NCBI Bookshelf. A service of the National Library of Medicine, National Institutes of Health.
Varki A, Cummings R, Esko J, et al., editors. Essentials of Glycobiology. Cold Spring Harbor (NY): Cold Spring Harbor Laboratory Press; 1999.
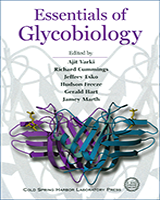
Essentials of Glycobiology.
Show detailsPrimary contributions to this chapter were made by H.H. Freeze (The Burnham Institute, La Jolla, California).
THIS CHAPTER EXPLORES THE DEGRADATION AND TURNOVER of glycoconjugates. Most glycoconjugates are degraded in lysosomes, and a portion of the liberated monosaccharides are reused for glycoconjugate synthesis (see Chapter 6). The degradation of glycans is ordered and often highly specific. It involves both endo- and exoglycosidases that eventually liberate monosaccharides, sometimes with the aid of noncatalytic proteins. Work on a series of rare human genetic disorders called lysosomal storage diseases was critical to unraveling these complex pathways (see Chapter 23). In each disease, a limited number of undegraded molecules accumulate in the lysosomes. Clever experiments combining enzymology with carbohydrate structural analysis revealed the steps of the pathways and also unlocked the mechanism of lysosomal enzyme targeting as described in Chapter 23. The dismantling of selected glycans is described, since each illustrates unique features.
The Lysosomal Enzymes (1–2)
Most of the endo- and exoglycosidases that degrade sugar chains and their modifications have pH optima between 4.0 and 5.5, but exceptions exist. Some lysosomal enzymes have much higher pH optima. Exoglycosidases cleave the glycosidic linkage of terminal sugars from the nonreducing end of the chain. By convention, that means that the residue at the outermost end of the molecule is at the extreme left end in a graphical depiction. The exoglycosidases recognize only one (occasionally two) monosaccharide together with its anomeric linkage, but they are much less particular about the structure of the molecule beyond the glycosidic linkage. This feature allows these enzymes broad specificity. However, exoglycosidases do not usually act unless all of the hydroxyl groups of the substrate sugar are unmodified. Substitutions such as O-acetyl groups, sulfate, or phosphate esters usually must be removed before exoglycosidases can work. Esterases cleave acetyl groups, and specific sulfatases cleave various sulfate groups from glycosaminoglycans and from N- or O-linked sugar chains before exoglycosidase cleavage. The endoglycosidases cleave internal glycosidic linkages of larger chains, yielding fragments that can then be degraded by exoglycosidases. Endoglycosidases are often more tolerant of modifications of the sugar chain residues, and in some cases, they even require them for optimal cleavage.
Even though the lysosomal glycosidases carry out similar reactions, they have only about 15–20% identical and 40–45% similar amino acids. There are no highly conserved “glycosidase” catalytic domains. Lysosomal enzymes are all N-glycosylated and most are targeted to the lysosome by the Man-6-P pathway discussed in Chapter 23. Therefore, they must share aspects of the recognition marker for GlcNAc-phosphotransferase and variable affinities for the large and small Man-6-P receptors. The concentration of enzymes within the lysosome is difficult to determine, but proteinases such as cathepsins B, D, and L have been estimated at 1 mm. Glycosidases are probably present at much lower concentrations.
Genetic Defects in Lysosomal Degradation of Glycans (1,3–5,9–10)
Loss of a lysosomal enzyme leads to accumulation of undegraded material in tissues and secretion of fragments into the urine. Most of the human disorders listed in Table 18.1 have animal models. Most of these affect glycoprotein degradation, except for the loss of β-galactosidase and β-N-acetylhexosaminidase, which also affects glycolipids.
Table 18.1
Defects in glycoprotein degradation.
Tables 18.1, 18.2, and 18.3 also show some of the major clinical symptoms of diseases associated with degradation of each type of glycoconjugate. In comparing all of the different diseases in these three tables, two features stand out. First, many of the diseases share overlapping symptoms, and yet each disease has unique features that rely on experienced clinicians for specific diagnosis. Second, many of the diseases present with a range of severities. Usually an infantile onset is the most severe, and the juvenile or adult onsets are milder. The later-onset forms may even affect different systems than those affected by the early-onset forms. It is not clear how accumulating different types of “undegraded material” in a lysosome leads to the different symptoms in each disease. It may mean that accumulating material is not the cause of different clinical manifestations, but it is an indicator of its severity. To the best of our knowledge, the cell has no way of sensing the chemical nature of accumulated material. The “lysosomal storage disease” label provides a convenient unifying theme. The range of clinical severities and time of onset may be related to the amount of residual activity in the defective enzyme and to the fine balance between synthesis and degradation of multiple glycans. Reducing synthesis somewhat may help to retard accumulation of undegraded material. These aspects are discussed later in this chapter.
Table 18.2
Mucopolysaccharidoses: Classification of defects and clinical symptoms.
Table 18.3
Disorders in glycolipid degradation.
Glycoprotein Degradation
The great majority of N- and O-linked oligosaccharide chains reaching the lysosome contain only six sugars—β-GlcNAc, α/β-GalNAc, α/β-Gal, α/β-Man, α-Fuc, α-Sia—and each should theoretically require only one anomer-specific glycosidase, assuming that each enzyme cleaves its substrate when bound to any other component. This number is actually quite close to the known number of enzymes in the degradation pathways. However, some linkages require a specific enzyme outside of this group, β-N-acetylhexosaminidase cleaves both β-GlcNAc and β-GalNAc residues, and degradation of the GlcNAcβ-Asn and GalNAcα-Ser/Thr linkages also requires specific enzymes.
Complex N-linked Chains (1,3–5)
Much of what we know of this pathway comes from analysis of products that accumulate in patients missing one of the degradative enzymes (Table 18.1) or from structural analysis of monosaccharide-labeled glycoproteins during degradation in perfused rat liver. By carrying out the latter studies in the presence of inhibitors of different lysosomal enzymes, a picture emerges of simultaneous and independent bidirectional degradation of the protein and the carbohydrate chains (Figure 18.1). The relative degradation rates vary depending on structural and steric factors of the protein and the sugar chains. The accumulation of GlcNAcβ1–4GlcNAcβ-Asn in cells that cannot cleave the GlcNAcβ-Asn linkage clearly shows that degradation of the sugar chain does not require prior cleavage from the protein. Much of the protein is probably degraded before N-glycan catabolism begins. Removal of the core fucose (Fucα1–6GlcNAc) and probably any peripheral fucose residues linked to the outer branches of the chain (Fucα1–3GlcNAc) appears to be the first step in degradation, since patients lacking this enzyme still have intact N-linked chains bound to asparagine. Glycosylasparaginase (aspartyl-N-acetyl-β-d-glucosaminidase) then cleaves the GlcNAcβ-Asn bond, provided the α-amino group is not in peptide linkage. In rodents and primates, chitobiase (an endo-β-N-acetylglucosaminidase) removes the reducing GlcNAc, leaving the oligosaccharide with only one terminal GlcNAc. In many other species, splitting of the chitobiose linkage (GlcNAcβ1–4GlcNAc) uses the β-N-acetylhexosaminidase mentioned below as the last step in degradation. Because either pathway appears to get the job done, the presence of this endo-β-N-acetylglucosaminidase (also called chitobiase) in some species is unexplained. The oligosaccharide chain is then sequentially degraded by sialidases and/or α-galactosidase and then β-galactosidase, β-N-acetylhexosaminidase, and α-mannosidases. The remaining Manβ1–4GlcNAc is split by mannosidase to mannose and GlcNAc or, in those species that do not have chitobiase, to chitobiose, which is then degraded by β-N-acetylhexosaminidase.

Figure 18.1
Degradation of complex N-linked oligosaccharide chains in primates and rodents. The lysosomal degradation pathway of glycoproteins carrying complex-type oligosaccharides proceeds simultaneously on the protein and oligosaccharide moieties. The oligosaccharides (more...)
Lysosomal sialidase (neuraminidase), β-galactosidase, and a serine carboxypeptidase called protective protein/cathepsin A form a complex in the lysosome that is required for efficient degradation of sialylated glycoconjugates. Cathepsin A protects β-galactosidase from rapid degradation and also activates the sialidase precursor. Mutations in this protective protein lead to galactosialidosis in which the simultaneous deficiencies in β-galactosidase and α-sialidase are secondary effects.
Other oligosaccharides having GalNAcβ1–4GlcNAc or GlcAβ1–3Gal or GalαGal on the outer branches must first have these residues removed by β-N-acetyl-hexosaminidase, β-glucuronidase, and α-galactosidase prior to any further digestion of the underlying oligosaccharide chain.
High-mannose-type Oligosaccharides (6–7)
High-mannose-type chains are hydrolyzed in the lysosome by an α-mannosidase to yield Manα1-6Manβ1–4GlcNAc, a common intermediate derived from hybrid and complex chains, in addition to the high-mannose type. A second α1,6-specific mannosidase can cleave this linkage in humans and rats, but only on molecules that have a single core region GlcNAc, i.e., those generated by chitobiase cleavage. Finally, β-mannosidase completes the job.
ER and Cytosolic Degradation of Oligosaccharides Derived from Dolichol Precursors and Misfolded Glycoproteins (6–8)
High-mannose-type oligosaccharides are also partially degraded in the cytosol by a different set of α-mannosidases with higher pH optima. These enzymes degrade free glycans liberated from the dolichol-linked precursors or from newly synthesized but misfolded glycoproteins that are en route to proteasome-mediated degradation. The degradation of the lipid-linked precursor begins with a pyrophosphatase cleave of the nonglucosylated cytosolic-facing precursor, primarily Man5. Normally, the next step in the biosynthetic pathway involves flipping the lipid-linked oligosaccharide to the lumen of the ER for completion. Oligosaccharide precursors that fail to flip may be degraded in this way. Once the phosphorylated Man5 chain is liberated from the lipid, a phosphatase and a cytosolic endo-β-N-acetylglucosaminidase remove the phosphate and reducing GlcNAc. Other dolichol-linked oligosaccharides may also be hydrolyzed within the lumen of the ER, and transported into the cytosol, but the details of this pathway and its relation to cleavage of oligosaccharides from proteins in the ER are less clear. Regardless of the mechanism, the cytosolic endo-β-N-acetylglucosaminidase mentioned above (a chitobiase distinct from the lysosomal one) clips a single GlcNAc. Several mannose residues are also removed, producing the same Man5 structure mentioned above. This molecule is then imported into the lysosome for its final degradation. Up to one third of the total lipid-linked oligosaccharides can be recovered as free glycans. These cleavages may be important for coordinating the level of oligosaccharide intermediates with protein synthetic rates.
Poorly folded glycoproteins were previously thought to be degraded in the ER, but more recently, it has become clear that this degradation occurs in the cytosol as an ATP-dependent process. The emerging view is that misfolded glycoproteins are reverse-translocated into the cytoplasm through the Sec61 translocation channel in the ER. The release of the polypeptide into the cytoplasm depends on ubiquitination, and final degradation is proteasome-dependent. Depending on the protein, the N-glycan may require a small amount of mannose trimming prior to ubiquitination, but complete degradation of the protein requires removal of all N-glycans using a cytosolic peptide-N-glycoamidase (not endoglycosidase) activity. These liberated oligosaccharides can then be cleaved by the cytosolic endo-β-N-acetylglucosaminidase and α-mannosidases similar to the degradation of the chains liberated from lipid-linked oligosaccharide.
Some evidence suggests that the removal of glycan chains from proteins might be physiologically important. Proximal peptide-N-glycoamidases can release the entire oligosaccharide chain, and in doing so, they convert an asparagine to an aspartic acid residue. Amino acid sequencing of some proteins shows aspartic acid where the DNA sequence predicts asparagine. The liberated glycans cannot be simply added to other glycoproteins in the ER, since addition requires the pyrophosphate-linked dolichol donors and not just the sugar chain itself. The liberated chains may need to be exported to prevent them from competing with ER lectins such as calnexin, calreticulin, or ERGIC53, a mannose-binding lectin in the ER.
O-Linked Oligosaccharides (9)
Degradation of typical α-GalNAc-initiated O-glycans has not been systematically studied. Since so many of the structures seen on N-linked chains are also found on O-glycans, they are probably degraded by the same group of exoglycosidases discussed above. The exception to this of course is the linkage region, GalNAcα-O-Ser/Thr. Patients with Schindler disease lack an α-N-acetylgalactosaminidase. This enzyme is specific for α-GalNAc and will not cleave α-GlcNAc. Patients lacking α-N-acetylgalactosaminidase accumulate GalNAc-containing glycopeptides in their urine, but curiously they also accumulate more complex, extended glycopeptides containing GlcNAc, galactose, and sialic acid. The structures are the same as those found on some native glycoconjugates. Their production may result from a general slowdown in degradation of the oligosaccharides, but this seems unlikely. Alternatively, the terminal GalNAcα-O-Ser/Thr glycopeptide could be generated as a normal degradation product using normal glycosidases and then rebuilt once again. How would this happen? There are several possibilities. One is that some of the partially graded glycopeptides enter a compartment (Golgi?) that contains the appropriate glycosyl transferases and sugar nucleotides. Monosaccharides are added sequentially before the products exit the cell. In this way, the glycopeptides would be behaving like oligosaccharide primers (see Chapter 40). However, another possibility is that the concentration of GalNAcα-O-Ser/Thr is high enough in the lysosome that lysosomal glycosidases use them as acceptors in a series of transglycosylation reactions. α-N-acetylgalactosaminidase has also been found to be part of the sialidase/β-galactosidase/cathepsinA complex mentioned above. If lysosomal glycosidases form complexes to degrade glycoconjugates more efficiently, partially degraded substrates may be in a preferred location to be acceptors in transglycosylation reactions. The same α-GalNAcase probably removes terminal α-GalNAc from blood-group-A-containing glycans (GalNAcα1,3Gal) and some glycolipids such as the Forsmann antigen, GalNAcα1,3GalNAcβ1,3Galα1,4Galβ1,4Glcβ-Cer.
Glycosaminoglycan Degradation (1,2,4,11–13)
The glycosaminoglycans, including heparan sulfate, chondroitin sulfate, dermatan sulfate, keratan sulfate, and hyaluronan, are degraded in a highly ordered fashion. The first three are O-xylose-linked to their core proteins, keratan sulfate can be both N- and O-linked depending on the tissue source, and hyaluronan is made as a free chain (see Chapter 11). Some proteoglycans are internalized from the cell surface, and the protein components are degraded. Then, the GAG chains are partially cleaved by enzymes such as endo-β-glucuronidases or endohexosaminidases that clip them at a few specific sites, probably depending on the sequence, generating approximately 10-kD fragments. This is probably important, because simple exoglycosidase digestion starting from one end would be very slow for such large chains. Endoglycosidase cleavage creates multiple terminal residues that can all be degraded by unique or overlapping sets of sulfatases and exoglycosidases. In general, an exoglycosidase will not degrade a substituted sugar, so these must first be removed. Structural analysis of partially degraded fragments in the lysosomes of cells from patients with a variety of genetic disorders called mucopolysaccharidoses (see Table 18.2) was critical to solving these degradation pathways. Again, note that there are a range of clinical severities and manifestations.
Hyaluronan
The most abundant GAG is HA, which is degraded in the lysosome by hyaluronidase (see Figure 18.2). The products are tetrasaccharides and larger fragments. Because HA is not synthesized on a protein, proteolysis is not required, and it is not sulfated; therefore, only β-glucuronidase and β-N-acetylhexosaminidase are needed for degradation, which occurs sequentially from the nonreducing end. Pig liver hyaluronidase has a sequence identical to that of the heme-binding serum protein hemopexin. Although serum hemopexin binds to HA, it does not appear to have hyaluronidase activity. Expression of the pig hyaluronidase enzyme in baculovirus produces a protein with hyaluronidase activity, showing that this primary sequence has the information needed for enzymatic activity. The relationship between lysosomal hyaluronidase and hemopexin is still unclear.

Figure 18.2
Degradation of hyaluronan. Hyaluronidase, an endoglycosidase, cleaves large chains into smaller fragments, each of which is then sequentially degraded from the nonreducing end.
Heparan Sulfate (14–15)
HS chains are first degraded by an endoglucuronidase followed by well-ordered sequential degradation (see Figure 18.3). A terminal IdoA-2-sulfate must be desulfated by a specific IdoA-2-sulfatase to make it a suitable substrate for α-iduronidase. If a GlcA-2-sulfate were at this position, a GlcA-2-sulfatase would first remove the sulfate, followed by β-glucuronidase cleavage. The terminal GlcNSO4 is the next sugar for cleavage, but this requires two steps. The sulfate is removed by N-sulfatase forming glucosamine, but this residue cannot be cleaved by α-N-acetylglucoaminidase. The amino group must be N-acetylated by an N-acetyl transferase embedded in the lysosomal membrane. In the first step, acetyl-CoA donates the acetyl group to a cytosolic-facing histidine residue at neutral pH. The acetyl group then becomes available on the luminal side of the lysosomal membrane and transferred at low pH to the amino group of glucosamine on the partially degraded HS chain. The terminal α-GlcNAc can now be cleaved. If the glucuronate residue is 2-sulfated, the sulfate is first removed, followed by β-glucuronidase cleavage. If the next α-GlcNAc is 6-O-sulfated, the sulfate is removed by a specific GlcNAc-6-sulfatase. Figure 18.3 and Table 18.2 provide a listing of the enzymatic defects and the disorders they cause.

Figure 18.3
Degradation of heparan sulfate. An endoglucuronidase first cleaves large chains into smaller fragments, and then each monosaccharide is removed from the nonreducing end as described in the text. N- and O-sulfate esters must first be removed before the (more...)
Dermatan Sulfate and Chondroitin Sulfate
A combination of endoglycosidases, sulfatases, and exoglycosidases degrade DS in the lysosome (Figure 18.4). Iduronate-2-sulfatase is followed by α-iduronidase. The terminal GalNAc-4-SO4 can be removed by either of two pathways. In the first pathway, a GalNAc-4-sulfate sulfatase acts, followed by β-N-acetylhexosaminidase A or B to remove GalNAc. In the second, β-N-acetylhexosaminidase A removes the entire GalNAc-4-SO4 unit followed by sulfatase cleavage. Absence of this sulfatase (MPS VI) is unique to the DS pathway. β-glucuronidase cleaves the β-GlcA residue and the process is repeated on the rest of the molecule.

Figure 18.4
Degradation of dermatan/chondroitin sulfate. Sequential degradation can proceed by two different routes. One route uses GalNAc-4-SO4ase followed by cleavage with β-N-acetylhexosaminidase A or B. The other route uses only β-N-acetylhexosaminidase (more...)
To degrade CS, GalNAc-6-SO4 sulfatase and GalNAc-4-SO4 sulfatase work in combination with β-N-acetylhexosaminidase A or B and β-glucuronidase. Hyaluronidase may also degrade CS, but no CS-specific endoglycosidases have been found.
Keratan Sulfate
KS is a heavily sulfated polylactosamine chain. Although some organisms have an endo-β-galactosidase that could cleave similar chains, mammalian cells do not have an endoglycosidase to break KS down. Sequential action of sulfatases and exoglycosidases is needed. Galactose-6-SO4 sulfatase is the same enzyme that desulfates GalNAc-6-sulfatase in CS degradation. This hydrolysis is followed by β-galactosidase digestion, leaving a terminal GlcNAc-6-SO4. Desulfation by the sulfatase followed by cleavage with β-N-acetylhexosaminidase A or B eliminates GlcNAc-6-SO4. Alternatively, β-N-acetylhexosaminidase A can directly release GlcNAc-6-SO4 followed by desulfation of the monosaccharide.
Degradation of the Linkage Regions of Proteoglycans
Degradation of the core region O-linked KS (skeletal type; type II) probably occurs by the same route as the other typical O-linked chains (Figure 18.5). The familiar N-linked corneal-type KS (type I) and the more recently discovered family of N-linked GAG chains are probably also degraded by the same set of enzymes used for N-linked degradation. The more typical xylose-linked GAG chains (DS, CS, HS) all share a common core tetrasaccharide. The finding of GlcAβ1,3Galβ1,3Galβ1,4Xylβ-O-Ser and xylose- and galactose-terminated core fragments suggests that endo-β-xylosidase and endo-β-galactosidase exist. An endo-β-xylosidase has been detected in the rabbit liver. It is possible that similar enzymes cleave different types of GAGs, but the details of how the linkage regions of GAGs are degraded remain to be established.

Figure 18.5
Stepwise lysosomal degradation of keratan sulfate. Sequential degradation of KS occurs from the nonreducing end, and like the degradation of DS and CS, the terminal GlcNAc-6-SO4 can be cleaved sequentially by a sulfatase and then by β-N-acetyl-hexosaminidase (more...)
Glycosphingolipid Degradation (1,16–18)
Glycosphingolipids (see Chapter 9) are degraded from the nonreducing ends by exoglycosidases while they are still bound to the lipid moiety, ceramide. Since glycosphingolipids have many of the same outer sugar sequences that are found in N- and O-glycans, many of the same glycosidases are used for their degradation (Figure 18.6). However, specialized hydrolases are needed for cleaving the glucose-ceramide and galactose-ceramide bonds and other linkages near the membrane. Besides these specific enzymes, additional noncatalytic sphingolipid activator proteins (some called saposins) help present the substrate to the enzyme for cleavage. Endoglycoceramidases have been reported in leech and earthworm and may be present in higher animals. This enzyme, like the peptide-N-glucoamidase, releases the entire glycan from the lipid.

Figure 18.6
Degradation of glycolipids. Required activator proteins are shown in parentheses.
Specialized Enzymes and Proteins for Glycosphingolipid Degradation
Some exoglycosidases for glycoprotein and GAG chain degradation also degrade glycolipids, but others are unique to glycolipid degradation. The absence of these unique enzymes causes glycolipid storage diseases listed in Table 18.3. Glucocerebrosidase, also called β-glucoceramidase, is specific for the degradation of the Glcβ-Cer bond, and its loss causes Gaucher's disease.
A specialized β-galactosidase, called β-galactoceramidase, hydrolyzes the bond between galactose and ceramide, and it can also cleave the terminal galactose from lactosyl ceramide. Its loss produces Krabbe disease. Galactosyl-ceramide is often found with a 3-sulfate ester (sulfatide), and thus a specific sulfatase, arylsulfatase A, is needed for its removal prior to β-galactosylceramidase action. Loss of this sulfatase causes metachromatic leukodystrophy and the accumulation of sulfatide. Glycolipids terminated with α-galactosamine residues are degraded by a specific α-galactosidase and its loss causes Fabry disease.
Activator Proteins
Two genes code for all of the known sphingolipid activator proteins. These proteins can also be used to form complexes with more than one degradative enzyme for more efficient hydrolysis.
GM2 Activator
GM2 activator protein forms a complex with either GM2 or GA2 and presents them to β-N-acetylhexosaminidase A for cleavage of terminal β-GalNAc. Sugars that lie too close to the lipid bilayer apparently have limited access to the soluble hexosaminidase. The activator protein binds a molecule of glycolipid, forming a soluble complex that can be cleaved by the hexosaminidase. The resulting product is now inserted back into the membrane, and the activator presents the next GM2 molecule, and so on. Genetic loss of this activator protein causes the accumulation of GM2 and GA2, resulting in the AB variant of GM2 gangliosidosis. The activator is probably targeted to the lysosomes via the Man-6-P pathway.
Saposins
Saposins are derived from a 524-amino-acid precursor called prosaposin which is processed into four homologous activator proteins, Sap-A, Sap-B, Sap-C, and Sap-D, each having about 80 amino acids and each having different properties. Sap-A and Sap-C both help β-glucosyl and β-galactosyl ceramidase degradation. Sap-B assists arylsulfatase A, α-galactosidase, α-sialidase, and β-galactosidase. The mechanism is thought to be similar to that of the GM2 activator by partially lifting a glycolipid molecule out of the membrane to form a complex that is cleaved by the soluble enzyme, followed by reinsertion of the substrate back into the membrane. Sap-D and Sap-B also assist in sphingomyelin degradation by sphingomyelinase. Complete absence of prosaposin is lethal in humans, and deficiencies in Sap-B and Sap-C lead to defects that clinically resemble aryl sulfatase A deficiency (metachromatic leukodystrophy) and Gaucher's disease. These symptoms might be predicted based on the enzyme that these saposins assist.
A very rare human disorder is the multiple sulfatase deficiency. All sulfatases are casualties of this defect, since they all require a posttranslational conversion of an active site cysteine residue to a 2-amino-3-oxopropionic acid for activity. In essence, the S group of cysteine is replaced by a double-bonded oxygen atom that probably acts as an acceptor for cleaved sulfate groups. Loss of the enzyme carrying out that reaction leads to inactive sulfatases.
Inhibition of Degradation (19–20)
There are several ways to prevent lysosomal degradation of glycoconjugates. One is by raising the intralysosomal pH in cells. Another is to inactivate a particular glycosidase genetically or to use a specific inhibitor. Although proteolysis inhibitors may also prevent efficient degradation, they will not totally block it.
General Inhibitors
Since nearly all lysosomal enzymes have acid pH optima, degradation can be severely curtailed by increasing the intralysosomal pH from 5–5.5 to 7, using agents such as ammonium chloride and chloroquine. These agents lower the rate of degradation, but they may not completely block it. Some lysosomal enzymes have relatively broad pH optima, and some of these enzymes actually have pH optima higher than the lysosome. It has been suggested that lysosomes may not always remain at low pH. Some “lysosomal” enzymes are actually present and active in early and late endosomes that have a higher pH than lysosomes.
Specific Inhibitors
Glycosylation inhibitors are covered in Chapter 40; however, some of these also inhibit specific lysosomal enzymes. For example, swainsonine blocks lysosomal α-mannosidase in addition to blocking the α-mannosidase II involved in glycoprotein processing. Sheep and cattle become neurologically deranged by eating food rich in swainsonine, which is also called locoweed. Since there are no neurological effects seen in transgenic knockout mice lacking the processing α-mannosidase II, it is likely that these temporary symptoms are induced because lysosomal α-mannosidase is inhibited. Undegraded oligosaccharides probably accumulate in affected animals. Although many inhibitors affect various enzymes when tested in in vitro enzymatic assays, they may not be effective in cells or whole animals because they may not enter the lysosome, or if they do, their concentration may not be sufficient.
Perspective on Synthesis and Degradation and Genetic Disorders
Genetic defects in lysosomal enzymes have provided the best insight into the catabolic pathways and their importance. For example, cocultivation of fibroblasts from patients with different storage diseases leads to the disappearance of stored material in both types of cells. Each supplies the corrective factors (lysosomal enzyme) that the other lacks through uptake via the Man-6-P receptors on the cell surface (for further details, see Chapter 23 on the discovery of Man-6-P targeting of lysosomal enzymes). Cross-correction highlights the fact that only a relatively small amount of enzyme may be needed to prevent accumulation of storage products and the complications they cause. Some estimates suggest that only 10% of normal β-N-acetylhexoaminidase activity may be sufficient to prevent pathological symptoms of Tay-Sachs disease. Since accumulation of stored material depends on the relative rates of glycoconjugate synthesis and degradation, the near absence of a glycosidase can be ameliorated by reducing the synthetic rate of the stored compound. This was cleverly tested in a mouse model of Tay-Sachs disease by using N-butyldeoxynojirimycin, an inhibitor of glucosylceramide synthase, the first step in glycosphingolipid biosynthesis. When normal mice were treated with this compound, the amount of glycosphingolipids fell 50–70% in all tissues without obvious pathological effects. When this compound was given to Tay-Sachs mice, the accumulation of GM2 in the brain was blocked and the amount of stored ganglioside was reduced. Thus, reducing the synthesis of the primary precursor reduced the load of GM2 that could be degraded by the mice.
As is discussed in Chapter 6, it is assumed that most of the monosaccharides derived from glycoconjugate breakdown are reutilized. The few studies exploring this issue suggest that reutilization of glycosidase-liberated sugars may be quite substantial.
Degradation and Resynthesis (21–22)
Degradation of glycoconjugates is not always complete. Partially degraded or incomplete glycans on glycoproteins and glycosphingolipids at the cell surface can be internalized and then elongated within a functional Golgi compartment containing the sugar nucleotide and the appropriate glycosyltransferase. In the case of glycosphingolipids, this pathway makes a substantial contribution to the total cellular synthesis, whereas in the case of glycoproteins, it probably makes a relatively small contribution. It is unknown whether these pathways are simply salvage and repair mechanisms or whether they play an integral part in an as yet unidentified physiological pathway.
Glycoprotein Reglycosylation
A variety of different experiments clearly show that the half-life of specific membrane proteins is longer than the half-life of their glycans. The more terminal monosaccharides turn over faster than those closer to the reducing end of the chain, suggesting that the monosaccharides are sequentially removed by exoglycosidases. This may occur at the cell surface or when proteins are endocytosed in the course of normal membrane recycling. Since the mildly acidic late endosomes contain lysosomal enzymes, whose pH optima can be quite broad, terminal sugars such as sialic acid can be cleaved. If the endocytosed proteins are not degraded in lysosomes, they may encounter sialyltransferases in the Golgi, become resialylated, and reappear again on the cell surface. A similar situation may occur if a glycoprotein has lost both sialic acid and galactose residues from its glycans. Some membrane proteins synthesized in the presence of oligosaccharide processing inhibitors can still reach the cell surface in an unprocessed form. Subsequent incubation in the absence of inhibitors leads to normal processing over time. The extent of processing and the kinetics depend on the protein and the cell line. Since Golgi enzymes are not distributed identically in all cells, it is difficult to make a general statement about how much “reprocessing” can occur. Most studies have monitored N-linked glycans, but membrane proteins with O-glycans show a similar response. Newly arrived plasma membrane proteins will acquire additional O-glycans at previously unoccupied glycosylation sites over time. Initiation of O-glycans is thought to occur in the cis-Golgi, but it is difficult to determine whether there is a small amount of O-GalNAc transferase that is active in a late (trans) Golgi compartment or whether the acceptor protein briefly encounters a high concentration of the glycosyltransferase in an early compartment.
Glycosphingolipid Recycling
The majority of the newly synthesized glucosyl ceramide arrives at the cell surface by a Golgi-independent cytosolic pathway. Some of this material, as well as Glc-Cer generated by partial degradation of more complex glycolipids, can recycle to the Golgi. There, like glycoproteins, they can serve as acceptors for synthesis of longer chains. In the lysosome, sphingosine and sphinganine are produced by complete degradation of glycosphingolipids, and reutilized. Together, these pathways, especially the latter one, may actually account for the majority of complex glycolipid synthesis in many cells. Depending on the physiological state of the cell and synthetic demands, the de novo pathway starting from serine and palmitoyl CoA may account for only 20–30% of the total synthesis. Shuttling the recycled components through and among the various organelles appears to involve vimentin intermediate filaments. Mechanisms and details of this process are presently lacking, but these studies offer a physiological function for glycolipid transfer proteins that were purified from brain preparations many years ago. For details of the biosynthetic pathway, see discussion in Chapter 9.
Future Directions
A recurring theme in this chapter is the fine balance between synthesis, degradation, and the identification of salvage pathways. Investigation of monosaccharide salvage pathways will be an area of future research and should reveal the importance of dietary contributions and recycling of degraded molecules to glycoconjugate synthesis. Lysosomal storage diseases have been treated by enzyme replacement therapy, such as in the case of Gaucher's disease, and gene replacement therapy is also a possibility. However, as mentioned above, an interesting alternative is to decrease the rate of synthesis of some glycans that contribute to the accumulation of stored material. This may lead to some improvement in patients' conditions.
References
- 1.
- Winchester B G. Lysosomal metabolism of glycoconjugates. Subcell. Biochem. 1996;27:191–238. [PubMed: 8993162]
- 2.
- Jourdian G W. Normal and pathological catabolism of glycoproteins. New Compr. Biochem. 1996;30:3–54.
- 3.
- Thomas G.H. and Beaudet A.L. 1995. Disorders of glycoprotein degradation and structure: α-Mannosidosis, β-mannosidosis, fucosidosis, sialidosis, aspartylglucosaminuria, and carbohydrate-deficient glycoprotein syndrome. In The metabolic and molecular bases of inherited diseases, 7th edition (ed. Scriver C.R. et al.), vol. II, pp. 2529–2562. McGraw-Hill, New York.
- 4.
- Michalski J -C. Normal and pathological catabolism of glycoproteins. New Compr. Biochem. 1996;30:55–97.
- 5.
- Mononen I, Fisher K J, Kaartinen V, Aronson N N Jr. Aspartylglycosaminuria: Protein chemistry and molecular biology of the most common lysosomal storage disorder of glycoprotein degradation. FASEB J. 1993;7:1247–1256. [PubMed: 8405810]
- 6.
- Cacan R, Verbert A. Free oligomannosides produced during the N-glycosylation process: Origin, intracellular trafficking and putative roles. Trends Glycosci. Glycotechnol. 1997;9:365–377.
- 7.
- Suzuki T, Kitajima K, Inoue S, Inoue Y. Occurrence and biological roles of “proximal glycanases” in animal cells. Glycobiology. 1994;4:777–789. [PubMed: 7734841]
- 8.
- de Virgilio M, Weninger H, Ivessa N E. Ubiquitination is required for the retro-translocation of a short-lived luminal endoplasmic reticulum glycoprotein to the cytosol for degradation by the proteasome. J. Biol. Chem. 1998;273:9734–9743. [PubMed: 9545309]
- 9.
- Desnick R.J. and Wang A.M. 1995. α-N-acetylgalactosaminidase deficiency: Schindler disease. In The metabolic and molecular bases of inherited diseases, 7th edition (ed. Scriver C.R. et al.), vol. II, pp. 2509–2528. McGraw-Hill, New York.
- 10.
- d'Azzo A., Andria G., Strisciuglio P., and Galjaard H. 1995. Galactosialidosis. In The metabolic and molecular bases of inherited diseases, 7th edition (ed. Scriver C.R. et al.), vol. II, pp. 2825–2838. McGraw-Hill, New York.
- 11.
- Neufeld E.F. and Muenzer J. 1995. The mucopolysaccharidoses. In The metabolic and molecular bases of inherited diseases, 7th edition (ed. Scriver C.R. et al.), vol. II, pp. 2465–2494. McGraw-Hill, New York.
- 12.
- Aronson N N Jr., Davidson E A. Catabolism of mucopolysaccharides by rat liver lysosomes in vivo. J. Biol. Chem. 1968;243:4494–4499. [PubMed: 4234724]
- 13.
- Aronson N N Jr., De Duve C. Digestive activity of lysosomes. II. The digestion of macromolecular carbohydrates by extracts of rat liver lysosomes. J. Biol. Chem. 1968;243:4564–4573. [PubMed: 5684010]
- 14.
- Bame K J, Rome L H. Acetyl-coenzyme A:α-glucosaminide N-acetyltransferase. Evidence for an active site histidine residue. J. Biol. Chem. 1986;261:10127–10132. [PubMed: 3733705]
- 15.
- Bame K J, Rome L H. Acetyl coenzyme A:α-glucosaminide N-acetyltransferase. Evidence for a transmembrane acetylation mechanism. J. Biol. Chem. 1985;260:11293–11299. [PubMed: 3897232]
- 16.
- Gravel R.A., Clarke J.T.R., Kaback M.M., Mahuran D., Sandhoff K., and Suzuki K. 1995. The GM2 gangliosides. In The metabolic and molecular bases of inherited diseases, 7th edition (ed. Scriver C.R. et al.), vol. II, pp. 2839–2879. McGraw-Hill, New York.
- 17.
- Sandhoff K., Harzer K., and Fürst W. 1995. Sphingolipid activator proteins. In The metabolic and molecular bases of inherited diseases, 7th edition (ed. Scriver C.R. et al.), vol. II, pp. 2427–2442. McGraw-Hill, New York.
- 18.
- Dierks T, Schmidt B, von Figura K. Conversion of cysteine to formylglycine: A protein modification in the endoplasmic reticulum. Proc. Natl. Acad. Sci. 1997;94:11963–11968. [PMC free article: PMC23670] [PubMed: 9342345]
- 19.
- Leinekugel P, Michel S, Conzelmann E, Sandhoff K. Quantitative correlation between the residual activity of β-hexosaminidase A and arylsulfatase A and the severity of the resulting lysosomal storage disease. Hum. Genet. 1992;88:513–523. [PubMed: 1348043]
- 20.
- Platt F M, Neises G R, Reinkensmeier G, Townsend M J, Perry V H, Proia R L, Winchester B, Dwek R A, Butters T D. Prevention of lysosomal storage in Tay-Sachs mice treated with N-butyldeoxynojirimycin. Science. 1997;276:428–431. [PubMed: 9103204]
- 21.
- Gillard B K, Clement R, Colucci-Guyon E, Babinet C, Schwarzmann G, Taki T, Kasama T, Marcus D M. Decreased synthesis of glycosphingolipids in cells lacking vimentin intermediate filaments. Exp. Cell Res. 1998;242:561–572. [PubMed: 9683542]
- 22.
- Gillard B K, Harrell R G, Marcus D M. Pathways of glycosphingolipid biosynthesis in SW13 cells in the presence and absence of vimentin intermediate filaments. Glycobiology. 1996;6:33–42. [PubMed: 8991507]
- Degradation and Turnover of Glycans - Essentials of GlycobiologyDegradation and Turnover of Glycans - Essentials of Glycobiology
- Homo sapiens stomatin (STOM), transcript variant 4, mRNAHomo sapiens stomatin (STOM), transcript variant 4, mRNAgi|1890333435|ref|NM_001270527.2|Nucleotide
- Other Classes of Golgi-derived Glycans - Essentials of GlycobiologyOther Classes of Golgi-derived Glycans - Essentials of Glycobiology
Your browsing activity is empty.
Activity recording is turned off.
See more...