is needed to obtain active compounds using these synthetic approaches, and the synthesis of di-, tri-, and tetrasaccharides with the desired modifications is rather labor intensive. Nevertheless, the approach has yielded insights into the binding and reactivity of the enzymes, and substrate analogs with selectivity for particular enzymes have been developed in this way. Because many of the transferases have now been purified and cloned, we can look forward to more detailed kinetic and crystallographic studies, which will provide clues for deriving mechanism-based inhibitors in the future (see Chapter 5).
GLYCOSIDE PRIMERS: MIMICKING WHAT ALREADY WORKS
The utility of any glycosyltransferase inhibitor ultimately depends on its ability to cross the plasma membrane and enter the Golgi where the glycosyltransferases reside. Unfortunately, many of the compounds described above lack activity in live cells, presumably because their polarity and charge prevents their uptake. More than 35 years ago, Okayama and colleagues found that D-xylose in β-linkage to a hydrophobic aglycone (the noncarbohydrate portion of a glycoside; e.g., p-nitrophenol) was taken up rather efficiently and inhibited the assembly of glycosaminoglycans on proteoglycans. Xylosides mimic the natural substrate, xylosylated serine residues in proteoglycan core proteins, and thus act as a substrate. “Priming” of chains occurs on the added xyloside, which diverts the assembly process from the endogenous core proteins and causes inhibition of proteoglycan formation. In general, cells incubated with xylosides secrete large amounts of individual glycosaminoglycan chains and accumulate proteoglycans containing truncated chains. The enormous success of β-D-xylosides in altering proteoglycan biosynthesis suggested that other glycosides might act as “primers” as well (Table 50.4). Subsequently, studies have shown that β-N-acetylgalactosaminides prime oligosaccharides found on mucins and inhibit O-glycosylation of glycoproteins. Other active glycosides include β-glucosides, β-galactosides, β-N-acetylglucosaminides, and even disaccharides and trisaccharides. These compounds require conjugation to appropriate aglycones and acetylation to neutralize the polar hydroxyl groups on the sugars. Cells contain several carboxyesterases that remove the acetyl groups. Apparently, this can occur in a way that makes the compounds available to the transferases in the Golgi.
TABLE 50.4
Examples of glycoside primers
Priming by glycosides occurs in a concentration-dependent manner, but the efficiency varies widely among different compounds and cell types. These variations may relate to the relative abundance of endogenous substrates, enzyme concentration and composition, the solubility of different glycosides, their susceptibility to hydrolysis, their uptake across the plasma membrane and into the Golgi, and their relative affinity for the glycosyltransferases. The type of chain made on a given primer also depends on concentration and aglycone structure, which may reflect selective partitioning of primers into different intracellular compartments or into different branches of biosynthetic pathways. Like priming, inhibition of glycoprotein, glycolipid, or proteoglycan formation occurs in a dose-dependent fashion, but the blockade is rarely complete, probably because of the inability of glycosides to mimic completely the endogenous substrates.
Primers represent starting points for making analogs that might have the properties of tight-binding inhibitors described in the previous section. Many of the compounds described in Table 50.3 could be converted to permeable acylated glycosides and tested in live cells for inhibitory activity. Active compounds might have the potential to become carbohydrate-based drugs for treating diseases and disorders dependent on glycosylation. Priming of oligosaccharides may have beneficial effects as well. Xylosides, for example, can be absorbed through the gut, and when consumed at sufficient concentration, they exhibit antithrombotic activity. Many glycosides occur naturally, since various organisms (especially plants) produce hydrophobic compounds as part of chemical defense and conjugate them to sugars in order to render them soluble. Thus, the human diet may contain various types of glycosides with interesting (and unknown) biological activities.
MODULATING GLYCAN STRUCTURES USING ENZYMES AND MONOSACCHARIDES ANALOGS
A general assumption in glycobiology is that each glycan linkage is catalyzed by a highly selective transferase, with specificity both for substrate and donor nucleotide sugar. However, many transferases will catalyze formation of other linkages or utilize sugar analogs with low efficiency, but with sufficient capacity to alter composition under specific conditions. Some transferases will tolerate even large adducts appended to the donor nucleotide sugar. For example, one of the α1–3 fucosyltransferases will transfer a GDP-fucose analog containing bulky substituents at the C-6 position of the sugar (such as the H-blood group antigen). A mutant form of β1–4 galactosyltransferase has been described that can use UDP-2-acetonyl-2-deoxy-D-galactose as donor. Treatment of O-GlcNAc-containing glycoproteins with the nucleotide analog and the mutant enzyme introduces a ketone-tagged sugar that can then be reacted with a biotinylating reagent, thus allowing selection and detection of the modified glycoproteins. Microbial enzymes have also been engineered to tolerate unusual monosaccharides and nucleotide sugars, making it possible to synthesize glycoconjugates with unusually modified glycans.
Naturally occurring transferases will also tolerate modified sugars. For example, cells will metabolically incorporate mannosamine analogs with an acyl, alkyl, or ketone group covalently linked to the amino group instead of acetate (Figure 50.4). Thus, the various kinases and nucleotidyltransferases will apparently accept the analogs as substrates. When ketone-containing derivatives are incorporated into a cell, cell-surface glycoconjugates become sensitive to reagents that react with carbonyl groups. Other highly chemo-selective derivatives and reagents have been developed, including azides that can be modified selectively with phosphines (Staudinger ligation) or activated alkynes. The highly selective reactivity of these functionalities allows tagging of endogenous glycoproteins for proteomic analysis and imaging studies in vivo when injected systemically in mice. A number of endogenous pathways will use azide-containing monosaccharides, including pathways for incorporation of sialic acid (ManAz), GlcNAc (GlcNAz), and GalNAc (GalNAz) (see Figure 50.4). Metabolic glycan engineering might be used for the inventory of altered glycosylation in normal and diseased tissue or for modulating protein–glycan interactions.

FIGURE 50.4
Unnatural monosaccharides can be transformed into activated nucleotide sugars that are transported into the Golgi compartment and then transferred to glycoconjugates.
INHIBITORS OF GLYCOLIPIDS AND GPI ANCHORS
Reagents have been described that can alter the assembly of glycolipids in cells. Xylosides have a mild effect on glycolipid formation, possibly because of the similarity between α 2–3Galβ1–4Xylβ-xylose and glucose and the assembly of a GM3-like compound (Neu5Ac O-R) on the primer. Because cells will take up intermediates in glycolipid biosynthesis, they behave like synthetic glycoside primers. For example, glucosylceramide will give rise to complex glycolipids when fed to cells. On the basis of this observation, an analog containing a reactive exocyclic epoxide group was prepared. The compound inhibits glycolipid formation (IC50 ~ 8 μM), presumably by reaction of the epoxide with a nucleophile in the active site of lactosylceramide synthase.
As mentioned above, the α-glucosidase inhibitor, N-butyldeoxynojirimycin, has been used to inhibit glycosphingolipid formation because it blocks glucosylceramide synthesis. This compound is now in clinical use for treating type I Gaucher’s disease, a lysosomal storage disorder in which glucocerebrosidase is missing (see Chapter 41). Its beneficial activity occurs through “substrate deprivation” by blocking synthesis of glycosphingolipids, thereby “depriving” the lysosome of substrate. The sphingolipid analogs shown in Figure 50.5 are more potent inhibitors. Lengthening the hydrocarbon chain from 10 to 16 carbons further enhances efficacy. These compounds can almost completely deplete glycolipids in cells, and they require only micromolar amounts for activity.

FIGURE 50.5
Inhibitors of glycosphingolipid formation. These commercially available analogs are potent inhibitors of the glucosyltransferase that initiates glycosphingolipid formation.
Inhibitors of GPI anchor formation have also been described. Mannose analogs (2-deoxy-2-fluoro-D-glucose and 2-deoxy-D-glucose) inhibit the formation of dolichol-P-mannose in vivo and thus inhibit GPI biosynthesis, but they lack specificity because these agents also can affect other pathways dependent on dolichol-P-mannose. Mannosamine inhibits GPI anchor formation both in Trypanosoma brucei and in mammalian cells by formation of ManNH2-Man-GlcN-PI. Apparently, mannosamine in its activated form (GDP-ManNH2) is used as a substrate in the second mannosyltransferase reaction, but the ManNH2-Man-GlcN-PI intermediate will not act as a substrate for the next α2-mannosyl-transferase (see Chapter 11). GlcNR-phosphatidylinositols with different substituents (R) act as substrate analogs and some act as suicide inhibitors in vitro. Another class of inhibitors is based on fatty acid analogs that only trypanosomes incorporate into GPI anchors. Trypanosomes, unlike their mammalian hosts, incorporate myristic acid into GPI anchors by exchanging myristic acid for other fatty acids in the phosphatidylinositol moiety. By making a series of analogs, one was found that is highly toxic to trypanosomes in culture and nontoxic to mammalian cells (10-[propoxy]decanoic acid). Reagents like these have the potential to become drugs for treating trypanosomiasis, which is endemic in sub-Saharan regions of Africa.
RATIONAL DESIGN USING CRYSTAL STRUCTURES
Neuraminidase (Sialidase) Inhibitors
Studies of influenza neuraminidase exemplify the power of rationally designed drugs. The crystal structure for influenza neuraminidase was obtained in 1983, and since then many other enzymes have been characterized from other sources. Even before the crystal structure had been obtained, an inhibitor for neuraminidase was deduced by assuming that the hydrolysis reaction probably involved a transition state with a carbocation intermediate at C-2. This would result in C-2 and C-3 adopting a trigonal planar (sp2) configuration, and therefore compounds that mimicked this geometry were hoped to have inhibitory activity. Indeed, Neu5Ac-2-ene (DANA; Figure 50.6) has a micromolar Ki value. Interestingly, this compound works on most sialidases, but not on the trypanosome trans-sialidase and only weakly on bacterial sialidases.

FIGURE 50.6
Structure of influenza neuraminidase inhibitors. Chemical structure of Neu5Ac, NANA; 2-deoxy-2,3-dehydro-N-acetyl neuraminic acid, DANA; 4-amino-DANA; 4-guanidino-DANA (Relenza, zanamivir); (3R, 4R, 5S)-4-acetamido-5-amino-3-(1-ethylpropoxyl)-1-cyclohexane-1-carboxylic (more...)
Visual inspection of the influenza enzyme with the inhibitor bound showed that two glutamate residues lined a pocket near O4 of the sialic acid residue and formed hydrogen bonds with O4 (Figure 50.7). The pocket was fairly open, suggesting that a bulkier substituent at this position might be tolerated, at least sterically. A substrate analog was produced containing a positively charged guanidinium group instead of O4, and binding studies showed that the analog had a Ki value of 10−11 M (4-guanidino-DANA; Figure 50.6). The higher affinity was presumably due to an additional salt bridge formed between the charged guanidinium group and the carboxylates lining the pocket. Interestingly, the analog does not work on bacterial sialidases because the pocket is filled with an arginine group and only one aspartate residue lines the pocket.

FIGURE 50.7
Crystallographic structures of influenza virus neuraminidase (N9 subtype) with two different rationally designed inhibitors bound in the active site. The inhibitors are shown as colored ball and stick models, where yellow is carbon, blue is nitrogen, (more...)
Subsequent studies have attempted to use noncarbohydrate analogs based on the similarity of benzoic acid to the partially planar ring of the proposed intermediate in hydrolysis. Reasonably good inhibitors were found in this way, as long as hydrogen bond donors and acceptors were added to the ring (Figure 50.6). The best inhibitor contained a guanidinium group in the same position relative to the carboxylate anion (as in 4-guanido DANA), but the crystal data showed that the analog actually bound to the enzyme in an orientation very different from that of sialic acid. As the crystal structures for other sialidases are solved, the design of species-specific analogs may be possible. This rational approach for making inhibitors has great potential, not only for neuraminidase inhibitors, but also for the design of compounds that might block the activity of various glycosyltransferases.
Sulfotransferase Inhibitors
A large family of Golgi sulfotransferases installs sulfate esters on a variety of glycans using PAPS as the active sulfate donor. High-resolution crystal structures are now available for both glycan-modifying sulfotransferases as well as soluble drug detoxifying sulfotransferases. These enzymes have in common a conserved fold that binds PAPS (see Chapter 5). A series of small aromatic compounds (such as 2,6-dichloro-4-nitrophenol and pentachlorophenol) and several disaccharide analogs of GlcNAc-6-sulfotransferase substrates have inhibitory activity. Screening targeted libraries of purine derivatives yielded compounds with high selectivity towards individual sulfotransferases, suggesting that subtle differences in the PAPS-binding sites can be exploited.
The ability to screen large libraries against sulfotransferases has been facilitated by the development of high-throughput assays. Most low-throughput assays measure the transfer of [35S] from [35S]PAPS or measure the radiolabel transfer to a carbohydrate substrate bearing a hydrophobic tail that can easily be isolated using a reverse-phase cartridge. Some sulfotransferases will catalyze the reverse reaction in the presence of high concentrations of a sulfated donor. For example, β-arylsulfotransferase IV (β-AST-IV) will catalyze the reverse transfer of the sulfuryl group from p-nitrophenol sulfate to PAP, generating PAPS and p–nitrophenolate ion. When coupled to another sulfotransferase of interest, β-AST-IV regenerates PAPS and stoichiometric amounts of the ion, which can be monitored by UV absorbance. The enzyme also will transfer sulfate to water. A library of 35,000 compounds with purine and pyrimidine scaffolds was screened using β-AST-IV and a fluorescence assay that measured desulfation of 4-methylumbelliferone sulfate. Multiple hits were obtained with moderate inhibition, and subsequent structure elaboration of the library resulted in the generation of a very tight binding small molecule inhibitor with a Km value five orders of magnitude lower then the natural substrate. In theory, this approach can be exploited for other enzymes for which high-throughput assays can be developed.
FURTHER READING
- Tifft CJ, Proia RL. Stemming the tide: Glycosphingolipid synthesis inhibitors as therapy for storage diseases. Glycobiology. 2000;10:1249–1258. [PubMed: 11159916]
- Asano N. Glycosidase inhibitors: Update and perspectives on practical use. Glycobiology. 2003;13:93R–104R. [PubMed: 12851286]
- Brown JR, Fuster MM, Esko JD. Glycoside primers and inhibitors of glycosylation. In: Wong C-H, editor. Carbohydrate-based drug discovery. Vol. 2. 2003. pp. 883–889.
- Wiley-VCH, Weinheim. de Macedo CS, Shams-Eldin H, Smith TK, Schwarz RT, Azzouz N. Inhibitors of glycosylphosphatidylinositol anchor biosynthesis. Biochimie. 2003;85:465–472. [PubMed: 12770785]
- Chapman E, Best MD, Hanson SR, Wong CH. Sulfotransferases: Structure, mechanism, biological activity, inhibition, and synthetic utility. Angew Chem Int Ed Engl. 2004;43:3526–3548. [PubMed: 15293241]
- Garman E, Laver G. Controlling influenza by inhibiting the virus’s neuraminidase. Curr. Drug Targets. 2004;5:119–136. [PubMed: 15011946]
- Butters TD, Dwek RA, Platt FM. Imino sugar inhibitors for treating the lysosomal glycosphingolipidoses. Glycobiology. 2005;15:43R–52R. [PubMed: 15901676]
- Gross BJ, Kraybill BC, Walker S. Discovery of O-GlcNAc transferase inhibitors. J Am Chem Soc. 2005;127:14588–14589. [PubMed: 16231908]
- Hang HC, Bertozzi CR. The chemistry and biology of mucin-type O-linked glycosylation. Bioorg Med Chem. 2005;13:5021–5034. [PubMed: 16005634]
- Ostash B, Walker S. Bacterial transglycosylase inhibitors. Curr Opin Chem Biol. 2005;9:459–466. [PubMed: 16118062]
- Prescher JA, Bertozzi CR. Chemistry in living systems. Nat Chem Biol. 2005;1:13–21. [PubMed: 16407987]
- Blanchard S, Thorson JS. Enzymatic tools for engineering natural product glycosylation. Curr Opin Chem Biol. 2006;10:263–271. [PubMed: 16675288]
- Dorfmueller HC, Borodkin VS, Schimpl M, Shepherd SM, Shapiro NA, van Aalten DM. GlcNAcstatin: A picomolar, selective O-GlcNAcase inhibitor that modulates intracellular O-GlcNAcylation levels. J Am Chem Soc. 2006;128:16484–16485. [PMC free article: PMC7116141] [PubMed: 17177381]
- Zhang C, Griffith BR, Fu Q, Albermann C, Fu X, Lee IK, Li L, Thorson JS. Exploiting the reversibility of natural product glycosyltransferase-catalyzed reactions. Science. 2006;313:1291–1294. [PubMed: 16946071]
- Brown JR, Crawford BE, Esko JD. Glycan antagonists and inhibitors: A fount for drug discovery. Crit Rev Biochem Mol Biol. 2007;42:481–515. [PubMed: 18066955]
- Rexach JE, Clark PM, Hsieh-Wilson LC. Chemical approaches to understanding O-GlcNAc glycosylation in the brain. Nat Chem Biol. 2008;4:97–106. [PMC free article: PMC3250351] [PubMed: 18202679]
- ADVANTAGES OF INHIBITORS
- INDIRECT INHIBITORS AND METABOLIC POISONS
- TUNICAMYCIN: INHIBITION OF DOLICHOL-PP-GLCNAC ASSEMBLY
- PLANT ALKALOIDS: NATURAL INHIBITORS OF GLYCOSIDASES
- INHIBITION OF O-GALNAC INITIATION OF MUCIN-TYPE GLYCANS
- INHIBITION OF O-GLCNAC MODIFICATION
- SUBSTRATE ANALOGS: DIRECTED SYNTHESIS OF INHIBITORS
- GLYCOSIDE PRIMERS: MIMICKING WHAT ALREADY WORKS
- MODULATING GLYCAN STRUCTURES USING ENZYMES AND MONOSACCHARIDES ANALOGS
- INHIBITORS OF GLYCOLIPIDS AND GPI ANCHORS
- RATIONAL DESIGN USING CRYSTAL STRUCTURES
- FURTHER READING
- Review Chemical Tools for Inhibiting Glycosylation.[Essentials of Glycobiology. 2015]Review Chemical Tools for Inhibiting Glycosylation.Esko JD, Bertozzi C, Schnaar RL. Essentials of Glycobiology. 2015
- Review Chemical Tools for Inhibiting Glycosylation.[Essentials of Glycobiology. 2022]Review Chemical Tools for Inhibiting Glycosylation.Vocadlo DJ, Lowary TL, Bertozzi CR, Schnaar RL, Esko JD. Essentials of Glycobiology. 2022
- Introducing N-glycans into natural products through a chemoenzymatic approach.[Carbohydr Res. 2008]Introducing N-glycans into natural products through a chemoenzymatic approach.Huang W, Ochiai H, Zhang X, Wang LX. Carbohydr Res. 2008 Nov 24; 343(17):2903-13. Epub 2008 Sep 9.
- Review Neoglycorandomization and chemoenzymatic glycorandomization: two complementary tools for natural product diversification.[J Nat Prod. 2005]Review Neoglycorandomization and chemoenzymatic glycorandomization: two complementary tools for natural product diversification.Langenhan JM, Griffith BR, Thorson JS. J Nat Prod. 2005 Nov; 68(11):1696-711.
- Modeling and synthesis of novel tight-binding inhibitors of cytochrome P450 2C9.[Bioorg Med Chem. 2008]Modeling and synthesis of novel tight-binding inhibitors of cytochrome P450 2C9.Peng CC, Rushmore T, Crouch GJ, Jones JP. Bioorg Med Chem. 2008 Apr 1; 16(7):4064-74. Epub 2008 Jan 18.
- Chemical Tools for Inhibiting Glycosylation - Essentials of GlycobiologyChemical Tools for Inhibiting Glycosylation - Essentials of Glycobiology
Your browsing activity is empty.
Activity recording is turned off.
See more...