NCBI Bookshelf. A service of the National Library of Medicine, National Institutes of Health.
Varki A, Cummings R, Esko J, et al., editors. Essentials of Glycobiology. Cold Spring Harbor (NY): Cold Spring Harbor Laboratory Press; 1999.
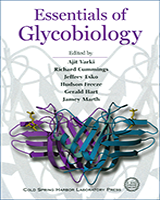
Essentials of Glycobiology.
Show detailsPrimary contributions to this chapter were made by A. Varki (University of California at San Diego).
THIS CHAPTER CONSIDERS PATHWAYS FOR THE BIOSYNTHESIS of the glycosphingolipids, describes the structure and classification of the different core series of these molecules, and outlines general principles regarding their biological roles in membrane structure, modulation of membrane protein function, cell-cell recognition, and host-pathogen interactions. The major emphasis is on higher animal glycosphingolipids, about which the most information is currently available.
Historical Background (1–4)
The glycosphingolipids were originally discovered in the 1940s as lipid-rich substances from normal tissues that accumulated in the tissues of patients with so-called “storage disorders” such as Tay-Sachs disease. Other investigators also extracted glycosphingolipids from the stroma of the erythrocytes of various animals. Their complex and unusual structure as well as their as yet unknown functions caused them to be called sphingolipids, after the enigmatic Egyptian Sphinx. Further structural work showed that these amphipathic molecules consisted of two components: an outer glycan portion attached to an inner lipid tail consisting of a sphingolipid called ceramide. The hydrophobic nature of the sphingolipid component causes these molecules to be embedded in the outer leaflet of cell membranes, along with phospholipids, cholesterol, and glycerolipids. More than 200 structurally distinct glycosphingolipids have been reported from a wide variety of eukaryotic sources.
Defining Structures, Major Classes, and Nomenclature (1–12)
The minimal motif that defines the glycosphingolipids is a monosaccharide attached directly to a ceramide unit. In higher-animal cells, this monosaccharide is usually glucose or galactose, giving glucosylceramide or galactosylceramide (Figure 9.1). Each of these basic units can then be further extended by the stepwise addition of further monosaccharides (Figures 9.2 and 9.3). The number of variations in extension of galactosylceramide is somewhat limited. Glucosylceramide typically has an additional galactose added, giving lactosylceramide, which is then extended in many ways, giving rise to several defined core structures (Table 9.1).

Figure 9.1
Pathways for the biosynthesis of ceramide and monohexosylceramides. The hexose shown is glucose, but the same pathway applies for galactosylceramide. All of the enzymatic steps take place on the cytosolic leaflet of the ER or Golgi membranes. The fatty (more...)

Figure 9.2
Pathways for the biosynthesis of the core structures of the glucosylceramides and galactosylceramides. Initiation takes place on the membranes of the ER or the Golgi. The types of possible outer chains and extensions are shown in Figure 9.3, and details (more...)

Figure 9.3
Pathways for the extension of various glycosphingolipids. The GlcNAc, Gal, or GalNAc residues indicated with an asterisk in Figure 9.2 can be extended in a variety of ways which are shown in this figure in composite form. The boxed structures on the right (more...)
Table 9.1
Names and abbreviations for major core structures of vertebrate glycosphingolipids.
Some of these classes of molecules tend to be enriched in certain tissues types, e.g., the ganglio-series in the brain and the neolacto-series in leukocytes. Although all existing classifications of glycosphingolipids are based on these variations in core glycan structure, it is important to note that the ceramide moiety can also show substantial structural heterogeneity. For example, the typical sphingosine base (Figure 9.1) can vary in the number of double bonds and in the length of the acyl chain. Likewise, the fatty acid attached to the sphingosine via an amide linkage (Figure 9.1) can vary in length from C14 to C24, and in its degree of unsaturation and/or hydroxylation. These variations in ceramide structure are also expressed in a cell-type-specific and developmentally regulated manner, implying that they have some specific purpose. In this regard, one interesting fact is that certain types of glycans are found to be enriched on certain subclasses of ceramides; e.g., in brain gangliosides, the C20 homolog is much more likely to be found in glycosphingolipids with more than one sialic acid residue. Another instance is the ceramide galactosyltransferase that synthesizes GalCer, which displays a marked preference for hydroxy fatty-acid-containing ceramides.
Glycosphingolipids are often simply referred to as glycolipids. This is because the other major class of lipids with attached glycans (the glycosylinositolphospholipids or GPI lipids; see Chapter 10) were only discovered and characterized much later, in the early 1980s. The term ganglioside defines a glycosphingolipid with one or more sialic acid residues (sulfated glycosphingolipids are also called gangliosides by some investigators). There is a well-known nomenclature system suggested by Svennerholm for the ganglio-series gangliosides (Figure 9.4). Despite the subsequent introduction of a more systematized official nomenclature for these molecules, the original system has persisted and prospered, not just because of its historical value, but also because investigators prefer to call specific molecules by a common name that is easy to remember. For example, the ganglioside with the structure: Neu5Acα3Galβ3GalNAcβ4Galβ4Glcβ1Cer would be called II2Neu5Ac-GgOSe4-Cer in the official IUPAC-IUB designation or simply GM1a in the Svennerholm nomenclature. For similar historical reasons, there also remain some other idiosyncracies in the details of glycosphingolipid nomenclature. For example, the structure called sialylparagloboside (Neu5Acα3Galβ4GlcNAcβ3Galβ4Glcβ1Ceramide) is actually based on a neolacto-core (Table 9.1) and not on a globoside core.

Figure 9.4
Pathways for the biosynthesis of the ganglio series glycosphingolipids. Note that the action of the GalNAc transferase commits the molecules to further extension along one of four different pathways; the consequences of genetic disruption of this enzyme (more...)
The glycosphingolipids are clearly significant contributors to the structure of the outer leaflet of most eukaryotic cell membranes. The molar ratio of these molecules relative to the other major membrane lipids (phospholipids, cholesterol, and glycerolipids) varies from being rather minor (e.g. <5% in erythrocytes) to being a very major component (e.g., 30% of the total lipids in neuronal plasma membranes). Although glycosphingolipids are found throughout all eukaryotes, the nature of the core structures can vary in different taxa. For example, invertebrates such as molluscs express a series of glycosphingolipids based on the core structure Manβ1–4Glcβ1–1′Ceramide, and inositolphosphate-ceramides are major sphingolipids in fungi and plants. For further discussion of this matter, see Chapter 3, which considers the evolution of glycan diversity.
Isolation, Purification, and Analysis (3,4,13–15)
Most glycosphingolipids can be obtained in good yield from cells and tissues by sequential organic extractions of increasing polarity. Subsequently, they can be fractionated away from other lipids in the extract and from each other using techniques such as DEAE ion-exchange chromatography and silica gel on either thin-layer plates or columns. High-performance liquid chromatography adaptations of these traditional methods have been particularly powerful in obtaining complete separations of these complex mixtures. For further details regarding the approach to the structural characterization of these molecules, see Chapter 38. It is noteworthy that the base treatment, which is traditionally used to eliminate phospholipids from crude extracts, can damage labile O-acetyl and O-acyl groups in glycosphingolipids. The availability of specific endoglycoceramidases that cleave the glycan portion away from the ceramide has introduced an alternative approach to the their analysis.
Biosynthesis (2,3,6,11,16–26)
The de novo biosynthesis of glycosphingolipids begins on the inner leaflet of membranes in the ER-Golgi pathway. Ceramide is first synthesized by the acylation and desaturation of d-erythro-sphinganine (Figure 9.1) and then glucosylated by a ceramide-specific glucosyltransferase or galactosylated by a specific galactosyltransferase. The glucosylation reaction has been shown to occur on the cytosolic face of ER and Golgi membranes, indicating that there must be a mechanism to “flip” the glucosylceramide into the lumen of the ER-Golgi pathway, where further extension takes place. Galactosylceramide appears to be synthesized with a more conventional topology, primarily within the ER. The next step for glucosylceramides is always the addition of a β-linked galactose residue, giving lactosylceramide. Thereafter, the molecules can be elongated in a stepwise fashion, giving a wide variety of different cores (see above and Figure 9.2). Some of these biosynthetic steps involve mutually exclusive branching events that “commit” the molecule to proceed along certain defined pathways. The classic example is the ganglio series pathway (Figure 9.4) in which the action of a single enzyme (GalNAc transferase) can restrict the subsequent extension of the molecule into the A, B, C, or O series and their subpathways. The outer extensions of glycosphingolipids, including the addition of sialic acids, fucose, blood group structures, or glucuronic acid residues share much in common with those of N- and O-glycans (Figure 9.3; see Chapters 7, 8, and 16). Thus, although the initial steps in glycosphingolipid biosynthesis are catalyzed by unique glycosyltransferases that recognize the hydrophobic nature of the target substrate, many of the outer extensions are thought to be catalyzed by shared glycosyltransferases that may also be involved in glycoprotein biosynthesis (see Chapter 16). However, this sharing of glycosyltransferases has not been formally proven in most instances. In addition, as with N- and O-glycans, the outer monosaccharide residues of glycosphingolipids are targets for additional modifications such as 9(7)-O-acetylation of the sialic acids, O-acylation of galactose residues in galactosylceramide, de-N-acetylation of sialic acids, and O-sulfation.
The organization of the glycosphingolipid biosynthetic steps in the Golgi apparatus has not been fully resolved. There is evidence for sequential subcompartmentation of the enzymatic activities in the order in which they actually work. However, as with the other Golgi glycosylation pathways, evidence also exists for considerable overlap between the compartments. In this regard, drugs that change the organization of the Golgi apparatus (like monensin and Brefeldin A) have yielded very useful, but not completely conclusive, results. Other drugs that can affect glycosphingolipid biosynthesis include glycoside primers (which penetrate the Golgi and act as alternate substrates for glycosphingolipid-modifying enzymes), N-butyldeoxynojirimycin, and the family of ceramide-related compounds that were rationally synthesized by Radin to be specific inhibitors of glucosylceramide synthesis. The first of these was a mixture of d- and l-threo-1-phenyl-2-decanoylamino-3-morpholino-1-propanol (PDMP). These were potent inhibitors of glycosphingolipid synthesis, but each had somewhat different effects and also affected sphingomyelin biosynthesis. Recently, d,l-threo-1-phenyl-2-hexadecanoylamino-3-pyrrolidino-1-propanol•HCl (PPPP) has been reported to be a much more specific inhibitor of glucosylceramide synthesis. An interesting fact is that genetic mutations in the glycosidases involved in glycosphingolipid degradation (see Chapter 18) can be ameliorated by some of these biosynthetic inhibitors (presumably by reducing the load of glycosphingolipids that need to be degraded).
Recent studies have indicated that in addition to de novo synthesis (pathway 1), ceramide for glycosylation can also come from hydrolysis of other sphingolipids (pathway 2) or from recycling of glycosphingolipids in an endosomal pathway (pathway 3). The ratio of these different pathways can vary greatly between cells. It is suggested that when the need for synthesis is low, e.g., in slowly dividing cells, synthesis may predominantly originate from ceramide salvaged from the hydrolytic pathways, whereas the need for increased synthesis is met by up-regulating the de novo biosynthesis pathway.
Trafficking, Turnover, and Degradation (2,7,16,18,20,22,27–39)
Theoretically, glycosphingolipids can enter into and traffic through any post-Golgi membrane compartment. In fact, they have a strong tendency to cluster together (by as yet uncertain physical principles) in the late part of the Golgi and be delivered primarily to the outer leaflet of the plasma membrane. Together with some GPI-anchored proteins, these clusters are proposed to develop into specialized “rafts” that tend to migrate to the apical domain of polarized epithelial cells. Evidence also indicates the existence of separate glycosphingolipid-enriched microdomains (GEMs). In a few cell types, certain glycosphingolipids have been reported to have a primarily internal location (e.g., lactosylceramide is concentrated in the granules of blood neutrophils). Some studies have claimed a truly unusual localization for glycosphingolipids, namely, an association with the cytoskeleton. How the glycosphingolipids are able to flip across the membrane to achieve such a cytosolic localization remains a mystery. In this regard, vimentin-deficient cells have been shown to have a defect in glycolipid synthesis via pathway 2 (see section on biosynthesis above).
Particularly in cell types with a high concentration of glycosphingolipids, the molecules are known to be shed from the surface and can be found in body fluids, often in mixed micelles or apolipoprotein complexes. In some instances, such shed gangliosides have been shown to be taken up by other cells and incorporated into their own membranes, in a manner that would be indistinguishable from that of molecules that were endogenously synthesized. The extent to which such intercellular transfer can take place in vivo is not known. When fluorescently labeled ceramide is added to culture medium, it is taken up into the cells, where it tends to accumulate in the Golgi apparatus, by unknown mechanisms.
Regardless of their original source, glycosphingolipids can be internalized from the cell surface, and then enter endosomal compartments, sometimes becoming part of the inner membranes of multivesicular bodies. Experiments with cultured cell lines suggest that such internalized glycosphingolipids can be remodeled to some extent in late Golgi compartments. However, the extent to which such remodeling actually occurs in vivo is not known. The bulk of the internalized glycosphingolipids eventually undergoes terminal degradation in the lysosomes, via a series of stepwise reactions catalyzed by specific lysosomal enzymes. Some of the final steps involve cleavages that are close to the cell membrane and require facilitation by specific sphingolipid activator proteins. These proteins are thought to “lift” the glycosphingolipids up from the membrane, giving certain glycosidases the ability to act more efficiently. Four of these activator proteins are derived by proteolysis of a single gene product, the sap precursor. In addition to these, the GM2-activator protein is a distinct gene product. For further information regarding the steps involved in lysosomal degradation of glycosphingolipids, as well as about human diseases in which these degrading enzymes are known to be genetically deficient, see Chapter 18. Eventually, glycosphingolipids are broken down to their individual components, which are then available for reutilization in various pathways. An exception may be the final step in removing glucose from glycosyl-ceramide. Some glucosylceramide generated from degradative pathways may be recycled for use in a new round of biosynthesis of glycosphingolipids. In this regard, inhibitors of glycosphingolipid biosynthesis are suggested as agents to reduce the total load of glycosylceramides that accumulate in patients with lysosomal storage disorders.
Relationships to Other Sphingolipids (8,29,30,32,40)
There is a tendency for glycobiologists to focus primarily on the structure and function of the glycan component of the glycosphingolipids. However, by virtue of their ceramide component, glycosphingolipids are also at the crossroads of a variety of other cellular pathways that are of potential biological significance (Figure 9.5). Besides glycosphingolipids, the other major ceramide derivative in most cells is sphingomyelin. This molecule is synthesized by the reaction of phosphorylcholine with ceramide, giving essentially a ceramide with a choline headgroup. Sphingomyelin can constitute the major pool of ceramide in a cell, exceeding even the glycosphingolipids as a whole group. Thus, the ceramide precursor pool may be subject to competition between sphingomyelin synthesis and the initiation of glycosphingolipid synthesis. In this regard, it is of particular interest that a number of extracellular agents and insults (e.g., tumor necrosis factor, Fas ligands, and cancer chemotherapeutic agents) cause the activation of endogenous sphingomyelinases, which then act on sphingomyelin to release ceramide. Many studies indicate an important role for this released ceramide in regulating responses such as cell cycle arrest, apoptosis, and cell senescence. In vitro, ceramide can also activate a serine-threonine protein phosphatase. In intact cells, it seems to regulate protein phosphorylation and some downstream targets such as interleukin converting enzyme (ICE)-like proteases, stress-activated protein kinases, and the retinoblastoma gene product. Thus, there is increasing evidence that ceramide is a key component of the intracellular stress response pathway.
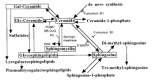
Figure 9.5
Metabolic interrelationships among glycosphingolipids and other ceramide and sphingosine derivatives. Some known inhibitors of the pathways are also shown. (PC) Phosphorylcholine; (DAG) diacylglycerol; (PDMP) d-threo-1-phenyl-2-decanoylamino-3-morpholino-1-propanol; (more...)
Another derivative of ceramide thought to have a signaling function is ceramide-1-phosphate. In addition, a partial breakdown product of galactosylceramide (psychosine, derived by fatty N-deacylation) is known to be a cytotoxic compound that is a strong inhibitor of protein kinase C and an activator of the src kinase. A further variation is the addition of different plasma substitutions to the galactosyl moiety of psychosine. Finally, the sphingosine moiety itself can also be a substrate for formation of a variety of other bioactive compounds that are thought to be involved in cell signaling, including di- and trimethylsphingosine and sphingosine-1-phosphate. In vitro experiments have suggested that several of these compounds can negatively or positively affect the signaling functions of several well-known cellular kinases. However, a coherent and conclusive picture has yet to emerge, and the in vivo significance of these observations remains mostly unproven. Likewise, the metabolic relationship of the ceramide moiety of glycosphingolipids to the rest of the sphingolipids in intact cells is still being worked out (see discussion of the three pathways of biosynthesis above).
Antibodies against Glycosphingolipids (8,29,41–43)
During the early days following the development of monoclonal antibody technology, a concerted effort was made by many investigators to see if such antibodies could differentiate between normal and cancer cells. Of the many “tumor-specific” antibodies that were raised, it was noted that more than half were directed against carbohydrates (see Chapter 35), and the majority of these reacted best with glycosphingolipids. Further studies indicated that most of these antibodies were actually detecting “onco-fetal” antigens, which are expressed at high levels in fetal and cancer tissues and at low levels in normal adult cells. Since that time, a variety of additional monospecific antibodies have been raised by other investigators using glycosphingolipids themselves as immunogens. It should be kept in mind that the immune system of vertebrates tends to have difficulties in generating high-affinity monospecific antibodies against carbohydrate structures. Thus, several of these antibodies (particularly of the IgM variety) may not be as monospecific as they were initially thought to be. Regardless, some of them have been successfully used for diagnostic and prognostic applications in human diseases, and a few are being exploited for attempts at therapy of tumors. Many have also been used to demonstrate cell-type regulation or expression of specific glycosphingolipid structures in a temporal and spatial manner during development. The precise meaning of these findings for the biology of cancer and development is still being explored.
From the pathological point of view, there are some situations wherein humans generate autoimmune antibodies against nervous system glycosphingolipids, associated with the corresponding pathology (see Chapter 37). For example, the Guillian-Barre and Miller-Fisher syndromes that occur following Campylobacter infections are associated with circulating antibodies against certain gangliosides, thought to result from molecular mimicry by bacterial lipooligosaccharide glycans. In addition, in some patients with human multiple myeloma, the clonal population of tumor cells secretes a monoclonal antibody with specificity directed against the sulfoglucuronosyl epitope of nervous system glycosphingolipids. This is associated with a demyelinating peripheral neuropathy which can prove to be worse than the primary tumor itself. Another proposed pathological role for gangliosides occurs in various cancers, which can produce and shed a large amount of these molecules. These shed molecules have been shown to have a strong immunosuppressive effect, via as yet unknown mechanisms.
Biological Roles (3,5,8,26,29,39,40,42,44–50)
There are many putative biological roles of glycosphingolipids, of which only a few have been conclusively established. From a physical point of view, glycosphingolipids may have an organizing role in the cell membrane. For example, glucosylceramides and ceramides are thought to be critical components of the epidermal (skin) permeability barrier. In this regard, it is noteworthy that they are similar to glycophospholipid (GPI) anchors in tending to have long acyl chains (24–26 carbons). It is thought that this common structural feature allows both types of lipids to extend into the inner leaflet of the phospholipid bilayer of cell membranes and also causes them to associate with each other and with cholesterol, forming putative “rafts” in the micromembrane (some investigators report the existence of distinct glycosphingolipid-enriched domains free of cholesterol that are thought to be involved in signal transduction via cytosolic proteins). Such aggregations of glycosphingolipids and GPI-anchored proteins are thought to form in the trans-Golgi region and then get targeted to the apical domains of polarized epithelial cells that line the lumen of various body cavities. Here, they can represent as much as 50% of the total lipid concentration of the outer leaflet of the apical membrane, likely providing physical protection against the hostile environment often encountered in such lumens, as well as specific binding sites for the adhesion of symbiotic bacteria. In the same location, other structural aspects of the same molecule can be utilized as highly specific receptor targets for a variety of bacteria, toxins, and viruses. The classic and well-established finding is that the B subunit of cholera toxin binds to the ganglioside GM1, triggering a conformational change and delivering the toxic A subunit to the interior of the cell (for additional specific examples, see Table 9.2). These types of interactions are obviously not of benefit to the organism that originally synthesized the glycosphingolipid in question.
Table 9.2
Examples of reported interactions between defined glycosphingolipids and specific binding proteins.
A recurring theme in the literature is the specific physical association of certain glycosphingolipids with certain cell membrane receptors (Table 9.2 gives several examples). Although the physical mechanism of such interactions is not certain, there is considerable evidence indicating that they can alter the biology of these receptors. For example, the tyrosine phosphorylation of the EGF receptor is specifically down-regulated by adding the ganglioside GM3, whereas the closely related structure sialylparagloboside has a similar effect only on the insulin receptor. A small modification of the GM3 molecule (de-N-acetylation) results in exactly the opposite response (stimulation of EGF receptor tyrosine phosphorylation). Related studies demonstrate the opposing effects of GM3 and lactosylceramide on cell growth. However, many of these effects are demonstrated following the exogenous addition of glycosphingolipids to cells. Thus, although at least a portion of the added glycosphingolipids are incorporated into the membrane, one cannot rule out other effects caused by the micellar forms of the added glycosphingolipids that can also be bound to cell surface proteins. In balance, the stereospecificity of these effects for the fine structure of the glycan portion of the glycosphingolipids implies that defined mechanisms must be involved.
Similar findings have been reported for the specific effects of ganglioside GQ1b in inducing neuritogenesis, and a suggestion has been made that this involves the NGF receptor and/or an ectoprotein kinase. Again, final mechanistic conclusions are lacking. Regardless, these findings have encouraged other investigators to try ganglioside infusions in a variety of central nervous system disorders, including stroke and demyelinating disorders. Particularly in stroke, there are several reports of beneficial effects of infusing gangliosides into the bloodstream of patients. Likewise, direct topical applications of conduritol B epoxide, a specific irreversible inhibitor of β-glucocerebrosidase, increased GlcCer levels in the basal, proliferative cell layer (fourfold increase) and stimulated proliferation. Simultaneous treatment with conduritol B epoxide plus GlcCer resulted in an additive increase in DNA synthesis, strongly suggesting that GlcCer directly stimulates epidermal mitogenesis.
It has also been suggested that gangliosides are involved in thermal adaptation of neuronal membranes. The data suggest a general rule that “the lower the environmental temperature the more polar is the composition of brain gangliosides.” Studies with model bilayer membranes also indicate that gangliosides can modulate basic membrane properties in a thermosensitive manner. Hakomori and colleagues have also provided evidence that glycosphingolipids can mediate low-affinity but high-specificity carbohydrate-carbohydrate interactions between different cell types. In particular, they propose that glycolipids bearing the Lewis X motif may help to mediate compaction of the early mouse embryo at the stage of morula formation (see Chapter 34). In most other instances, the biological significance of such carbohydrate-carbohydrate interactions has yet to be formally proven.
Natural and Induced Disorders in Biosynthesis (36,51–56)
As mentioned above, genetic defects in a variety of lysosomal enzymes and in the sphingolipid activator proteins result in distinct storage diseases that are characterized by the accumulation of specific intermediates that cannot be further degraded. These diseases are considered further in Chapter 18. The existence of a cultured cell line that is completely deficient in glucosylceramide synthase indicates that the glucosylceramide series of glycosphingolipids are not essential for the growth of single cells in a culture dish and that the complexities of glycosphingolipid structures have their critical functions primarily in the intact multicellular animal. In fact, in contrast to the large number of metabolic errors found in the glycosphingolipid degradative pathways, there are very few naturally defined genetic defects in the biosynthesis of glycosphingolipids. On the other hand, dramatic changes in glycosphingolipid expression occurring during vertebrate ontogeny and cellular activation indicate that the complexities of glycosphingolipid biosynthesis may be required in the intact animal.
Targeted genetic mutations in an intact mammal are obviously required to define the true in vivo biological roles of glycosphingolipids (see Table in Chapter 33). Some of the gene “knockouts” affecting the outer chains of N- and O-glycans (e.g., FucT-VII and α1–3 galactosyltransferase; see Chapters 17, 18, 32, and 33) may well affect outer-chain structures on glycosphingolipids as well; this has yet to be investigated systematically. So far, only two gene disruptions that selectively affect glycosphingolipid synthesis have been performed. Elimination of the ceramide galactosyltransferase has given the most interesting and provocative results. Since the proximate products of this enzyme reaction (GalCer and sulfatides) are major components of the myelin sheaths of axons in the vertebrate nervous system, investigators expected to see a complete disorganization of myelin. Surprisingly, the mice retained the ability to form myelin. However, this myelin contained GlcCer, which had apparently substantially replaced the GalCer. Despite myelin of relatively normal ultrastructural appearance, the mice showed generalized tremors and mild ataxia, and electrophysiological analysis showed conduction deficits consistent with diminished insulation capacity of the myelin sheath. Furthermore, with increasing age, the mutant mice developed progressive hindlimb paralysis and severe vacuolation of the ventral region of the spinal cord. These results indicate that GalCer and sulfatide have important roles in myelin function and stability.
The biggest surprise in this field has arisen from the genetic ablation of the GalNAc transferase (GM2/GD2 synthase) that is required for the formation of most of the complex gangliosides of the ganglio series (see Figure 9.4). Despite the lack of these major gangliosides, the mice showed no major defects in their nervous systems and no gross functional changes, only a slight reduction in neural conduction velocity in some peripheral nerves. An increase in GM3 and GD3 occurs in the brains of these mutant mice and seems to be sufficient to compensate for the lack of complex gangliosides. These findings indicate that contrary to all prior expectations, the complex gangliosides are not required for the primary morphogenesis and organogenesis of the brain. As is often the case in such studies, further analyses have revealed more subtle defects in these animals, e.g., sporadic axonal degeneration and a major defect in spermatogenesis. An alternative approach is the overexpression of the same gene in transgenic mice. In this case, the mice showed about tenfold higher expression of the GM2/GD2 synthase gene in tissues such as the skin, and the endogenous gangliosides were dramatically converted away from GM3 toward GM1. Although no gross morphological changes were observed, much stronger inflammatory reactions involving neutrophils were observed in the transgenic mice, suggesting an increased sensitivity of neutrophils to chemotactic factors in the transgenic mice.
Overall, one must conclude that the complexities of at least the ganglio series of glycosphingolipids may not be required for most normal organ formation in the mouse embryo. Supporting this notion has been the lack of effects of inhibitors of glucosylceramide synthesis on the in vitro development of embryos of the medaka fish and the mouse. In the latter experiments, it was even shown that the level of glycosphingolipid production had fallen to approximately 90% of that in the control. These data indicate either that very small levels of glycosphingolipids are sufficient to mediate their critical roles in development or that these dramatic patterns of selective expression have some other as yet undefined purpose. One possibility (discussed further in Chapter 3) is that some of this diversity is actually meant to evade recognition by exogenous pathogens and toxins. In this scenario, the extensive diversity of glycosphingolipid structures would assure that pathogens or toxins with exquisite specificity for recognition of glycosphingolipids could only affect a few cells within a given host or host organ.
Future Directions
Glycosphingolipids possess a remarkable degree of structural diversity, and numerous enzymes are involved in their synthesis, recycling, and turnover. Ultimately, the understanding of their specific functions will require the genetic dissection of each step in their synthesis. The cloning of these enzymes, and the generation and analysis of mutant animals, is a formidable but necessary task. Moreover, such animals will have to not only be analyzed for intrinsic pathophysiology, but also be exposed to a variety of specific pathogens, to see if they show altered disease susceptibility.
References
- 1.
- Stults C L M, Sweeley C C, Macher B A. Glycosphingolipids: Structure, biological source, and properties. Methods Enzymol. 1989;179:167–214. [PubMed: 2695766]
- 2.
- Sandhoff K, Van Echten G. Ganglioside metabolism—Topology and regulation. Adv. Lipid Res. 1993;26:119–138. [PubMed: 8379447]
- 3.
- Nagai Y. and Iwamori M. 1995. Cellular biology of gangliosides. In Biology of the sialic acids (ed. Rosenberg A.), pp. 197–241. Plenum Press, New York.
- 4.
- Wiegandt H. The chemical constitution of gangliosides of the vertebrate nervous system. Behav. Brain Res. 1995;66:85–97. [PubMed: 7755905]
- 5.
- Karlsson K -A. Animal glycosphingolipids as membrane attachment sites for bacteria. Annu. Rev. Biochem. 1989;58:309–350. [PubMed: 2673013]
- 6.
- Shayman J A, Radin N S. Structure and function of renal glycosphingolipids. Am. J. Physiol. 1991;260:F291–F302. [PubMed: 2000947]
- 7.
- Hakomori S, Igarashi Y. Functional role of glycosphingolipids in cell recognition and signaling. J. Biochem. 1995;118:1091–1103. [PubMed: 8720120]
- 8.
- Hakomori S. Tumor malignancy defined by aberrant glycosylation and sphingo(glyco)lipid metabolism. Cancer Res. 1996;56:5309–5318. [PubMed: 8968075]
- 9.
- Dasgupta S, Everhart M B, Bhat N R, Hogan E L. Neutral monoglycosylceramides in rat brain: Occurrence molecular expression and developmental variation. Dev. Neurosci. 1997;19:152–161. [PubMed: 9097030]
- 10.
- Ishizuka I. Chemistry and functional distribution of sulfoglycolipids. Prog. Lipid Res. 1997;36:245–319. [PubMed: 9640458]
- 11.
- Ichikawa S, Hirabayashi Y. Glucosylceramide synthase and glycosphingolipid synthesis. Trends Cell Biol. 1998;8:198–202. [PubMed: 9695839]
- 12.
- Ogawa-Goto K, Abe T. Gangliosides and glycosphingolipids of peripheral nervous system myelins—A minireview. Neurochem. Res. 1998;23:305–310. [PubMed: 9482242]
- 13.
- Izu H, Izumi Y, Kurome Y, Sano M, Kondo A, Kato I, Ito M. Molecular cloning expression, and sequence analysis of the endoglycoceramidase II gene from Rhodococcus species strain M-777. J. Biol. Chem. 1997;272:19846–19850. [PubMed: 9242646]
- 14.
- Wang L X, Pavlova N V, Li S C, Li Y T, Lee Y C. A fluorometric assay of ceramide glycanase with 4-methylumbelliferyl β-d-lactoside derivatives. Glycoconj. J. 1996;13:359–365. [PubMed: 8781966]
- 15.
- Li Y -T, Li S -C. Ceramide glycanase from leech, Hirudo medicinalis, and earthworm, Lumbricus terrestris. Methods Enzymol. 1989;179:479–487. [PubMed: 2695772]
- 16.
- Hoekstra D, Kok J W. Trafficking of glycosphingolipids in eukaryotic cells: Sorting and recycling of lipids. Biochim. Biophys. Acta Rev. Biomembr. 1992;1113:277–294. [PubMed: 1450202]
- 17.
- Freeze H H, Sampath D, Varki A. α- and β-xylosides alter glycolipid synthesis in human melanoma and Chinese hamster ovary cells. J. Biol. Chem. 1993;268:1618–1627. [PubMed: 8420936]
- 18.
- Van Echten G, Sandhoff K. Ganglioside metabolism. Enzymology, topology, and regulation. J. Biol. Chem. 1993;268:5341–5344. [PubMed: 8449895]
- 19.
- Stoffel W, Bosio A. Myelin glycolipids and their functions. Curr. Opin. Neurobiol. 1997;7:654–661. [PubMed: 9384539]
- 20.
- Li R X, Kong Y, Ladisch S. Nerve growth factor-induced neurite formation in PC12 cells is independent of endogenous cellular gangliosides. Glycobiology. 1998;8:597–603. [PubMed: 9592126]
- 21.
- Nomura T, Takizawa M, Aoki J, Arai H, Inoue K, Wakisaka E, Yoshizuka N, Imokawa G, Dohmae N, Takio K, Hattori M, Matsuo N. Purification, cDNA cloning, and expression of UDP-Gal: Glucosylceramide β-1,4-galactosyltransferase from rat brain. J. Biol. Chem. 1998;273:13570–13577. [PubMed: 9593693]
- 22.
- Platt F M, Neises G R, Reinkensmeier G, Townsend M J, Perry V H, Proia R L, Winchester B, Dwek R A, Butters T D. Prevention of lysosomal storage in Tay-Sachs mice treated with N-butyldeoxynojirimycin. Science. 1997;276:428–431. [PubMed: 9103204]
- 23.
- Augusteyn R C, de Jersey J, Webb E C, Zerner B. On the homology of the active-site peptides of liver carboxylesterases. Biochim. Biophys. Acta. 1969;171:128–137. [PubMed: 4884138]
- 24.
- Watanabe R, Wu. K, Paul P, Marks D L, Kobayashi T, Pittelkow M R, Pagano R E. Up-regulation of glucosylceramide synthase expression and activity during human keratinocyte differentiation. J. Biol. Chem. 1998;273:9651–9655. [PubMed: 9545298]
- 25.
- Gillard B K, Clement R G, Marcus D M. Variations among cell lines in the synthesis of sphingolipids in de novo and recycling pathways. Glycobiology. 1998;8:885–890. [PubMed: 9675221]
- 26.
- Lloyd K O, Furukawa K. Biosynthesis and functions of gangliosides: Recent advances. Glycoconj. J. 1998;15:627–636. [PubMed: 9881769]
- 27.
- O'Brien J S, Kishimoto Y. Saposin proteins: Structure, function, and role in human lysosomal storage disorders. FASEB J. 1991;5:301–308. [PubMed: 2001789]
- 28.
- Gillard B K, Thurmon L T, Marcus D M. Variable subcellular localization of glycosphingolipids. Glycobiology. 1993;3:57–67. [PubMed: 8448386]
- 29.
- Hakomori S, Igarashi Y. Gangliosides and glycosphingolipids as modulators of cell growth, adhesion, and transmembrane signaling. Adv. Lipid Res. 1993;25:147–162. [PubMed: 8396311]
- 30.
- Hannun Y A. The sphingomyelin cycle and the second messenger function of ceramide. J. Biol. Chem. 1994;269:3125–3128. [PubMed: 8106344]
- 31.
- Radin N S. Treatment of Gaucher disease with an enzyme inhibitor. Glycoconj. J. 1996;13:153–157. [PubMed: 8737239]
- 32.
- Sandhoff K, Kolter T. Topology of glycosphingolipid degradation. Trends Cell Biol. 1996;6:98–103. [PubMed: 15157485]
- 33.
- Van der Bijl P, Strous G J, Lopes-Cardozo M, Thomas-Oates J, van Meer G. Synthesis of non-hydroxy-galactosylceramides and galactosyldiglycerides by hydroxy-ceramide galactosyltransferase. Biochem. J. 1996;317:589–597. [PMC free article: PMC1217527] [PubMed: 8713090]
- 34.
- Hooper N M. Membrane biology: Do glycolipid microdomains really exist? Curr. Biol. 1998;8:R114–R116. [PubMed: 9501972]
- 35.
- Kniep B, Skubitz K M. Subcellular localization of glycosphingolipids in human neutrophils. J. Leukocyte Biol. 1998;63:83–88. [PubMed: 9469476]
- 36.
- Kolter T, Sandhoff K. Recent advances in the biochemistry of sphingolipidoses. Brain Pathol. 1998;8:79–100. [PMC free article: PMC8098506] [PubMed: 9458169]
- 37.
- Schapiro F B, Lingwood C, Furuya W, Grinstein S. pH-independent retrograde targeting of glycolipids to the Golgi complex. Am. J. Physiol. 1998;274:C319–C332. [PubMed: 9486120]
- 38.
- Gillard B K, Clement R, Colucci-Guyon E, Babinet C, Schwarzmann G, Taki T, Kasama T, Marcus D M. Decreased synthesis of glycosphingolipids in cells lacking vimentin intermediate filaments. Exp. Cell Res. 1998;242:561–572. [PubMed: 9683542]
- 39.
- Hakomori S, Handa K, Iwabuchi K, Yamamura S, Prinetti A. New insights in glyosphingolipid function: “Glycosignaling domain,” a cell surface assembly of glycosphingolipids with signal transducer molecules, involved in cell adhesion coupled with signaling. Glycobiology. 1998;8:XI–XVIII. [PubMed: 9840984]
- 40.
- Hannun Y A. Functions of ceramide in coordinating cellular responses to stress. Science. 1996;274:1855–1859. [PubMed: 8943189]
- 41.
- Marcus D M, Schwarting G A. Immunochemical properties of glycolipids and phospholipids. Adv. Immunol. 1976;23:203–240. [PubMed: 793358]
- 42.
- Feizi T. Demonstration by monoclonal antibodies that carbohydrate structures of glycoproteins and glycolipids are onco-developmental antigens. Nature. 1985;314:53–57. [PubMed: 2579340]
- 43.
- Fredman P, Lekman A. Glycosphingolipids as potential diagnostic markers and/or antigens in neurological disorders. Neurochem. Res. 1997;22:1071–1083. [PubMed: 9239764]
- 44.
- Tsuji S, Yamashita T, Matsuda Y, Nagai Y. A Novel Glycosignaling System: Gq1b-dependent Neuritogenesis of Human Neuroblastoma Cell Line, Goto, is Closely Associated with Gq1b-dependent Ecto-type Protein Phosphorylation. Neurochem. Int. 1992;21:549–554. [PubMed: 1303737]
- 45.
- Kielczynski W, Bartholomeusz R K, Harrison L C. Characterization of ganglioside associated with the thyrotrophin receptor. Glycobiology. 1994;4:791–796. [PubMed: 7734842]
- 46.
- Karlsson K A. Microbial recognition of target-cell glycoconjugates. Curr. Opin. Struct. Biol. 1995;5:622–635. [PubMed: 8574698]
- 47.
- Nagai Y. Functional roles of gangliosides in bio-signaling. Behav. Brain Res. 1995;66:99–104. [PubMed: 7755906]
- 48.
- Kasahara K, Watanabe Y, Yamamoto T, Sanai Y. Association of Src family tyrosine kinase Lyn with ganglioside GD3 in rat brain—Possible regulation of Lyn by glycosphingolipid in caveolae-like domains. J. Biol. Chem. 1997;272:29947–29953. [PubMed: 9368072]
- 49.
- Simons K, Ikonen E. Functional rafts in cell membranes. Nature. 1997;387:569–572. [PubMed: 9177342]
- 50.
- Boubelik M, Floryk D, Bohata J, Dráberová L, Macák J, Smíd F, Dráber P. Lex glycosphingolipids-mediated cell aggregation. Glycobiology. 1998;8:139–146. [PubMed: 9451023]
- 51.
- Fenderson B A, Ostrander G K, Hausken Z, Radin N S, Hakomori S. A ceramide analogue (PDMP) inhibits glycolipid synthesis in fish embryos. Exp. Cell Res. 1992;198:362–366. [PubMed: 1729139]
- 52.
- Ichikawa S, Nakajo N, Sakiyama H, Hirabayashi Y. A mouse B16 melanoma mutant deficient in glycolipids. Proc. Natl. Acad. Sci. 1994;91:2703–2707. [PMC free article: PMC43438] [PubMed: 8146177]
- 53.
- Coetzee T, Fujita N, Dupree J, Shi R, Blight A, Suzuki K, Popko B. Myelination in the absence of galactocerebroside and sulfatide: Normal structure with abnormal function and regional instability. Cell. 1996;86:209–219. [PubMed: 8706126]
- 54.
- Takamiya K, Yamamoto A, Furukawa K, Yamashiro S, Shin M, Okada M, Fukumoto S, Haraguchi M, Takeda N, Fujimura K, Sakae M, Kishikawa M, Shiku H, Aizawa S. Mice with disrupted GM2/GD2 synthase gene lack complex gangliosides but exhibit only subtle defects in their nervous system. Proc. Natl. Acad. Sci. 1996;93:10662–10667. [PMC free article: PMC38211] [PubMed: 8855236]
- 55.
- Brigande J V, Platt F M, Seyfried T N. Inhibition of glycosphingolipid biosynthesis does not impair growth or morphogenesis of the postimplantation mouse embryo. J. Neurochem. 1998;70:871–882. [PubMed: 9453585]
- 56.
- Takamiya K, Yamamoto A, Furukawa K, Zhao J M, Fukumoto S, Yamashiro S, Okada M, Haraguchi M, Shin M, Kishikawa M, Shiku H, Aizawa S. Complex gangliosides are essential in spermatogenesis of mice: Possible roles in the transport of testosterone. Proc. Natl. Acad. Sci. 1998;95:12147–12152. [PMC free article: PMC22799] [PubMed: 9770454]
- Historical Background
- Defining Structures, Major Classes, and Nomenclature
- Isolation, Purification, and Analysis
- Biosynthesis
- Trafficking, Turnover, and Degradation
- Relationships to Other Sphingolipids
- Antibodies against Glycosphingolipids
- Biological Roles
- Natural and Induced Disorders in Biosynthesis
- Future Directions
- References
- Glycosphingolipids - Essentials of GlycobiologyGlycosphingolipids - Essentials of Glycobiology
- txid1816218[Organism:noexp] (2)Nucleotide
Your browsing activity is empty.
Activity recording is turned off.
See more...