NCBI Bookshelf. A service of the National Library of Medicine, National Institutes of Health.
Varki A, Cummings RD, Esko JD, et al., editors. Essentials of Glycobiology. 2nd edition. Cold Spring Harbor (NY): Cold Spring Harbor Laboratory Press; 2009.
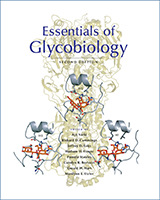
Essentials of Glycobiology. 2nd edition.
Show detailsMany microorganisms exploit host cell-surface glycans as receptors for cell attachment and tissue colonization, and a large number of pathogenic species depend on these interactions for infection. This chapter describes examples of proteins on the surface of microorganisms (adhesins or hemagglutinins), secreted proteins (heat-labile toxins), their glycan partners on mammalian cell surfaces (receptors), and insights into the molecular interactions that take place.
BACKGROUND
Viruses, bacteria, and protozoa express an enormous number of glycan-binding proteins or lectins. Many of these microbial lectins were originally detected based on their ability to aggregate red blood cells (i.e., to induce hemagglutination). The first microbial hemagglutinin identified was in the influenza virus, and it was shown by Alfred Gottschalk in the early 1950s to bind to erythrocytes and other cells though sialic acid residues of cell-surface glycoconjugates. Don Wiley and his associates crystallized the viral hemagglutinin and determined its structure in 1981, and later they solved the structure of cocrystals prepared with sialyllactose. Since then, a number of viral hemagglutinins have been identified and crystallized. These studies set the stage for analyzing other types of microbial lectins produced by bacteria and protozoa.
Nathan Sharon and colleagues first described bacterial surface lectins in the 1970s. These lectins also have hemagglutinating activity, but their primary function is to facilitate attachment or adherence of bacteria to host cells, a prerequisite for bacterial colonization and infection (see Chapter 39). Thus, bacterial lectins are often called adhesins, and the glycan ligands on the surface of the host cells are called receptors. Note that the term “receptor” in this case is equivalent to “ligand” for animal cell lectins. Bacteria also produce toxins, whose actions often depend on glycan-binding subunits that allow the toxin to combine with membrane glyco-conjugates and deliver the functionally active toxic subunit across the plasma membrane. During the last 30 years, many adhesins and toxins have been described, cloned, and characterized. Additionally, adhesins have been discovered on various parasites. The interactions of adhesins with glycan receptors can help determine the tropism of the organism.
Colonization of tissues by microorganisms is not always pathogenic. For example, the normal flora of the lower gastrointestinal tract is determined by appropriate and desirable colonization by beneficial bacteria. The initial events in the formation of nitrogen-fixing nodules in leguminous root tips by species of Rhizobium may also involve lectins on the root tip binding to Nod factors generated by the bacterium (see Chapter 37).
Many adhesins contain carbohydrate-recognition domains (CRDs) that bind to the same carbohydrates as endogenous mammalian lectins (see Chapter 26). Like animal cell lectins, some microbial adhesins bind to terminal sugar residues, whereas others bind to internal sequences found in linear or branched oligosaccharide chains. Detailed studies of the specificity of microbial lectins have led to the identification and synthesis of powerful inhibitors of adhesion that may form the basis for therapeutic agents for treating infection (see Chapter 51).
VIRAL GLYCAN-BINDING PROTEINS
By far, the best-studied example of a viral glycan-binding protein is the influenza virus hemagglutinin, which binds to sialic acid–containing glycans. The affinity of this interaction is relatively low, like that of other glycan-binding proteins with their glycan ligands, but the avidity for cell membranes increases because of oligomerization of the hemagglutinin into trimers and the high density of glycan receptors present on the host cell (see Chapter 27). Binding is a prerequisite for fusion of the viral envelope with the plasma membrane and for uptake of the virus into cells. The specificity of the interaction of the hemagglutinin with host glycans varies considerably for different subtypes of the virus. Human strains of influenza-A and -B viruses bind primarily to cells containing Neu5Acα2–6Gal-, whereas chicken influenza viruses bind to Neu5Acα2–3Gal- and porcine strains bind to both Neu5Acα2–3Gal- and Neu5Acα2–6Gal-containing receptors. This linkage preference is due to certain amino acid changes in the hemagglutinin. Influenza-C virus, in contrast, binds exclusively to glycoproteins and glycolipids containing 9-O-acetylated N-acetylneuraminic acid (see Chapter 39).
The specificity of the hemagglutinin correlates with the structures of sialylated glycans expressed on target epithelial cells in animal hosts. For example, trachea epithelial cells in humans express glycans with a preponderance of Neu5Acα2–6Gal linkages, whereas other tissues contain many more Neu5Acα2–3Gal-terminated glycans. Thus, the specificity of the hemagglutinin determines the tropism of the virus with respect to species and target cells. The hemagglutinin is also the major antigen against which neutralizing antibodies are produced, and antigenic changes in this protein are in part responsible for new viral outbreaks. In addition, it plays a critical role inside the cell where it facilitates pH-dependent fusion of the viral envelope with endosomal membranes after internalization.
The crystal structure of the hemagglutinin shows two disulfide-bonded subunits, HA1 and HA2, which are derived by cleavage of a precursor protein. The monomer consists of a hydrophilic carboxy-terminal domain located inside the viral envelope and a hydrophobic membrane-spanning domain. An elongated triple-helical coiled stem region extends 135 Å from the membrane topped by a globular domain that contains the carbohydrate-recognition domain (Figure 34.1). The crystal structure also revealed a hydrophobic pocket near the CRD, which explains why sialosides containing a hydrophobic aglycone bind the hemagglutinin with greater affinity than simple glycans.

FIGURE 34.1
Structure of the influenza virus hemagglutinin (HA) ectodomain. (a) A schematic diagram of the trimeric ectodomain of the H3 avian HA from A/duck/Ukr/63 showing residues HA1 9–326 and HA2 1–172. (Gray, red, blue) Modeled carbohydrate side (more...)
In addition to the hemagglutinin, influenza-A and -B virions express a sialidase (traditionally and incorrectly called neuraminidase) that cleaves sialic acids from glycoconjugates. Its functions may include (1) prevention of viral aggregation by removal of sialic acid residues from virion envelope glycoproteins, (2) dissociation of newly synthesized virions inside the cell or as they bud from the cell surface, and (3) desialylation of soluble mucins at sites of infection in order to improve access to membrane-bound sialic acids. In influenza-C virus, a single glycoprotein contains both the hemagglutinin activity and the receptor-destroying activity, which in this case is an esterase that cleaves the 9-O-acetyl group from acetylated sialic acid receptors. Powerful inhibitors have been designed based on the crystal structure of the sialidase from influenza-A virus. Some of these inhibit the enzyme activity at nanomolar concentrations and are in clinical use as antiviral agents (see Chapter 51).
Rotaviruses, the major killer of children worldwide, also can bind to sialic acid residues. These viruses only bind to the intestinal epithelium of newborn infants during a period that appears to correlate with the expression of specific types and arrangements of sialic acids on glycoproteins. Many other viruses (e.g., adenovirus, reovirus, Sendai virus, and polyomavirus) also appear to use sialic acids for infection, and crystal structures are now available for several of their sialic acid–binding domains (Table 34.1).
TABLE 34.1
Examples of viral lectins and hemagglutinins
A number of viruses use heparan sulfate proteoglycans as adhesion receptors (e.g., herpes simplex virus [HSV], foot-and-mouth disease virus, and dengue flavivirus [Table 34.1]). In many cases, the proteoglycans may be part of a coreceptor system in which the microorganisms make initial contact with a cell-surface proteoglycan and later with another receptor. For example, herpesvirus infection is thought to involve viral glycoproteins gC and/or gB binding to cell-surface heparan sulfate proteoglycans, followed by binding of viral glycoprotein gD to one of several cell-surface receptors, including heparan sulfate and one or more members of the Hve (herpesvirus entry) family of receptors. This step leads to fusion of the viral envelope with the host-cell plasma membrane. The coreceptor role of proteoglycan is reminiscent of the formation of ternary complexes required for antithrombin inhibition of thrombin and for fibroblast growth factor (FGF) cell signaling (see Chapter 35). The HSV glycoprotein gB binds heparan sulfate and promotes virus-cell fusion and syncytium formation (cell–cell fusion) as well as adherence. The mechanism by which heparan sulfate facilitates membrane fusion is unknown, but perhaps it acts like a template facilitating the association of fusogenic membrane proteins of the virus or host cell. Although it is clear that cell-surface proteoglycans act as adhesion receptors, their role in invasion and pathogenesis has not been established.
Dengue flavivirus, the causative agent of dengue hemorrhagic fever, binds to heparan sulfate. Modeling the primary sequence of the viral envelope protein on the crystal structure of a related virion envelope protein suggests that the heparan sulfate–binding site may lie along a groove in the protein lined by positively charged amino acids (Figure 34.2). Thus, the glycosaminoglycan (GAG)-binding adhesins may have a more open structure, consistent with the combining sites of other heparin-binding proteins (see Chapter 35). Foot-and-mouth disease viruses normally bind to epithelial cells through integrins, but after passage in cell culture, they can acquire the capacity to bind heparan sulfate. The combining site for heparin is located in a shallow depression formed by three of the major capsid proteins (Figure 34.3). HIV also can bind to heparan sulfate and other sulfated polysaccharides by way of the V3 loop of the gp120 glycoprotein. Thus, the heparan-sulfate-binding adhesins appear to pick out carbohydrate units within the polysaccharide chains as opposed to binding to terminal sugars. Interestingly, the interaction of the gD glycoprotein of Herpes simplex virus with heparan sulfate shows specificity for a particular substructure in heparan sulfate containing a 3-O-sulfated glucosamine residue, the formation of which is catalyzed by specific isozymes of the glucosaminyl 3-O-sulfotransferase gene family (see Chapter 16). However, it is unclear whether other viruses show a preference for specific heparan sulfate oligosaccharides. A growing body of literature suggests that heparan sulfate and heparin can block the interaction of the viruses with host cells, suggesting a simple therapeutic approach for treating viral infections.

FIGURE 34.2
Two views of a putative heparin-binding site in dengue virus envelope protein. The envelope protein monomer is shown in ribbon form, displayed along its longitudinal axis and as an external side view. (Top) Note the alignment of positively charged amino (more...)

FIGURE 34.3
Model of foot-and-mouth disease virus (FMDV) in complex with heparin trisaccharides. (a) A schematic depiction of the icosahedral capsid of FMDV. VP1–3 contribute to the external features of the capsid: (blue) VP1; (green) VP2; (red) VP3. The (more...)
BACTERIAL ADHESION TO GLYCANS
Bacterial lectins occur commonly in the form of elongated, submicroscopic, multisubunit protein appendages, known as fimbriae (hairs) or pili (threads), which interact with glycoprotein and glycolipid receptors on host cells. The best characterized of these are the mannose-specific type-1 fimbriae, the galabiose-specific P fimbriae, and the N-acetylglucosamine-binding F-17 fimbriae produced by different strains of Escherichia coli. Fimbriated bacteria express 100–400 of these appendages, which typically have a diameter of 5–7 nm and can extend hundreds of nanometers in length (Figure 34.4). Thus, pili extend well beyond the bacterial glycocalyx formed from lipopolysaccharide and capsular polysaccharides (see Chapter 20), which can actually interfere with their activity. The carbohydrate-recognition domain of the fimbriae is found in the minor subunit (FimH of type-1 fimbriae, PapG of P fimbriae, and F17G of F17 fimbriae) that is usually located at the tip of the fimbriae. Some of the other bacterial lectins are monomeric or oligomeric membrane proteins. Most bacteria (and possibly other microorganisms) have multiple adhesins with different carbohydrate specificities, which help define the range of susceptible tissues (i.e., the microbe’s ecological niche). Binding is generally of low affinity, but because the adhesins and the receptors often cluster in the plane of the membrane, the resulting avidity can be great. Perhaps an appropriate analogy for adhesin-receptor binding is the interaction of the two faces of Velcro™ strips.

FIGURE 34.4
E. coli express multiple pili as indicated by the fine filaments surrounding the cells. (Reprinted, with permission of Elsevier, from Sharon N. 2006. Biochim. Biophys. Acta 1760: 527–537; courtesy of David L. Hasty, University of Tennessee, Memphis, (more...)
Examination of the high-resolution, three-dimensional structure of the glycan-binding subunit FimH of type-1 fimbriae in complex with bound mannose revealed that although mannose exists in solution as a mixture of α and β anomers, only the former was found in the complex. It is buried at a deep and negatively charged site at the edge of FimH (Figure 34.5). All of the mannose hydroxyl groups, except the anomeric one, interact extensively with combining-site residues, almost all of which are situated at the ends of β-strands or in the loops extending from them. Part of the hydrogen-bonding network is identical to that found in mannose complexes of other lectins, such as those of plants.

FIGURE 34.5
α-Anomer of mannose in the combining site of FimH. The mannose is buried in a unique site at the tip of the receptor-binding domain (left) in a deep and negatively charged pocket (right). FimH selects the α configuration around the free (more...)
In the urinary tract, the type-1 fimbriae of E. coli mediate binding of the bacteria to a protein called uroplakin Ia. This glycoprotein presents high levels of terminally exposed mannose residues that are capable of specifically interacting with FimH. Anchorage of E. coli to the urothelial surface via type-1 fimbriae–uroplakin Ia interactions may play a role in their colonization of the bladder and eventual ascent through the ureters against urine flow to invade the kidneys. Another receptor for these fimbriae is the urinary Tamm–Horsfall glycoprotein, which acts as a soluble inhibitor of the adhesion of the bacteria to the uroplakins and helps to clear the bacteria from the urinary tract. Indeed, mice lacking the Tamm–Horsfall gene are considerably more susceptible to bladder colonization by type-1-fimbriated E. coli than normal mice, whereas they are equally susceptible to P-fimbriated E. coli that do not bind to the same glycoprotein. Type-1 fimbriae also are instrumental in the attachment of E. coli to human polymorphonuclear cells and to human and mouse macrophages. This is often followed by the ingestion and killing of the bacteria, a phenomenon named lectinophagocytosis, which is an early example of innate immunity (see Chapter 39).
Other binding specificities have been described as well (Table 34.2). The specificity of binding can explain the tissue tropism of the organism. The columnar epithelium that lines the large intestine expresses Galα1–4Gal-Cer, whereas the cells lining the small intestine do not. Thus, Bacterioides, Clostridium, E. coli, and Lactobacillus only colonize the large intestine under normal conditions. P-fimbriated E. coli as well as different toxins bind specifically to galabiose (Galα1–4Gal) and galabiose-containing oligosaccharides, most commonly as constituents of glycolipids. Binding occurs either to terminal nonreducing galabiose units or to internal ones (i.e., when the disaccharide is capped by other sugars). P-fimbriated E. coli adhere mainly to the upper part of the kidney, where galabiose is abundant. E. coli K99 provides a striking illustration of the fine specificity of bacterial surface lectins and their relationship to the animal tropism of the bacteria. This organism binds to glycolipids that contain N-glycolylneuraminic acid (Neu5Gc), in the form of Neu5Gcα2–3Galβ1–4Glc, but not to those that contain N-acetylneuraminic acid. These two sugars differ in only a single hydroxyl group, present in the acyl substituent on the amino group at C-5 of N-glycolylneuraminic acid and absent in that of N-acetylneuraminic acid. N-glycolylneuraminic acid is found on intestinal cells of newborn piglets, but it disappears when the animals develop and grow, and it is not formed normally by humans. This can explain why E. coli K99 can cause often lethal diarrhea in piglets but not in adult pigs or humans.
TABLE 34.2
Examples of interactions of bacterial adhesins with glycans
The relationship between microbes and the host can be quite complex. For example, colonization of germ-free mice with Bacteroides thetaiotaomicron, a normal resident microbe of the small intestine, can induce an α1–2 fucosyltransferase in the mucosal epithelial cells. The bacteria bind to L-fucose residues and also use it as a carbon source.
TOXINS THAT BIND GLYCANS
A number of secreted bacterial toxins also bind to glycans (Table 34.3). The best-studied example is the toxin from Vibrio cholera (cholera toxin), which consists of A and B sub-units in the ratio AB5. Its crystal structure shows that the B subunits bind to the Galβ1–3GalNAc moiety of GM1 ganglioside receptors through CRDs located on the base of the subunits (Figure 34.6). The A subunit is loosely held above the plane of the B subunits, with a single α-helix penetrating through a central core created by the pentameric B subunits. Upon binding to membrane glycolipids through the B subunits, the A subunit is delivered to the interior of the cell by an unknown mechanism. In cholera toxin, the affinity of the B subunit for its glycan ligand (GM1) is unusually high compared to the binding of related AB5 toxins from Shigella dysenteria, Bordetella pertussis, and E. coli to their glycan receptors (Kd in the nanomolar range for cholera toxin B subunit vs. millimolar range for Shiga toxin B subunit binding to Pk determinants). In both examples, the formation of the pentameric complex greatly increases the avidity of the interaction. This phenomenon is being exploited to make oligovalent glycan ligands as protective agents against the toxin.
TABLE 34.3
Examples of receptors for bacterial toxins

FIGURE 34.6
Crystal structure of cholera toxin B-subunit pentamer with bound GM1 pentasaccharide shown from the bottom (a) and from the side (b). (Redrawn, with permission, from Merritt E.A., Sarfaty S., van den Akker F., L’Hoir C., Martial J.A., and Hol (more...)
Bacillus thuringiensis, which lives in the soil, produces crystal toxins (Bt toxins) that can kill larval stages of plant-pathogenic insects, but they are harmless to most other organisms, including humans. Bt toxins are used in crop protection by spraying plants or by genetically engineering crops to express the toxins. Recent work demonstrates that Bt toxins act by binding to glycolipids that line the gut in nematodes and presumably other invertebrates (see Chapter 23). These receptors belong to the arthroseries of glycolipids and include in their structure the characteristic ceramide-linked, mannose-containing core tetrasaccharide GalNAcβ1–4GlcNAcβ1–3Manβ1–4GlcβCer, which is found only in invertebrates and is conserved between nematodes and insects but is absent in vertebrates (see Chapter 24). Thus, the specificity of Bt toxins determines their tissue and species sensitivity.
Shiga toxin produced by Shigella dysenteria and the homologous Shiga-like toxins of E. coli (also called verotoxins) will bind to Galα1–4Gal determinants on both glycolipids and glycoproteins, but only the binding to glycolipids results in cell death. The apparent preference of most toxins for glycolipids may be related to the juxtaposition of glycolipid glycans to the membrane surface, compared to the more distal location of glycans attached to glycoproteins and proteoglycans. Binding of a toxin or bacterium to a glycolipid also might increase the likelihood of further interactions with the membrane (e.g., binding to another receptor or membrane intercalation).
PARASITE LECTINS
In addition to viruses and bacteria, a number of parasites also utilize glycans as receptors for adhesion (Table 34.4). Entamoeba histolytica expresses a 260-kD heterodimeric lectin that binds to terminal galactose/N-acetylgalactosamine residues on glycoproteins and glycolipids. Binding may have a role in attachment, invasion, and cytolysis of intestinal epithelium, and it may function in binding the amoeba to bacteria as a food source. The lectin is heterodimeric, with a transmembrane subunit of 170 kD and a glycosylphosphatidylinositol (GPI)-anchored subunit of 35 kD. The glycan-binding site is located in a cysteine-rich domain. The importance of this receptor in virulence has been established by antisense silencing of the adhesin and by expression of a dominant-negative form of the light subunit. Thus, the adhesin is a potential target to manage E. histolytica infection (see Chapter 40).
TABLE 34.4
Examples of glycan receptors for parasites
The interaction of Plasmodium falciparum (malaria) merozoites with red blood cells depends on sialic acids present on the host cell, in particular on the major erythrocyte membrane protein glycophorin. In this organism, attachment is mediated by a family of specific sialic acid–binding adhesin on merozoites, the most prominent of which is called EBA-175 (erythrocyte-binding antigen-175). The adhesin shows specificity for the type of sialic acid, with preference for Neu5Ac, rather than for 9-O-acetyl-Neu5Ac or Neu5Gc. Soluble Neu5Ac and Neu5Acα2–6Gal-containing oligosaccharides do not competitively inhibit the binding of EBA-175 to erythrocytes, but Neu5Acα2–3Gal-containing oligosaccharides are effective inhibitors, indicating that the adhesin is sensitive to the linkage of the sialic acid to the underlying galactose. Binding to erythrocytes leads to invasion and eventual production of additional merozoites. Other organisms expressing sialic acid adhesins can also bind to erythrocytes (e.g., influenza virus), but these interactions do not lead to productive infections in these nonnucleated cells. Thus, under these circumstances, the erythrocyte might be considered to be a clearance mechanism for these agents (see Chapter 3).
Plasmodium-infected erythrocytes also express GAG-binding proteins that are thought to facilitate adherence of infected cells to tissues. The circumsporozoite form of P. falciparum binds to heparan sulfate in a tissue-specific manner, with preferred binding to the basal surface of hepatocytes and the basement membrane of kidney tubules. The carboxyl terminus of the protein contains positively charged residues. Clustering of the circumsporozoite protein on the surface of the organism may generate a high concentration of positively charged residues that facilitate binding. Recent studies show that the extent of sulfation of heparan sulfate that the parasite encounters determines whether it migrates or productively invades host cells.
MICROBIAL GLYCAN LIGANDS FOR ANIMAL CELL LECTINS
Like mammalian cells, bacteria, viruses, and parasites are covered by glycans. The enveloped viruses contain virally encoded membrane glycoproteins and may pick up host glycoproteins and glycolipids during the budding process. Bacteria contain a cell wall composed of peptidoglycan, teichoic acids (Gram-positive organisms), and lipopolysaccharide (Gram-negative organisms) and also, in some species, a capsule (see Chapter 20). All of these glycans can potentially interact with host-cell lectins. Thus, it is not surprising that interactions between microbial glycans and host lectins can also aid in infection and colonization.
Trypanosoma cruzi has developed an interesting strategy of molecular camouflage in which a parasite-encoded trans-sialidase transfers sialic acid from serum glycoproteins in the host to membrane proteins on its own surface. The primary function of this reaction is most likely to cover surface glycans as a way of preventing host immune reactivity, although the trans-sialidase may also act as an adhesin. Neisseria gonorrhoeae uses low levels of tissue CMP-sialic acid to cover itself with sialic acid residues, making it resistant to the alternative pathway of complement. It also appears likely that these and other sialylated pathogens interact with host via the Siglec family of sialic acid–binding lectins (see Chapter 32). Schistosomes, which are parasitic filarial worms, contain the Lewisx antigen that is also found on human leukocytes. Because Lewisx is recognized by selectins, the presence of these carbohydrates may provide a mechanism for attachment or transcellular migration of the parasite. However, these glycans also generate a massive anti-Lewisx antibody response in the host.
In a similar way, the capsules and lipopolysaccharide that surround bacteria, as well as yeast cell walls, contain glycans that may be recognized by mammalian cell lectins. For example, yeast mannans are recognized by both soluble and macrophage mannose-binding proteins, which have an important role in innate immune defense during the preimmune phase in infants. Highly virulent forms of pathogenic bacteria such as Streptococcus contain a hyaluronan capsule, which may interact with hyaluronan-binding proteins (such as CD44) present on host-cell surfaces and facilitate colonization. The structure and biology of these types of glycans and glycan-binding proteins are discussed in Chapters 20, 21, and 40.
ROLE IN INFECTIOUS DISEASE
As pointed out earlier, the major function of the microbial lectins is to mediate adhesion of the organisms to host cells or tissues, which is a prerequisite for infection to occur. This has been extensively demonstrated both in vitro (in studies with isolated cells and cell cultures) and in vivo (in experimental animals) and is supported in some cases by clinical data. For example, lectin-deficient microbial mutants often lack the ability to initiate infection. In addition, type-1 fimbrial expression is associated with the severity of urinary tract infection in children. Moreover, mono- or oligosaccharides have been shown to protect against infection by lectin-carrying bacteria in experimental models.
Glycans recognized by microbial surface lectins block the adhesion of the organisms to animal cells not only in vitro, but also in vivo, and thus protect animals against infection by such organisms. For example, coadministration of methyl α-mannoside with type-1-fimbriated E. coli into the urinary bladder of mice reduces significantly the rate of urinary tract infection, whereas methyl α-glucoside, which is not inhibitory to the fimbriae, has no effect. The protective effect of antiadhesive sugars has been demonstrated in a variety of studies with different pathogenic bacteria and animals, from rabbits to monkeys. Multivalent ligands should prove even more potent.
The ability of exogenous heparin and related polysaccharides to inhibit viral replication suggests that this approach might lead to polysaccharide-based antiviral pharmaceutical agents. As more crystal structures become available, the ability to custom design small-molecule inhibitors that fit into the carbohydrate-recognition domains of adhesins should improve. Already, the structures of influenza hemagglutinin and sialidase have suggested numerous ways to modify sialic acid to fit better into the active sites. Some of these compounds are presently in use to limit the spread of virus (see Chapters 50 and 51).
FURTHER READING
- Rostand KS, Esko JD. Microbial adherence to and invasion through proteoglycans. Infect Immun. 1997;65:1–8. [PMC free article: PMC174549] [PubMed: 8975885]
- Kitov PI, Sadowska JM, Mulvey G, Armstrong GD, Ling H, et al. Shiga-like toxins are neutralized by tailored multivalent carbohydrate ligands. Nature. 2000;403:669–672. [PubMed: 10688205]
- Williams SJ, Davies GJ. Protein–carbohydrate interactions: Learning lessons from nature. Trends Biotechnol. 2001;19:356–362. [PubMed: 11513999]
- Hooper LV, Midtvedt T, Gordon JI. How host-microbial interactions shape the nutrient environment of the mammalian intestine. Annu Rev Nutr. 2002;22:283–307. [PubMed: 12055347]
- Sharon N, Lis H. History of lectins: From hemagglutinins to biological recognition molecules. Glycobiology. 2004;14:53R–62R. [PubMed: 15229195]
- Spear PG. Herpes simplex virus: Receptors and ligands for cell entry. Cell Microbiol. 2004;6:401–410. [PubMed: 15056211]
- Griffitts JS, Aroian RV. Many roads to resistance: How invertebrates adapt to Bt toxins. BioEssays. 2005;27:614–624. [PubMed: 15892110]
- Newburg DS, Ruiz-Palacios GM, Morrow AL. Human milk glycans protect infants against enteric pathogens. Annu Rev Nutr. 2005;25:37–58. [PubMed: 16011458]
- Olofsson S, Bergstrom T. Glycoconjugate glycans as viral receptors. Ann Med. 2005;37:154–172. [PubMed: 16019714]
- Sharon N. Carbohydrates as future anti-adhesion drugs for infectious diseases. Biochim. Biophys. Acta. 2006;1760:527–537. [PubMed: 16564136]
- Sinnis P, Coppi A. A long and winding road: The Plasmodium sporozoite’s journey in the mammalian host. Parasitol Int. 2007;56:171–178. [PMC free article: PMC1995443] [PubMed: 17513164]
- Mandlik A, Swierczynski A, Das A, Ton-That H. Pili in Gram-positive bacteria: Assembly, involvement in colonization and biofilm development. Trends Microbiol. 2008;16:33–40. [PMC free article: PMC2841691] [PubMed: 18083568]
- Review Microbial Lectins: Hemagglutinins, Adhesins, and Toxins.[Essentials of Glycobiology. 2015]Review Microbial Lectins: Hemagglutinins, Adhesins, and Toxins.Nizet V, Varki A, Aebi M. Essentials of Glycobiology. 2015
- Review Microbial Lectins: Hemagglutinins, Adhesins, and Toxins.[Essentials of Glycobiology. 2022]Review Microbial Lectins: Hemagglutinins, Adhesins, and Toxins.Lewis AL, Kohler JJ, Aebi M. Essentials of Glycobiology. 2022
- Carbohydrate receptor mimicry as a basis for antibacterial therapy.[Curr Opin Drug Discov Devel. 2...]Carbohydrate receptor mimicry as a basis for antibacterial therapy.Armstrong GD. Curr Opin Drug Discov Devel. 2000 Mar; 3(2):191-202.
- Review Microbial cell surface proteins and secreted metabolites involved in multispecies biofilms.[Pathog Dis. 2014]Review Microbial cell surface proteins and secreted metabolites involved in multispecies biofilms.Demuyser L, Jabra-Rizk MA, Van Dijck P. Pathog Dis. 2014 Apr; 70(3):219-30. Epub 2014 Jan 27.
- Review Bacterial adhesion to oral tissues: a model for infectious diseases.[J Dent Res. 1989]Review Bacterial adhesion to oral tissues: a model for infectious diseases.Gibbons RJ. J Dent Res. 1989 May; 68(5):750-60.
- Microbial Lectins: Hemagglutinins, Adhesins, and Toxins - Essentials of Glycobio...Microbial Lectins: Hemagglutinins, Adhesins, and Toxins - Essentials of Glycobiology
Your browsing activity is empty.
Activity recording is turned off.
See more...