NCBI Bookshelf. A service of the National Library of Medicine, National Institutes of Health.
Varki A, Cummings R, Esko J, et al., editors. Essentials of Glycobiology. Cold Spring Harbor (NY): Cold Spring Harbor Laboratory Press; 1999.
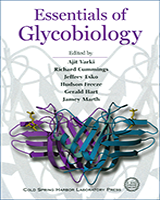
Essentials of Glycobiology.
Show detailsPrimary contributions to this chapter were made by H.H. Freeze (The Burnham Institute, La Jolla, California).
EACH MAJOR FORM OF GLYCOSYLATION in eukaryotic cells is defined by the linkage between the first sugar and the aglycone, the noncarbohydrate moiety, most of which are synthesized in the ER and Golgi. This chapter explores examples of unusual glycosylation events in the ER/Golgi pathway, the systems in which they occur, specific proteins modified, glycosyltransferases involved, and a few proposed functions. Some of these modifications have only been seen in lower eukaryotes, others only in higher eukaryotes, and still others span the evolutionary spectrum.
Two types of single sugar modifications are not covered here. One is the addition of sugars such as glucuronic acid to bile acids and xenobiotics. Their function is to increase the water solubility of the conjugates. The other type is called glycation (not be confused with glycosylation). This is the nonenzymatic addition of sugars to proteins in which the aldehyde group of a sugar such as glucose reacts with free amino groups on proteins.
O-linked Modifications
The term O-linked glycosylation is typically assumed to represent the common addition of GalNAc to serine or threonine residues (see Chapter 8). However, there are many other forms of O-glycosylation (for a summary, see Table 12.1). Since O-(β)GlcNAc is the established term for GlcNAc bound to serine and threonine hydroxyl groups in cytosolic and nuclear proteins, the same nomenclature is adopted for the O-linked modifications described in this chapter.
Table 12.1
Less common types of glycosylation in the Golgi.
Proteins with EGF-like Modules Can Have Glc β-O-Ser and Fuc α-O-Ser/Thr(1)
A select group of mammalian proteins with EGF-like modules is modified by two rare forms of glycosylation. The proteins include the mammalian version of the widely distributed notch-1 and several proteins involved in the coagulation cascade.
O-(α)Fucose(2–4)
The O-fucosylation pathway is defined by the linkage Fucα-Ser/Thr. The first evidence suggesting the existence of this type of pathway was the identification of a glycopeptide, Glcβ1–3Fucα-Thr, in human urine. This discovery generated little interest at the time, but interest increased when O-fucose was identified in human urokinase. This led to its discovery in other clotting proteins (Table 12.1) such as tissue plasminogen activator (t-PA) and clotting factor VII. All of the O-fucosylated proteins identified so far have an EGF-like module and glycosylation occurs at a similar serine or threonine in each one, as shown in Figure 12.1. In factor VII, the glycan is extended with three additional sugars to form Siaα2–6Galβ1–4GlcNAcβ1–3Fucα-. This sialyl-lactosamine triad is the familiar signature of many serum glycoproteins with N-glycans (see Chapter 7). Addition of sialic acid and galactose may use sialyl and galactosyltransferases employed by other pathways, but formation of the GlcNAcβ1–3Fuc bond probably requires a transferase unique to this pathway. Until recently, fucose was usually considered to be a terminal sugar, so there was no inclination to search for glycosyltransferases that use fucose as an acceptor. As more structures are identified, some of the old “rules” change. For example, N-glycans in octopus rhodopsin have a nonterminal fucose (Galβ1–4 Fucα1–6GlcNAc) in the core region, and SKP1 in Dictyostelium has a nonterminal fucose in a hydroxyproline-linked oligosaccharide synthesized in the cytosol (see Chapter 13).

Figure 12.1
Consensus sequences for Xyl-Glc and O-Fuc addition in EGF-like modules.
O-(α)Fuc is also found on glycoproteins made by CHO cells. This cell line is a favorite with glycobiologists because it can be used for making glycosylation-defective mutants (see Chapter 31). A subset of the O-fucosylated glycoproteins in CHO cells also carries the Glcβ1–3 extension similar to the original glycopeptide found in human urine. Again, forming this disaccharide structure probably involves a specific β-glucosyltransferase. The CHO proteins have not been identified, and thus it is not known if they have an EGF-like module. Small synthetic peptides that resemble parts of the EGF-like module are poor acceptors for in vitro O-fucosyl transferase assays, which may mean that all the proper disulfide bonds of the EGF-like module are required or that other aspects of the protein contribute to the specificity.
The function of O-Fuc is not known, but a fucose lectin-type receptor on the hepatocyte surface was suggested to be involved in clearance of t-PA from the circulation. Fucose and fucosyl-albumin conjugates inhibited binding, and α-fucosidase digestion of t-PA reduced binding. Lectin-type receptors are known to control the half-life of other circulatory glycoproteins, as discussed in Chapter 22, but the influence of fucose on the half-life of t-PA has not yet been determined. The human mannose receptor (see Chapter 25) has a much higher affinity for t-PA than other proteins containing high-mannose-type sugar chains. Could O-(α)Fuc have an enhancing role in mediating t-PA clearance? Probably not, since mutating the O-(α)Fuc attachment site has no effect on its binding to the mannose receptor. The addition of other sugars to O-(α)Fuc on other proteins suggests that those sugars could have other functions. One contribution could be in cell fate determination through the widely distributed protein, notch. Mammalian notch-1 has a series of 36 tandem EGF-like modules, and 12 of them have the consensus sequence for O-fucosylation. When expressed in CHO cells, this molecule is modified with mono- and oligosaccharide versions of O-(α)Fuc.
O-fucosylation has been preserved through phylogeny, since it seems to occur on selected proteins expressed during development of the primitive eukaryote, Dictyostelium discoideum. Only a few proteins are modified, but they also occur in EGF-like modules with mucin-like domains and form part of the coat surrounding spores made during multicellular development of this organism (see Chapter 19).
O-(β)Glucose(5)
An O-(β)-linked glucose residue defines this pathway. Factors VII, IX, protein Z, and thrombospondin from human or bovine sources (Figure 12.1) have Xylα1–3+/- Xylα1–3Glcβ-O- at a similar serine residue in their EGF-like modules. The human notch-1 protein also has O-glucosylation. The consensus site for addition occurs only nine amino acids away from the O-fucosylation site of these proteins. Glucosylation occurs on all molecules, but the addition of both xylose residues occurs in only a portion of the molecules. Each of the xylose residues is added sequentially by a separate xylosyltransferase. No model peptides have been used to study acceptor specificity. Recombinant factor VII without the glycosylation site has only slightly reduced catalytic activity. The functional significance of this unusual modification remains unknown, but its identification in notch-1 offers a new avenue of investigation.
Glcα1–2Galβ-O-Hydroxylysine(6–8)
This pathway was first recognized in the 1960s as acting on collagen and has since been extended to a few other proteins that have collagen-like modules. The first step in the pathway is the obligate hydroxylation of lysine to hydroxylysine. Once the acceptor sites are created, the two-step glycosylation rapidly follows on most of the available acceptor sites. Several other proteins with conserved collagen-like domains that form triple-helical structures also carry the disaccharide, including pulmonary surfactant proteins, complement factor C1q, and rat and human mannan-binding proteins made by the liver. The mannan- binding protein found in the serum assembles into large aggregates by interchain disulfide bonds that depend on the creation of hydroxylysine, and presumably on glycosylation. Triple-helix formation of collagen can also prevent the addition of galactose to the glucosylated form, perhaps by sterically blocking the saccharide residue.
Primitive forms of collagen in sponges and sea anemone also have disaccharide-modified hydroxylysine, so these modifications have probably partnered with collagen for a long time. These glycosylations are thought to be involved in mediating, or in some cases preventing, aggregations of the triple helix in these molecules.
O-Mannose (9–11)
O-αMan was identified in yeast in the 1950s and is discussed in more detail in Chapter 19. Briefly, mannose is α-linked to serine/threonine and may be elongated by several additional mannose residues using a battery of mannosyltransferases that extend similar structures on N-linked chains in yeast.
Mannose was first identified as the protein-linked sugar in a rat brain proteoglycan in 1979. Because it was released by mild base hydrolysis with simultaneous reduction of mannose to mannitol, the anomeric linkage could not be determined. Gal(β1–4)[Fucα1–3]GlcNAcβ1–2Man, GlcNAcβ1–2Man, and mannose by itself were found (Figure 12.2). More recently, O-mannose-based glycans containing four to eight sugars have been found in total brain glycopeptides and a tetrasaccharide derived from α-dystroglycan. This protein is found on Schwann cell membranes and links another membrane protein, β-dystroglycan, to laminin in the extracellular matrix. The oligosaccharide Sia2–3Galβ1–4GlcNAc-β1–2Man-Ser/Thr accounts for two thirds of the O-glycans of α-dystroglycan and is probably involved in the important α-dystroglycan-laminin binding, since sialidase prevents the interaction, but removal of N-glycans does not. In addition, dystroglycan isolated from sheep brain has been shown to contain a fucosylated, nonsialylated O-mannose-based structure with the Galβ1–4GlcNAcβ1–2Man linkage. This Lewis X (Lex) determinant is well known on N-glycans and on more typical GalNAc(α)-O-glycans. Some of the structures are shown in Figure 12.2. The activated mannose donor used to initiate this pathway is unknown. The O-mannose biosynthetic pathway may be more diverse and extensive than expected and may prove to be important for specific cell adhesion processes.

Figure 12.2
O-mannose-based oligosaccharides.
A small portion of total rat brain glycopeptides has O-mannose-linked glycans and carries the HNK-1 antigenic epitope, an unusual 3-O-sulfated glucuronic acid found at the nonreducing end of the chain. This epitope is implicated in neuronal cell adhesion. Some of the O-mannose-based chains with HNK-1 antigen are substituted with a second GlcNAc creating a branched structure. These GlcNAc transferases are probably unique to the O-mannose pathway and different from those used for the addition of GlcNAc to N-linked chains.
The key to finding these O-mannose-linked chains was the HNK-1-specific antibody, since it recognizes the nonreducing end of a chain, but it is indifferent to the sugar found at the reducing terminus. Selective recognition of a portion of the sugar chain is often found with both antibodies and glycosyltransferases. Other proteins besides α-dystroglycan that carry these interesting O-glycans have not been identified, but they must be abundant since the estimated ratio of mannose-terminated to GalNAc-terminated O-glycans in total brain glycopeptides is approximately 1:3 (A.M. Lawson and T. Feizi, pers. comm.).
O-αGlcNAc
Chapter 14 describes a broad range of functions of nuclear and cytoplasmic proteins modified by O-(β)GlcNAc. However, O-(α)GlcNAc can be found in some proteins traversing the ER/Golgi pathway. So far, it has only been recognized in a group of glycoproteins found in D. discoideum. It was initially defined by a monoclonal antibody reactivity and later the structure was solved. α-GlcNAc is added within a series of multiple mucin-like PTVT peptide repeats. Mutants lacking this Golgi-added O-αGlcNAc have impaired multicellular development because an adhesion protein called contact site A or gp80 is more sensitive to proteolysis when it lacks this modification. The adhesion molecule has multiple mucin-like domain repeats, and the cluster of α-GlcNAc residues is thought to cause this region to form a rod-like structure. This conformation is not maintained without glycosylation, and a cell surface protease is able to cleave it.
Phosphoglycosylation in Lower Eukaryotes (12)
Dictyostelium adds GlcNAcα-1-P and Fucβ-1-P directly to serine residues of selected proteins. Leishmania adds Manα-1-P, which is sometimes extended with more sugars. None of these modifications have yet been found in other organisms.
GlcNAcα-1-P-Ser and Fucβ-1-P-Ser inDictyostelium(13–16)
Dictyostelium is a unicellular amoeba that can grow on bacteria or in synthetic growth media. When the food source is exhausted, up to 100,000 amoeba aggregate to form a multicellular organism (see Chapter 19). During vegetative growth, GlcNAcα-1-P is directly added from UDP-GlcNAc to serine residues of a select group of lysosomal proteins. Several papain-like cysteine proteinases are the major carriers of this modification. Although cysteine proteinases are highly conserved enzymes throughout plants and animals, the Dictyostelium enzymes have additional serine-rich domains containing 20–110 amino acids where GlcNAc-1-P is added. Small peptides that resemble the serine motifs from these enzymes are good artificial acceptors in in vitro assays, but the native conformation of the cysteine proteinases imparts an added level of recognition specificity. In fact, mammalian cysteine proteinases that lack the serine domain and cannot be phosphoglycosylated still bind to GlcNAcα-1-P transferase and can act as inhibitors. This probably means that GlcNAcα-1-P transferase recognizes a conformation-dependent structural feature on the proteinase as well as the local glycosylation site, analogous to that seen with the GlcNAcα-1-P transferase responsible for the first step in the addition of Man-6-P to mammalian lysosomal enzymes (see Chapter 23).
The function of GlcNAcα-1-P-Ser in Dictyostelium is unproven, but it may help to segregate two groups of lysosomal proteins from each other. Lysosomal proteins with GlcNAcα-1-P and those with Man-6-P sort to different endosomal-lysosomal vesicles. These vesicles are also functionally distinct since they sequentially fuse with bacteria phagocytosed by the amoeba as seen in Figure 12.3. The cysteine proteinase-containing vesicles fuse first, followed by those containing enzymes with Man-6-P. Surprisingly, pulse chase labeling with bacteria shows that proteins with the two modifications hardly ever reside in the same vesicle, suggesting that the first set of GlcNAcα-1-P-containing proteins is retrieved before the second wave of Man-6-P-containing proteins attacks the bacterial carcass (Figure 12.3). Why do this? It may be because the Dictyostelium endosomal-lysosomal system is constantly changing pH, initially dropping from 6.5 to approximately 5 and then back to 6.5 during the course of 1 hour. The GlcNAcα-1-P-containing proteins, such as the cysteine proteinases in the first wave, have higher pH optima than those in the next wave. In this way, the amoeba can efficiently digest the cargo by taking advantage of the full pH range. Retrieval of the lysosomal enzymes is also highly efficient.
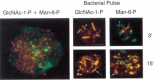
Figure 12.3
(Left panel) Dictyostelium amoeba were stained with an antibody against GlcNAc-1-P (FITC, green) or Man-6-P (TRITC, red). There is no colocalization of the two types of modifications. The yellow areas suggesting colocalization resolve into separate green (more...)
Proteins carrying Fucβ-1-P-Ser were first recognized because they bound to a carbohydrate-specific antibody. Fucβ-1-P occurs on only a limited set of mucin-like proteins that include two lysosomal cysteine proteinases, as well as a set of proteins that are packaged into vesicles containing unassembled spore coat components. Small serine-containing peptides are substrates for Fucβ-1-P transferase. The function of this modification is unknown, but mutants without Fucβ-1-P have more porous spore coats and may have decreased survival in nature.
Manα-1-P-Ser/Thr in Leishmania(17)
The protozoan parasites of the Leishmania genus synthesize a family of phosphorylated glycans important in the life cycle of the organism as it resides in both the insect and mammalian hosts (see also Chapter 39). One component called the proteophosphoglycans (PPG) is composed of large repeating polymers of Man-1-P and galactose (Galβ1–4 Manα1-P) that are O-linked to the mucin-like core proteins through Manα-1-P residues (see Chapter 36). Some of the Manα-1-P-initiated chains are also extended by only an additional mannose residue. Several species of Leishmania synthesize a filamentous secreted acid phosphatase. This molecule contains a Ser/Thr-rich domain that carries chains initiated through Man-1-P and then extended with various combinations of Man, Manα-1-P, Glc, and Gal. It is unknown whether the same Manα-1-P transferase initiates both types of glycans. The function of these chains and the reason they are initiated with Manα-1-P are unknown.
N-linked Modifications (18–19)
There is only one other type of N-glycosylation besides the familiar GlcNAc-β-Asn. Technical barriers to the release, biosynthetic labeling, and identification of putative N-linked sugars may explain the limited membership in this group.
Glcβ-Asn was first identified in Halobacteria where it initiates the major N-glycosylation pathway that involves transfer of a preformed lipid-linked oligosaccharide to the acceptor proteins with Asn-X-Thr/Ser sequences. The pathway has features in common with the N-linked pathway in eukaryotes. This modification is documented on only one vertebrate cell glycoprotein, the basement glycoprotein laminin. Once again, a specific antibody against this structure was the key in finding it in a mammalian glycoprotein. The antigen is localized to the kidney glomerular basement membrane. The presence in laminin of the antigen defined by the Glcβ-Asn antibody was seen only after acid treatment. The acid presumably removed other monosaccharides and exposed Glcβ-Asn to the antibody. The remaining structure was confirmed to be Glcβ-Asn by GC-MS. This is important because it rules out the trivial explanation that the antibody simply cross-reacted with a similar structure. The number of peripheral monosaccharides removed by the acid treatment is unknown, but the results suggest that this pathway could be more complex than the addition of a single glucose unit. The function of Glcβ-Asn is unknown.
C-Glycosylation (20–22)
Another novel type of glycosylation is called C-mannosylation. The C-1 atom of a single mannose residue is added in an α linkage to the C-2 atom of the indole moiety of Trp-7 in RNase 2 (see Figure 12.4). Note that this is not a typical glycosidic linkage, but rather is a C-C bond. This molecule was first detected in human urine. Structural analysis, biosynthetic studies in mammalian cell lines, and a specific antibody against the modification show that C-mannosylation is not a chemically induced artifact. Many mammalian cells transfected with RNase 2 can C-mannosylate this protein, showing that they already contain the necessary transferase. Plant protoplasts and bacteria do not C-mannosylate expressed RNase 2. This may mean that it is a relatively recent form of glycosylation or that the transferase has a different recognition specificity. Site-directed mutagenesis shows that the critical glycosylation sequence in RNase 2 is Trp-X-X-Trp, with the first Trp being modified and X being any amino acid. Synthetic peptides with this motif can be C-mannosylated in vitro, and a database search shows that more than 300 mammalian cell proteins have this sequence. Two of these were examined directly for C-mannosylation. One, the β-chain of fibrinogen B, was not modified, but human interleukin 12β produced in CHO cells was C-mannosylated. These findings suggest that other proteins with the Trp-X-X-Trp motif may be similarly modified. Eight proteins that have thrombospondin type I repeats are also modified by C-mannose. The modification appears to compete with protein folding since already folded proteins are poor substrates in vitro. This finding suggests that the reaction may occur in the ER. The extent of C-mannosylation is variable in different cells. Dol-P-Man produced from GDP-Man is the biosynthetic donor for C-mannosylation. C-mannosylation was only discovered recently, and thus the search for a function of this highly specific modification is only just beginning.

Figure 12.4
C-mannosylation biosynthetic pathway and structure details of Trp-7 in RNase 2.
Glycolipids (23–25)
Some nonvertebrates have “unusual” glycosphingolipids compared to those described for vertebrates in Chapter 9. The glycans are attached to ceramide via Glcβ1 linkage but are often extended with β1–4 mannose. In some cases, the Manβ1–4Glcβ1Cer core can be extended by “typical” structures based on vertebrate standards or by unusual ones that can include internal fucose and sialic acids, methylated monosaccharides, and multiple phosphoethanolamines or phosphonates. Very little is known about the functions of these glycosphingolipids, but a Drosophila cell surface molecule called gliolectin, which is expressed during embryonic development on a subset of glial cells, binds specifically to some neutral and zwitterionic (phosphoethanolamine-containing) glycolipids.
Glycosaminoglycans
The known proteoglycans (except for the keratan sulfate proteoglycans) are initiated by β-xylose to serine, but it is important to remember that nearly all of the studies of proteoglycans, even in nonvertebrates, are focused on examining for a particular type of glycosaminoglycan, e.g., chondroitin or heparan sulfate, in the organism. Few studies have yet focused on the structure of the linkage region itself in nonvertebrate species.
Future Directions
Many unusual forms of glycosylation occur in the Golgi of eukaryotes. In some cases, the modification is limited to a certain class of proteins that share a particular module or have specific conformational features. Some of these forms first appeared in lower organisms and are preserved through evolution. Biological roles are only suggested and the search for their functions continues. The high selectivity for protein targets plus the maintenance of these glycosylations in diverse organisms suggests specific function(s). Other unusual forms of glycosylation will probably be identified in higher organisms by using specific antibodies or rigorous structural analysis. Some of them may be modified versions of those already found in the “treasure chest” of lower eukaryotes and invertebrates.
References
- 1.
- Harris R J, Spellman M W. O-linked fucose and other post-translational modifications unique to EGF modules. Glycobiology. 1993;3:219–224. [PubMed: 8358148]
- 2.
- Wang Y, Spellman M W. Purification and characterization of a GDP-fucose:polypeptide fucosyltransferase from Chinese hamster ovary cells. J. Biol. Chem. 1998;273:8112–8118. [PubMed: 9525914]
- 3.
- Moloney D J, Lin A I, Haltiwanger R S. The O-linked fucose glycosylation pathway. Evidence for protein-specific elongation of O-linked fucose in Chinese hamster ovary cells. J. Biol. Chem. 1997;272:19046–19050. [PubMed: 9228088]
- 4.
- Stults N L, Cummings R D. O-linked fucose in glycoproteins from Chinese hamster ovary cells. Glycobiology. 1993;3:589–596. [PubMed: 8130391]
- 5.
- Nishimura H, Yamashita S, Zeng Z, Walz D A, Iwanaga S. Evidence for the existence of O-linked sugar chains consisting of glucose and xylose in bovine thrombospondin. J. Biochem. 1992;111:460–464. [PubMed: 1618736]
- 6.
- Noelken M E, Hudson B G. Carbohydrate moiety of vertebrate collagens. New Compr. Biochem. 1995;29:589–616.
- 7.
- Colley K J, Baenziger J U. Identification of the post-translational modifications of the core-specific lectin. The core-specific lectin contains hydroxyproline, hydroxylysine, and glucosylgalactosylhydroxylysine residues. J. Biol. Chem. 1987;262:10290–10295. [PubMed: 3611062]
- 8.
- Ma Y, Shida H, Kawasaki T. Functional expression of human mannan-binding proteins (MBPs) in human hepatoma cell lines infected by recombinant vaccinia virus: Post-translational modification, molecular assembly, and differentiation of serum and liver MBP. J. Biochem. 1997;122:810–818. [PubMed: 9399586]
- 9.
- Yuen C T, Chai W, Loveless R W, Lawson A M, Margolis R U, Feizi T. Brain contains HNK-1 immunoreactive O-glycans of the sulfoglucuronyl lactosamine series that terminate in 2-linked or 2,6-linked hexose (mannose). J. Biol. Chem. 1997;272:8924–8931. [PubMed: 9083013]
- 10.
- Chiba A, Matsumura K, Yamada H, Inazu T, Shimizu T, Kusunoki S, Kanazawa I, Kobata A, Endo T. Structures of sialylated O-linked oligosaccharides of bovine peripheral nerve α-dystroglycan. The role of a novel O-mannosyl-type oligosaccharide in the binding of α-dystroglycan with laminin. J. Biol. Chem. 1997;272:2156–2162. [PubMed: 8999917]
- 11.
- Finne J, Krusius T, Margolis R K, Margolis R U. Novel mannitol-containing oligosaccharides obtained by mild alkaline borohydride treatment of a chondroitin sulfate proteoglycan from brain. J. Biol. Chem. 1979;254:10295–10300. [PubMed: 39937]
- 12.
- Haynes P A. Phosphoglycosylation—A new structural class of glycosylation. Glycobiology. 1998;8:1–5. [PubMed: 9451009]
- 13.
- Souza G M, Mehta D P, Lammertz M, Rodriguez-Paris J, Wu R, Cardelli J A, Freeze H H. Dictyostelium lysosomal proteins with different sugar modifications sort to functionally distinct compartments. J. Cell Sci. 1997;110:2239–2248. [PubMed: 9378773]
- 14.
- Mehta D P, Etchison J R, Wu R, Freeze H H. UDP-GlcNAc:Ser-protein N-acetylglucosamine-1-phosphotransferase from Dictyostelium discoideum recognizes serine-containing peptides and eukaryotic cysteine proteinases. J. Biol. Chem. 1997;272:28638–28645. [PubMed: 9353330]
- 15.
- Freeze H.H. 1997. Post-translational modification and sorting of lysosomal enzymes in Dictyostelium: A perspective. In Dictyostelium—A model system for cell and developmental biology (ed. Y. Maeda et al.), pp. 93–107. Universal Academy Press, Inc. and Yamada Science Foundation.
- 16.
- Srikrishna G, Wang L, Freeze H H. Fucose β-1-P-Ser is a new type of glycosylation: Using antibodies to identify a novel structure in Dictyostelium discoideum and study multiple types of fucosylation during growth and development. Glycobiology. 1998;8:799–811. [PubMed: 9639541]
- 17.
- Mengeling B J, Beverley S M, Turco S J. Designing glycoconjugate biosynthesis for an insidious intent: Phosphoglycan assembly in Leishmania parasites. Glycobiology. 1997;7:873–880. [PubMed: 9363429]
- 18.
- Schreiner R, Schnabel E, Wieland F. Novel N-glycosylation in eukaryotes: Laminin contains the linkage unit β-glucosylasparagine. J. Cell Biol. 1994;124:1071–1081. [PMC free article: PMC2119980] [PubMed: 8132707]
- 19.
- Sumper M, Wieland F T. Bacterial glycoproteins. New Comp. Biochem. 1995;29:455–474.
- 20.
- Doucey M A, Hess D, Cacan R, Hofsteenge J. Protein C-mannosylation is enzyme-catalyzed and uses dolichyl-phosphate-mannose as a precursor. Mol. Biol. Cell. 1998;9:291–300. [PMC free article: PMC25252] [PubMed: 9450955]
- 21.
- Krieg J, Hartmann S, Vicentini A, Glasner W, Hess D, Hofsteenge J. Recognition signal for C-mannosylation of TRP-7 in Rnase 2 consists of sequence TRP-X-X-TRP. Mol. Biol. Cell. 1998;9:301–309. [PMC free article: PMC25254] [PubMed: 9450956]
- 22.
- Krieg J, Glasner W, Vicentini A, Doucey M A, Loffler A, Hess D, Hofsteenge J. C-mannosylation of human RNase 2 is an intracellular process performed by a variety of cultured cells. J. Biol. Chem. 1997;272:26687–26692. [PubMed: 9334252]
- 23.
- Wiegandt H. Insect glycolipids. Biochem. Biophys. Acta. 1992;1123:117–126. [PubMed: 1739742]
- 24.
- Stults C L, Sweeley C C, Macher B A. Glycosphingolipids: Structure, biological source, and properties. Methods Enzymol. 1989;179:167–214. [PubMed: 2695766]
- 25.
- Tiemeyer M, Goodman C S. Gliolectin is a novel carbohydrate-binding protein expressed by a subset of glia in the embryonic Drosophila nervous system. Development. 1996;122:925–36. [PubMed: 8631270]
- Other Classes of Golgi-derived Glycans - Essentials of GlycobiologyOther Classes of Golgi-derived Glycans - Essentials of Glycobiology
Your browsing activity is empty.
Activity recording is turned off.
See more...