NCBI Bookshelf. A service of the National Library of Medicine, National Institutes of Health.
Varki A, Cummings R, Esko J, et al., editors. Essentials of Glycobiology. Cold Spring Harbor (NY): Cold Spring Harbor Laboratory Press; 1999.
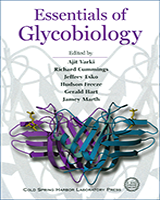
Essentials of Glycobiology.
Show detailsPrimary contributions to this chapter were made by J.D. Esko (University of California at San Diego).
INHIBITORS OF GLYCOSYLTRANSFERASES and glycosidases provide important tools for studying glycosylation in cells, tissues, and whole organisms. This chapter discusses various types of inhibitors, including natural products, substrate-based tight-binding inhibitors, glycoside primers, and one example of a rationally designed inhibitor. The use of these compounds for studying reaction chemistry and glycoconjugate biology and their use as potential therapeutics are also covered.
Introduction
Chapters 31–33 described various natural and induced mutants with defects in glycosylation. These genetic experiments have helped define genes encoding various transferases and glycosidases, and in some cases, alternate biosynthetic pathways have been uncovered. Mutants also provide insights into the function of glycosylation in cells and tissues as well as models for human in-born errors in metabolism and disease. However, one limitation of studying mutants is that the analyses are usually restricted to the cell or organism from which the mutant strain was isolated. In addition, many mutations are lethal in animals, which makes the study of the gene in adult animals more difficult.
Inhibitors provide another approach for studying glycosylation that avoids some of the problems associated with studying mutants. Many of these compounds are small molecules that are taken up readily by a variety of cell types, thus circumventing the cumbersome task of isolating similar mutants from different organisms or cells. Some inhibitors block glycosyltransferases (e.g., substrate analogs), whereas others act on processing enzymes (e.g., the alkaloids). Some act on central steps in metabolism (e.g., chlorate blocks the formation of PAPS) and therefore have pleiotropic effects on different pathways of glycan synthesis. Others are highly specific and affect only a single enzyme reaction (e.g., neuraminidase inhibitors). Some of the compounds can be absorbed through the gut, which provides an opportunity for designing drugs to treat human diseases and disorders correlated with altered glycosylation.
Glycosylation inhibitors arise naturally in plants, fungi, and bacteria, probably as part of a chemical defense strategy against other competing organisms in the same econiche. Other compounds have been synthesized based on the known substrate preferences for a particular enzyme or its X-ray crystal structure. In either case, inhibitors act as leads for producing additional analogs, often with increased activity or altered specificity.
This chapter describes several classes of inhibitors that act on glycosyltransferases and glycosidases in vertebrate cells (Table 40.1). Because the field is quite large, it is impossible to review all of the literature in the area. Therefore, only those inhibitors that act on specific enzymes or metabolic pathways and that illustrate certain basic concepts are discussed. Agents that block protein/carbohydrate interactions are discussed in Chapter 41 and elsewhere. Chapter 21 briefly discusses the aminoglycoside antibiotics.
Table 40.1
Classes of inhibitors.
Indirect Inhibitors and Metabolic Poisons (1–15)
A number of inhibitors have been described that block glycosylation by interfering with metabolism of common precursors or by interfering with intracellular transport activities. Some of these compounds act indirectly by interfering with the transit of proteins between the ER, Golgi, and trans-Golgi network (e.g., the fungal metabolite, Brefeldin A). Brefeldin A causes retrograde transport of Golgi components located proximal to the trans-Golgi network. Thus, treating cells with Brefeldin A separates enzymes located in the trans-Golgi network from those found in the ER and Golgi and uncouples the assembly of the core structures of some glycans from later reactions, such as sialylation or sulfation. In proteoglycan biosynthesis, Brefeldin A blocks the formation of chondroitin sulfate entirely but allows some residual heparan sulfate synthesis to continue, suggesting that the enzymes involved in heparan sulfate formation spread throughout the Golgi and the trans-Golgi network, whereas those involved in chondroitin synthesis are almost entirely located in the trans-Golgi network. This approach provides a snapshot of the particular system under study. Care should be taken in extrapolating the effects of Brefeldin A in one system to another, because the localization of the enzymes varies considerably in different cell types.
Some inhibitors act at key steps in intermediary metabolism where precursors involved in glycosylation are formed. For example, a glutamine analog, 6-diazo-5-oxo-l-norleucine, blocks glutamine:fructose-6-phosphate amidotransferase, the enzyme that forms glucosamine from fructose and glutamine (see Chapter 6). Depressing glucosamine production in this way has a pleiotropic effect on the assembly of glycans, since all of the major families of glycans contain GlcNAc or GalNAc. The effect of 6-diazo-5-oxo-l-norleucine on glycosylation can be partially reversed by feeding cells glucosamine or by the salvage of glucosamine from the turnover of glycoprotein and proteoglycans.
Chlorate is another type of general inhibitor that blocks sulfation. The chlorate anion (ClO42-) is an analog of sulfate (SO42-) and forms an abortive complex with the sulfurylase involved in the formation of phosphoadenosine-5′-phosphosulfate, the active sulfate donor for all known sulfation reactions. Thus, treating cells with chlorate (usually 10–30 mm) inhibits sulfation by more than 90%, but the effect is not specific for any particular class of glycan or sulfation reaction (e.g., tyrosine sulfation is also affected). The effect of chlorate can be overcome with inorganic sulfate, consistent with its being a competitive inhibitor.
A number of sugar analogs have been made with the hope that they might exhibit selective inhibition of glycosylation. 2-deoxyglucose and fluorinated analogs of sugars (3-deoxy-3-fluoro- and 4-deoxy-4-fluoroglucosamine, 2-deoxy-2-fluoro-glucose, and 2-deoxy-2-fluoro-mannose) inhibit glycoprotein biosynthesis as measured by incorporation of labeled [3H]glucosamine or [3H]fucose, but the mechanism underlying the inhibitory effect is unclear in many cases. Early studies of 2-deoxyglucose showed that the analog was converted to UDP-2-deoxyglucose as well as to GDP-2-deoxyglucose and dolichyl-P-2-deoxyglucose. Inhibition of glycoprotein formation occurs apparently due to accumulation of various dolichol oligosaccharides containing 2-deoxyglucose, which cannot be elongated or transferred to glycoproteins normally. Unfortunately, many of these compounds may have other effects as well due to their conversion into other reactants through intermediary metabolism.
Several of the glycosyltransferases will tolerate adducts appended to the donor nucleotide sugar. For example, one of the α3-fucosyltransferases will transfer a fucose analog on GDP containing bulky substituents (even another sugar!) at the C6 position. This observation makes it possible to modify the structure of oligosaccharides on the surface of a cell with purified enzymes and synthetic donors. Another approach consists of using mannosamine analogs in which an acyl, alkyl, or ketone group is covalently linked to the amino group in place of acetate (Figure 40.1). The mannosamine analogs are incorporated into the nucleotide precursor pools and end up in novel sialic acid analogs on cell surface glycoproteins and glycolipids. When ketone-containing derivatives are incorporated, the cell surface glycoconjugates become sensitive to reagents that react with carbonyl groups.

Figure 40.1
Mannosamine analogs. Several derivatives of mannosamine containing different functionalities linked to the amino group are taken up by cells, converted to the corresponding derivative of CMP-sialic acid, and transferred to glycoproteins and glycolipids. (more...)
Tunicamycin: Inhibition of DOL-PP-GlcNAc Assembly(4,6,16–22)
Tunicamycin was first identified in Streptomyces lysosuperificus, and related compounds were found later in other microorganisms. Tunicamycin derives its name from its antiviral activity, which was due to inhibition of viral coat (or “tunica”) formation. Tunicamycin belongs to a class of nucleoside antibiotics composed of uridine, an 11-carbon disaccharide called aminodeoxydialdose (tunicamine; Figure 40.2), and a fatty acid of variable length (13–17 carbons), branching, and unsaturation.

Figure 40.2
Structure of tunicamycin. Tunicamycin consists of uridine conjugated to the dialdose, tunicamine. The compound is part of a family of related agents that vary in the length, branching, and degree of unsaturation of the fatty acid amide (R) linked to tunicamine. (more...)
Tunicamycin inhibits N-glycosylation in eukaryotes by blocking the transfer of GlcNAc-1-P from UDP-GlcNAc to dolichyl-P (catalyzed by GlcNAc phosphototransferase, GPT), thereby decreasing dolichyl-PP-GlcNAc. Other GlcNAc transferase reactions are not inhibited (e.g., GlcNAcTI-V; see Chapters 7 and 16), but the transfer of GlcNAc-1-P to undecaprenyl-P and the formation of undecaprenyl-PP-MurNAc pentapeptide involved in bacterial peptidoglycan biosynthesis are sensitive to tunicamycin (see Chapter 21). Tunicamycin acts as a tight binding competitive inhibitor presumably because it resembles the donor nucleotide sugar. Indeed, the Ki value for tunicamycin is about 5 × 10-8 m, whereas the Km value for UDP-GlcNAc is approximately 3 × 10-6 m. Another compound called amphomycin inhibits mannosylphosphoryldolichol synthesis by forming a complex with dolichylmonophosphate. However, this compound is a lipopeptide and apparently forms complexes with the glycosyl-carrier lipid, preventing its participation in the enzymatic reaction.
The actual amount of tunicamycin needed to inhibit glycosylation varies in different cells (0.1–10 μg/ml), possibly due to variable uptake and culture conditions or differences in the level of expression of the phosphotransferase. Tunicamycin is cytotoxic to cells, and resistant mutants overproduce GPT. Similarly, transfection of cells with the cloned GPT confers resistance, suggesting that the variable dose of inhibitor required in different cells may reflect variation in enzyme levels.
Tunicamycin has been used extensively for studying the role of N-glycans in glycoprotein maturation, secretion, and function, as documented by more than 2500 papers citing its use since its first discovery in 1973. Recently, the drug was shown to induce apoptosis preferentially in cancer cells, presumably due to alterations in glycosylation of various cell surface receptors and signaling molecules. Although the mechanism underlying its apoptosis-inducing activity may be complex, the observation suggests the possibility of a chemotherapeutic treatment based on inhibiting glycosylation (see Chapter 35).
Plant Alkaloids: Natural Inhibitors of Glycosidases (4,6,23–32)
Plant alkaloids block glycosylation by inhibiting the processing glycosidases involved in N-glycan formation (Table 40.2). Unlike tunicamycin, which blocks glycosylation of glycoproteins entirely, the alkaloids inhibit the trimming reactions that occur after the Glc3Man9GlcNAc2 oligosaccharide is attached to a glycoprotein (see Chapter 7). Thus, the inhibitory alkaloids generally do not interfere with protein folding, but the glycoproteins that appear on the cell surface lack the characteristic termini found on mature oligosaccharides.
Table 40.2
Examples of alkaloids that inhibit glycosidases involved in N-linked glycan biosynthesis.
One class of alkaloids inhibits the α-glucosidases involved in the initial processing of the N-glycans and in quality control of protein folding (see Chapter 22). This class includes castanospermine (from the seed of the Australian chestnut tree, Castanosperum australe), which inhibits both α-glucosidases I and II; australine (also from C. australe), which preferentially inhibits α-glucosidase I; and deoxynojirimycin (from Streptomyces species), which preferentially inhibits α-glucosidase II. As expected, castanospermine and australine cause accumulation of fully glucosylated chains, whereas deoxynojirimycin results in chains containing one to two glucose residues. Treating cells with these inhibitors revealed that some trimming of the mannose residues can occur independently of removal of the glucose residues (see Chapter 7).
Swainsonine was first discovered in plants from the western United States (Astragalus species, aka locoweed) and Australia (Swainsona canescens), and it was later found in the fungus Rhizoctonia leguminocola that infects red clover. Consumption of these plants causes a severe abnormality called locoism, which includes accumulation of glycoproteins in the lymph nodes. Thus, swainsonine is part of a chemical defense strategy used by plants against grazing animals (and probably insects). Swainsonine inhibits α-mannosidase II, causing the accumulation of high-mannose oligosaccharides (Man4GlcNAc2 and Man5GlcNAc2) and hybrid-type chains at the expense of complex oligosaccharides. It also inhibits the lysosomal α-mannosidase. Mannostatin A works in a similar way, but differs significantly in structure from swainsonine (Table 40.2). Other mannosidase inhibitors include deoxymannojirimycin and kifunensin, which selectively inhibit α-mannosidase I. As expected, these agents cause the accumulation of Man7–9GlcNAc2 oligosaccharides on glycoproteins.
All of these inhibitors have in common polyhydroxylated ring systems that mimic the orientation of hydroxyl groups in the natural substrates, but a strict correlation between stereochemistry and enzyme target (α-glucosidase vs. α-mannosidase) does not exist. The compounds contain nitrogen, usually in place of the ring oxygen. One idea is that the nitrogen in the protonated state may mimic the positive charge on the ring oxygen that arises from delocalization of charge from the tentative carbocation at C1 generated during the hydrolysis reaction. Although no crystal structures for the processing glycosidases are available, the structure of the glucoamylase that removes glucose residues from starch has been solved with and without deoxynojirimycin in the active site. The crystal structure shows a glutamate residue that forms a salt bridge with the protonated form of the ring nitrogen. Thus, the relatively low pK of the amino group makes the substrate look like the transition state of the hydrolytic reaction.
Alkylated and acylated analogs of the alkaloids have been made and shown to have interesting and useful properties. N-butylation of deoxynojirimycin actually converts the glucosidase inhibitor into an inhibitor of glycolipid biosynthesis, but the mechanism of inhibition is unknown. Alkylation of the amino group or acylation of the hydroxyl groups can raise the potency of the compound, presumably by facilitating uptake across the plasma and Golgi membranes. Some of these compounds have shown positive effects as drugs for treating diabetes, cancer, and HIV infection. N-hydroxyethyl-deoxynojirimycin (Miglitol®) inhibits the disaccharidases in the intestine involved in digestion of sucrose and other dietary sugars, thus lowering the amount that is available for absorption and consequently decreasing blood sugar levels. Swainsonine and its carbonoyloxy analogs have potent antitumor activity in mice and humans, suggesting that these inhibitors may provide novel chemotherapeutic approaches for treating cancer.
Substrate Analogs: Directed Synthesis of Inhibitors (33–44)
A number of inhibitors have been developed based on the concept that substrate analogs might act as tight-binding inhibitors of specific transferases. Analogs of acceptors have the advantage that they should be more selective for specific enzymes than nucleotide sugar donors, which are used by multiple transferases. The general strategy is to modify the acceptor hydroxyl group or hydroxyls in the immediate vicinity (Table 40.3). About half of the compounds that have been made lack inhibitory activity, presumably because removal or alkylation of the targeted hydroxyl group prevents binding of the analog to the enzyme. In other cases, the analogs exhibit Ki values in the approximate range of the Km values for the parent substrate. As one might expect, the analogs usually act competitively with respect to the unmodified substrate, but in a few cases, the inhibition pattern was more complex, suggesting possible binding outside the active site.
Table 40.3
Synthetic substrate-based inhibitors of glycosyltransferases.
In addition to acceptor analogs, a number of nucleotide sugar derivatives have been made. Several of these exhibit strong inhibitory activity, but because they contain the negatively charged pyrophosphate linkage between the nucleoside and the sugar, they cannot cross the plasma membrane and therefore lack activity in vivo. “Bisubstrate” analogs consist of the nucleoside sugar donor covalently linked to the acceptor substrate by way of a neutral bridging group. This arrangement potentially generates inhibitors whose binding characteristics should reflect the product of the affinity constants for each substrate (approximated by the product of the individual Km values). The two bisubstrates that have been made have low Ki values (2–16 μm) in the range of the Km values for the nucleotide sugar donor, suggesting that the correct geometry for the bridging group may have not been attained. More analogs need to be made to determine if these compounds will have useful properties in vivo.
A certain amount of serendipity is needed to obtain active compounds using these synthetic approaches, and the synthesis of di-, tri-, and tetrasaccharides with the desired modifications is rather labor intensive. Nevertheless, the approach has yielded insights into the binding and reactivity of the enzymes, and substrate analogs with selectivity for particular enzymes have been developed in this way. Because many of the transferases have now been purified and cloned, we can look forward to more detailed kinetic and crystallographic studies, which will provide clues for deriving mechanism-based inhibitors in the future.
Glycoside Primers: Mimicking What Already Works (45–56)
The utility of any glycosyltransferase inhibitor ultimately depends on its ability to cross the plasma membrane and enter the Golgi where the glycosyltransferases reside. Unfortunately, many of the compounds described above lack activity in live cells, presumably because their polarity prevents their uptake. Okayama et al. 25 years ago found that d-xylose in β-linkage to a hydrophobic aglycone was taken up rather efficiently and inhibited the assembly of glycosaminoglycans on proteoglycans. Inhibition was caused by “priming” of chains on the added xyloside, which diverted the assembly process from the endogenous core proteins. In general, cells incubated with xylosides secrete large amounts of individual glycosaminoglycan chains and accumulate proteoglycans containing truncated chains. The enormous success of β-d xylosides in altering proteoglycan biosynthesis suggested that other glycosides might act as “primers” as well (Table 40.4). Subsequent studies have shown that α-N-acetylgalactosaminides prime oligosaccharides found on mucins and inhibit O-glycosylation of glycoproteins. Other active glycosides include β-glucosides, β-galactosides, β-N-acetylglucosaminides, and even disaccharides and trisaccharides. These compounds require conjugation to appropriate aglycones and acetylation to neutralize the polar hydroxyl groups on the sugars. Cells contain several carboxylesterases that remove the acetyl groups. Apparently this can occur in a way that makes the compounds available to the transferases in the Golgi.
Table 40.4
Examples of glycoside primers.
Priming by glycosides occurs in a concentration-dependent manner, but the efficiency varies widely among different compounds and cell types. These variations may relate to the relative abundance of endogenous substrates, enzyme concentration and composition, the solubility of different glycosides, their susceptibility to hydrolysis, their uptake across the plasma membrane and into the Golgi, and their relative affinity for the glycosyltransferases. The type of chain made on a given primer also depends on concentration and aglycone structure, which may reflect selective partitioning of primers into different intracellular compartments or into different branches of biosynthetic pathways.
Glycoside primers act as inhibitors of glycoprotein and proteoglycan assembly by subverting the cellular machinery for making oligosaccharides on endogenous proteins. Like priming, inhibition occurs in a dose-dependent fashion, but the blockade is rarely complete, probably due to the inability of glycosides to completely mimic the endogenous core glycoprotein substrates. It is important to remember that (1) glycosides inhibit the formation of entire classes of glycoconjugates, but the effects on individual proteins may vary, and (2) inhibition occurs in the midst of priming. The primed material may also have a composition different from that of the oligosaccharides produced on core proteins. Thus, any effect on a biological phenomenon may be due to the decline in glycoprotein or proteoglycan assembly or the increase in free oligosaccharide chains. (3) The possibility should always be considered that the enhanced rate of synthesis of oligosaccharides on primers may deplete pools of nucleotide sugars and thus alter the assembly of other classes of glycoconjugates besides those that are generated on the primer. For example, xylosides will prime compounds resembling intermediates in glycosaminoglycan biosynthesis, an unusual compound containing GalNAc in α-linkage to the tetrasaccharide core found in chondroitin sulfate and heparan sulfate, a compound related to ganglioside GM3, Xylβ4Xyl-R, GlcA(3S)Xyl-R, in addition to free glycosaminoglycan chains. Although this lack of specificity may seem to be a disadvantage, the products that have been found have revealed previously undescribed pathways in cells.
Primers represent starting points for making analogs that might have the properties of tight-binding inhibitors described in the previous section. Many of the compounds described in Table 40.3 could be converted to permeable acylated glycosides and tested in live cells for inhibitory activity. Active compounds might have the potential of becoming carbohydrate-based drugs for treating diseases and disorders dependent on glycosylation.
Priming of oligosaccharides may have beneficial effects as well. Xylosides, for example, can be absorbed through the gut, and when consumed at sufficient concentration, they exhibit antithrombotic activity. Many glycosides occur naturally, since various organisms (especially plants) produce hydrophobic compounds as part of chemical defense and conjugate them to sugars in order to render them soluble. Thus, the human diet may contain various types of glycosides with interesting (and unknown) biological activities.
Inhibitors of Glycolipids and GPI Anchors (57–62)
Reagents have been described that can alter the assembly of glycolipids in cells. As mentioned above, xylosides have a mild effect on glycolipid formation, possibly due to the assembly of a GM3-like compound (Neu5Acα3Galβ4Xylβ-O-R) on the primer. In glycolipid biosynthesis, many of the intermediates behave like the synthetic glycosides described above in the sense that cells will take them up and use them as primers. Glucosylceramide is a naturally occurring intermediate, and it will give rise to more complex glycolipids when fed to cells. On the basis of this observation, an analog containing a reactive exocyclic epoxide group was prepared. The compound inhibits glycolipid formation (IC50 ~8 μm), presumably by reaction of the epoxide with a nucleophile in the active site of lactosylceramide synthase.
As mentioned above, the α-glucosidase inhibitor, N-butyldeoxynojirimycin, has been used to inhibit glycosphingolipid formation because it inhibits glucosylceramide synthesis. However, the sphingolipid analogs shown in Figure 40.3 are more potent inhibitors. Lengthening the hydrocarbon chain from 10 to 16 carbons further enhances efficacy. These compounds can cause almost complete depletion of glycolipids in cells, and they require only micromolar amounts for activity.

Figure 40.3
Inhibitors of glycosphingolipid formation. These commercially available analogs are potent inhibitors of the glucosyltransferase that initiates glycosphingolipid formation.
Inhibitors of GPI anchor formation have also been described. Mannosamine inhibits GPI anchor formation both in Trypanosoma brucei and in mammalian cells by formation of ManNH2-Man-GlcN-PI. Apparently, mannosamine in its activated form (GDP-ManNH2) is used as an analog of mannose in the second mannosyltransferase reaction, but the ManNH2-Man-GlcN-PI intermediate will not act as a substrate for the next α2 mannosyltransferase (see Chapter 10). Another class of inhibitors is based on fatty acid analogs that only trypanosomes incorporate into GPI anchors. Trypanosomes, unlike their mammalian hosts, incorporate myristic acid into GPI anchors by exchanging myristic acid for other fatty acids in the phosphatidylinositol moiety. By making a series of heteroatom-containing analogs, one was found that was highly toxic to trypanosomes in culture and nontoxic to mammalian cells (10-[propoxy]decanoic acid). A reagent such as this has the potential of becoming a drug for treating trypanosomiasis, which is endemic in sub-Saharan regions of Africa.
Neuraminidase Inhibitors: Rational Design from X-ray Crystal Structures (63–66)
Studies of the influenza neuraminidase exemplify the power of rationally designed drugs. The crystal structure for influenza neuraminidase was obtained in 1983, and since then many other sialidases have been characterized from other sources. Even before the crystal structure had been obtained, an inhibitor for neuraminidase was deduced by assuming that the hydrolysis reaction probably involved a transition state with a carbocation intermediate at C2. This would result in C2 and C3 adopting a trigonal planar (sp2) configuration, and therefore compounds that mimicked this geometry might have inhibitory activity. Indeed, Neu5Ac-2-ene (Figure 40.4) has a micromolar Ki value. Interestingly, this compound works on most sialidases, but not on the trypanosome trans-sialidase, and only weakly on bacterial sialidases.

Figure 40.4
Structure of influenza neuraminidase inhibitors. The analog Neu5Ac-2-ene is thought to resemble the transition state in hydrolysis, and the addition of the guanidinium group permits higher-affinity binding to the active site.
Visual inspection of the influenza enzyme with the inhibitor bound showed that two glutamate residues lined a pocket near O4 of the sialic acid residue and formed hydrogen bonds with O4 (Figure 40.5). The pocket was fairly open, suggesting that a bulkier substituent at this position might be tolerated, at least sterically. A substrate analog was produced containing a positively charged guanidinium group instead of O4, and binding studies showed that the analog had a Ki value of 10-11 m (Figure 40.4). The higher affinity was presumably due to an additional salt bridge formed between the charged guanidinium group and the carboxylates lining the pocket. Interestingly, the analog does not work on the bacterial enzyme because the pocket is filled with an arginine group and only one aspartate residue lines the pocket.

Figure 40.5
Binding site for sialic acid in influenza neuraminidase. The crystal structure showed the orientation of active-site residues of the enzyme that interact with the substrate. The O4 pocket is at the top of the figure. The two conserved glutamate residues (more...)
Subsequent studies have attempted to use noncarbohydrate analogs based on the similarity of benzoic acid to the partially planar ring of the proposed intermediate in hydrolysis. Reasonably good inhibitors were found in this way, as long as hydrogen bond donors and acceptors were added to the ring. The best inhibitor contained a guanidinium group in the same position relative to the carboxylate anion, but the crystal data showed that the analog actually bound to the enzyme in an orientation very different from that of sialic acid. As the crystal structures for other sialidases are solved, the design of species-specific analogs may be possible. This rational approach for making inhibitors has great potential not only for neuraminidase inhibitors, but also for the design of compounds that might block the activity of various glycosyltransferases.
Future Directions
The various types of inhibitors described in this chapter were generated with the hope of studying the mechanism of action of individual enzymes and as probes to alter glycosylation in cells and tissues. Progress in this area has been modest in comparison with the development of inhibitors for other metabolic pathways. In part, this reflects the relatively recent cloning of many of the transferases, the sparse information on reaction mechanisms, and inherent difficulties in preparing carbohydrate-based analogs. Nevertheless, the available agents have been useful tools, and second-generation derivatives have had unusual properties that may prove of therapeutic value. As more crystal structures for glycosyltransferases emerge, improvements in inhibitor design and action should be forthcoming, which in turn should lead to compounds with greater specificity. Armed with enzyme-specific inhibitors, glycobiologists will be able to explore the biological function of the individual enzymes in normal and pathophysiology.
References
- 1.
- Ellis D B, Sommar K M. Biosynthesis of respiratory tract mucins. II. Control of hexosamine metabolism by l-glutamine: d-fructose 6-phosphate aminotransferase. Biochim. Biophys. Acta. 1972;276:105–112. [PubMed: 5047698]
- 2.
- Trujillo J L, Gan J C. Glycoprotein biosynthesis. VI. Regulation of uridine diphosphate N-acetyl-D-glucosamine metabolism in bovine thyroid gland slices. Biochim. Biophys. Acta. 1973;304:32–41. [PubMed: 4699997]
- 3.
- Schwarz R T, Datema R. The lipid pathway of protein glycosylation and its inhibitors: The biological significance of protein-bound carbohydrates. Adv. Carbohydr. Chem. Biochem. 1982;40:287–379. [PubMed: 6188345]
- 4.
- Elbein A D. Inhibitors of the biosynthesis and processing of N-linked oligosaccharides. CRC Crit. Rev. Biochem. 1984;16:21–49. [PubMed: 6232113]
- 5.
- Baeuerle P A, Huttner W B. Chlorate—A potent inhibitor of protein sulfation in intact cells. Biochem. Biophys. Res. Commun. 1986;141:870–877. [PubMed: 3026396]
- 6.
- Elbein A D. Inhibitors of the biosynthesis and processing of N-linked oligosaccharide chains. Annu. Rev. Biochem. 1987;56:497–534. [PubMed: 3304143]
- 7.
- Iozzo R V, Clark C C. Modulation of heparan sulfate biosynthesis. Effects of 6-diazo-5-oxo-L-norleucine and low glutamine on the synthesis of heparan sulfate proteoglycan by human colon carcinoma cells. J. Biol. Chem. 1987;262:11188–11199. [PubMed: 2956263]
- 8.
- Humphries D E, Silbert J E. Chlorate: A reversible inhibitor of proteoglycan sulfation. Biochem. Biophys. Res. Commun. 1988;154:365–371. [PubMed: 2969240]
- 9.
- van Echten G, Iber H, Stotz H, Takatsuki A, Sandhoff K. Uncoupling of ganglioside biosynthesis by Brefeldin A. Eur J. Cell Biol. 1990;51:135–139. [PubMed: 2328734]
- 10.
- Spiro R C, Freeze H H, Sampath D, Garcia J A. Uncoupling of chondroitin sulfate glycosaminoglycan synthesis by Brefeldin A. J Cell Biol. 1991;115:1463–1473. [PMC free article: PMC2289244] [PubMed: 1955486]
- 11.
- Sampath D, Varki A, Freeze H H. The spectrum of incomplete N-linked oligosaccharides synthesized by endothelial cells in the presence of Brefeldin A. J Biol. Chem. 1992;267:4440–4455. [PubMed: 1537830]
- 12.
- Srivastava G, Kaur K J, Hindsgaul O, Palcic M M. Enzymatic transfer of a preassembled trisaccharide antigen to cell surfaces using a fucosyltransferase. J. Biol. Chem. 1992;267:22356–22361. [PubMed: 1429589]
- 13.
- Uhlin-Hansen L, Yanagishita M. Differential effect of Brefeldin A on the biosynthesis of heparan sulfate and chondroitin/dermatan sulfate proteoglycans in rat ovarian granulosa cells in culture. J. Biol. Chem. 1993;268:17370–17376. [PubMed: 8349620]
- 14.
- Woynarowska B, Skrincosky D M, Haag A, Sharma M, Matta K, Bernacki R J. Inhibition of lectin-mediated ovarian tumor cell adhesion by sugar analogs. J. Biol. Chem. 1994;269:22797–22803. [PubMed: 8077232]
- 15.
- Mahal L K, Yarema K J, Bertozzi C R. Engineering chemical reactivity on cell surfaces through oligosaccharide biosynthesis. Science. 1997;276:1125–1128. [PubMed: 9173543]
- 16.
- Takatsuki A, Tamura G. Tunicamycin, a new antibiotic. 3. Reversal of the antiviral activity of tunicamycin by aminosugars and their derivatives. J. Antibiot. 1971;24:232–238. [PubMed: 5103535]
- 17.
- Takatsuki A, Arima K, Tamura G. Tunicamycin, a new antibiotic. I. Isolation and characterization of tunicamycin. J. Antibiot. (Tokyo). 1971;24:15–223. [PubMed: 5572750]
- 18.
- Bodanszky M, Sigler G F, Bodanszky A. Structure of the peptide antibiotic amphomycin. J. Am. Chem. Soc. 1973;95:2352–2357. [PubMed: 4709239]
- 19.
- McDowell W, Schwarz R T. Dissecting glycoprotein biosynthesis by the use of specific inhibitors. Biochimie. 1988;70:1535–1549. [PMC free article: PMC7126144] [PubMed: 3149521]
- 20.
- Zhu X, Zeng Y, Lehrman M A. Evidence that the hamster tunicamycin resistance gene encodes UDP-GlcNAc:dolichol phosphate N-acetylglucosamine-1-phosphate transferase. J. Biol. Chem. 1992;267:8895–8902. [PubMed: 1315744]
- 21.
- Zeng Y C, Elbein A D. UDP-N-acetylglucosamine:dolichyl-phosphate N-acetylglucosamine-1-phosphate transferase is amplified in tunicamycin-resistant soybean cells. Eur. J. Biochem. 1995;233:458–466. [PubMed: 7588788]
- 22.
- Dricu A, Carlberg M, Wang M, Larsson O. Inhibition of N-linked glycosylation using tunicamycin causes cell death in malignant cells: Role of down-regulation of the insulin like growth factor 1 receptor in induction of apoptosis. Cancer Res. 1997;57:543–548. [PubMed: 9012488]
- 23.
- Humphries M J, Matsumoto K, White S L, Olden K. Inhibition of experimental metastasis by castanospermine in mice: Blockage of two distinct stages of tumor colonization by oligosaccharide processing inhibitors. Cancer Res. 1986;46:5215–5222. [PubMed: 3093061]
- 24.
- Elbein A D. Glycosidase inhibitors: Inhibitors of N-linked oligosaccharide processing. FASEB J. 1991;5:3055–3063. [PubMed: 1743438]
- 25.
- Winchester B, Fleet G W. Amino-sugar glycosidase inhibitors: Versatile tools for glycobiologists. Glycobiology. 1992;2:199–210. [PubMed: 1498417]
- 26.
- Dennis J W, White S L, Freer A M, Dime D. Carbonoyloxy analogs of the anti-metastatic drug swainsonine. Activation in tumor cells by esterases. Biochem. Pharmacol. 1993;46:1459–1466. [PubMed: 8240396]
- 27.
- Harris E M, Aleshin A E, Firsov L M, Honzatko R B. Refined structure for the complex of 1-deoxynojirimycin with glucoamylase from Aspergillus awamori var. X100 to 2.4-Å resolution. Biochemistry. 1993;32:1618–1626. [PubMed: 8431441]
- 28.
- Goss P E, Baptiste J, Fernandes B, Baker M, Dennis J W. A phase I study of swainsonine in patients with advanced malignancies. Cancer Res. 1994;54:1450–1457. [PubMed: 8137247]
- 29.
- Jacob G S. Glycosylation inhibitors in biology and medicine. Curr. Opin. Struct. Biol. 1995;5:605–611. [PubMed: 8574695]
- 30.
- Kang M S, Liu P S, Bernotas R C, Harry B S, Sunkara P S. Castanospermine analogues: Their inhibition of glycoprotein processing α-glucosidases from porcine kidney and B16F10 cells. Glycobiology. 1995;5:147–152. [PubMed: 7772863]
- 31.
- Asano N, Kato A, Matsui K, Watson A A, Nash R J, Molyneux R J, Hackett L, Topping J, Winchester B. The effects of calystegines isolated from edible fruits and vegetables on mammalian liver glycosidases. Glycobiology. 1997;7:1085–1088. [PubMed: 9455909]
- 32.
- Platt F M, Reinkensmeier G, Dwek R A, Butters T D. Extensive glycosphingolipid depletion in the liver and lymphoid organs of mice treated with N-butyldeoxynojirimycin. J. Biol. Chem. 1997;272:19365–19372. [PubMed: 9235935]
- 33.
- Palcic M M, Heerze L D, Srivastava O P, Hindsgaul O. A bisubstrate analog inhibitor for α(1—2)-fucosyltransferase. J. Biol. Chem. 1989;264:17174–17181. [PubMed: 2551897]
- 34.
- Hindsgaul O, Kaur K J, Srivastava G, Blaszczyk-Thurin M, Crawley S C, Heerze L D, Palcic M M. Evaluation of deoxygenated oligosaccharide acceptor analogs as specific inhibitors of glycosyltransferases. J. Biol. Chem. 1991;266:17858–17862. [PubMed: 1917926]
- 35.
- Kajihara Y, Hashimoto H, Kodama H. Methyl-3-O-(2-acetamido-2-deoxy-6-thio-β-d-glucopyranosyl)-β-d- galactopyranoside: A slow reacting acceptor-analogue which inhibits glycosylation by UDP-d-galactose-N-acetyl-d-glucosamine-(1—4)-β-d-galactosyltransferase. Carbohydr. Res. 1992;229:C5–C9. [PubMed: 1516098]
- 36.
- Kajihara Y, Kodama H, Wakabayashi T, Sato K, Hashimoto H. Characterization of inhibitory activities and binding mode of synthetic 6′-modified methyl N-acetyl-β-lactosaminide toward rat liver CMP-D-Neu5Ac: d-galactoside-(2→6)-α-d-sialyltransferase. Carbohydr. Res. 1993;247:179–193. [PubMed: 8221719]
- 37.
- Khan S H, Crawley S C, Kanie O, Hindsgaul O. A trisaccharide acceptor analog for N-acetylglucosaminyltransferase V which binds to the enzyme but sterically precludes the transfer reaction. J. Biol. Chem. 1993;268:2468–2473. [PubMed: 8428922]
- 38.
- Lowary T L, Hindsgaul O. Recognition of synthetic deoxy and deoxyfluoro analogs of the acceptor α-l-Fuc p-(1→2)-β-d-Gal p-OR by the blood-group A and B gene-specified glycosyltransferases. Carbohydr. Res. 1993;249:163–195. [PubMed: 8252553]
- 39.
- Lowary T L, Hindsgaul O. Recognition of synthetic O-methyl, epimeric, and amino analogues of the acceptor α-l-Fuc p-(1→2)-β-d-Gal p-OR by the blood-group A and B gene-specified glycosyltransferases. Carbohydr. Res. 1994;251:33–67. [PubMed: 8149379]
- 40.
- Helland A C, Hindsgaul O, Palcic M M, Stults C L, Macher B A. Methyl 3-amino-3-deoxy-β-d-galactopyranosyl-(1→4)-2-acetamido-2-deoxy-β-d-glucopyranoside: An inhibitor of UDP-D-galactose: β-d-galactopyranosyl-(1→4)-2-acetamido-2-deoxy-d-glucose (1→3)-α-d-galactopyranosyltransferase. Carbohydr. Res. 1995;276:91–98. [PubMed: 8536260]
- 41.
- Reck F, Springer M, Meinjohanns E, Paulsen H, Brockhausen I, Schachter H. Synthetic substrate analogues for UDP-GlcNAc: Manα1-3R β1-2-N-acetylglucosaminyltransferase. 1. Substrate specificity and inhibitors for the enzyme. Glycoconj. J. 1995;12:747–754. [PubMed: 8748150]
- 42.
- Hashimoto H, Endo T, Kajihara Y. Synthesis of the first tricomponent bisubstrate analogue that exhibits potent inhibition against GlcNAc:β-1,4-galactosyltransferase. J. Org. Chem. 1997;62:1914–1915. [PubMed: 11671487]
- 43.
- Lu P P, Hindsgaul O, Li H, Palcic M M. Synthesis and evaluation of eight aminodeoxy trisaccharide inhibitors for N-acetylglucosaminyltransferase-V. Carbohydr Res. 1997;303:283–291. [PubMed: 9373934]
- 44.
- Murray B W, Wittmann V, Burkart M D, Hung S C, Wong C H. Mechanism of human α-1,3-fucosyltransferase V: Glycosidic cleavage occurs prior to nucleophilic attack. Biochemistry. 1997;36:823–831. [PubMed: 9020780]
- 45.
- Okayama M, Kimata K, Suzuki S. The influence of p-nitrophenyl β-d-xyloside on the synthesis of proteochondroitin sulfate by slices of embryonic chick cartilage. J. Biochem. 1973;74:1069–1073. [PubMed: 4770369]
- 46.
- Robinson H C, Brett M J, Tralaggan P J, Lowther D A, Okayama M. The effect of d-xylose, β-d-xylosides, and β-d-galactosides on chondrotin sulphate biosynthesis in embryonic chicken cartilage. Biochem. J. 1975;148:25–34. [PMC free article: PMC1165502] [PubMed: 1156397]
- 47.
- Kuan S F, Byrd J C, Basbaum C, Kim Y S. Inhibition of mucin glycosylation by aryl-N-acetyl-α-galactosaminides in human colon cancer cells. J. Biol. Chem. 1989;264:19271–19277. [PubMed: 2509474]
- 48.
- Zhuang D, Grey A, Harris-Brandts M, Higgins E, Kashem M A, Dennis J W. Characterization of O-linked oligosaccharide biosynthesis in cultured cells using paranitrophenyl α-d-GalNAc as an acceptor. Glycobiology. 1991;1:425–433. [PubMed: 1820202]
- 49.
- Fritz T A, Lugemwa F N, Sarkar A K, Esko J D. Biosynthesis of heparan sulfate on β-d-xylosides depends on aglycone structure. J. Biol. Chem. 1994;269:300–307. [PubMed: 8276811]
- 50.
- Neville D C A, Field R A, Ferguson M A J. Hydrophobic glycosides of N-acetylglucosamine can act as primers for polylactosamine synthesis and can affect glycolipid synthesis in vivo. Biochem. J. 1995;307:791–797. [PMC free article: PMC1136719] [PubMed: 7741710]
- 51.
- Freeze H H, Etchison J R. A new side of xylosides and their close relatives: Co-localization mapping glycosyltransferases in the functional Golgi. Trends Glycosci. Glycotechnol. 1996;8:65–77.
- 52.
- Martin N B, Masson P, Sepulchre C, Theveniaux J, Millet J, Bellamy F. Pharmacologic and biochemical profiles of new venous antithrombotic β-d-xyloside derivatives: Potential antiathero/thrombotic drugs. Semin. Thromb. Hemost. 1996;22:247–254. [PubMed: 8836009]
- 53.
- Pörtner A, Etchison J R, Sampath D, Freeze H H. Human melanoma and Chinese hamster ovary cells galactosylate n-alkyl-β-glucosides using UDP gal:GlcNAc β1,4 galactosyltransferase. Glycobiology. 1996;6:7–13. [PubMed: 8991512]
- 54.
- Miura Y, Yamagata T. Glycosylation of lactosylceramide analogs in animal cells: Amphipathic disaccharide primers for glycosphingolipid synthesis. Biochem. Biophys. Res. Commun. 1997;241:698–703. [PubMed: 9434771]
- 55.
- Sarkar A K, Rostand K S, Jain R K, Matta K L, Esko J D. Fucosylation of disaccharide precursors of sialyl LewisX inhibit selectin-mediated cell adhesion. J. Biol. Chem. 1997;272:25608–25616. [PubMed: 9325281]
- 56.
- Taylor W H, Sinha A, Khan I, McDaniel S T, Esko J D. Primers of glycosaminoglycan biosynthesis from Peruvian rainforest plants. J. Biol. Chem. 1998;273:22260–22266. [PubMed: 9712841]
- 57.
- Vunnam R R, Radin N S. Analogs of ceramide that inhibit glucocerebroside synthetase in mouse brain. Chem. Phys. Lipids. 1980;26:265–278. [PubMed: 6445239]
- 58.
- Doering T L, Raper J, Buxbaum L U, Adams S P, Gordon J I, Hart G W, Englund P T. An analog of myristic acid with selective toxicity for African trypanosomes. Science. 1991;252:1851–1854. [PubMed: 1829548]
- 59.
- Pan Y T, Kamitani T, Bhuvaneswaran C, Hallaq Y, Warren C D, Yeh E T, Elbein A D. Inhibition of glycosylphosphatidylinositol anchor formation by mannosamine. J. Biol. Chem. 1992;267:21250–21255. [PubMed: 1400435]
- 60.
- Freeze H H, Sampath D, Varki A. α-and β-xylosides alter glycolipid synthesis in human melanoma and Chinese hamster ovary cells. J. Biol. Chem. 1993;268:1618–1627. [PubMed: 8420936]
- 61.
- Zacharias C, van Echten-Deckert G, Plewe M, Schmidt R R, Sandhoff K. A truncated epoxy-glucosylceramide uncouples glycosphingolipid biosynthesis by decreasing lactosylceramide synthase activity. J. Biol. Chem. 1994;269:13313–13317. [PubMed: 8175761]
- 62.
- Abe A, Radin N S, Shayman J A, Wotring L L, Zipkin R E, Sivakumar R, Ruggieri J M, Carson K G, Ganem B. Structural and stereochemical studies of potent inhibitors of glucosylceramide synthase and tumor cell growth. J. Lipid. Res. 1995;36:611–621. [PubMed: 7775872]
- 63.
- von Itzstein M, Wu W Y, Kok G B, Pegg M S, Dyason J C, Jin B, Van Phan T, Smythe M L, White H F, Oliver S W, Colman P M, Varghese J N, Ryan D M, Woods J M, Bethell R C, Hotham V J, Cameron J M, Penn C R. Rational design of potent sialidase-based inhibitors of influenza virus replication. Nature. 1993;363:418–423. [PubMed: 8502295]
- 64.
- Taylor G. Sialidases: Structures, biological significance and therapeutic potential. Curr. Opin. Struct. Biol. 1996;6:830–837. [PubMed: 8994884]
- 65.
- von Itzstein M, Colman P. Design and synthesis of carbohydrate-based inhibitors of protein-carbohydrate interactions. Curr. Opin. Struct. Biol. 1996;6:703–709. [PubMed: 8913694]
- 66.
- Chand P, Babu Y S, Bantia S, Chu N, Cole L B, Kotian P L, Laver W G, Montgomery J A, Pathak V P, Petty S L, Shrout D P, Walsh D A, Walsh G M. Design and synthesis of benzoic acid derivatives as influenza neuraminidase inhibitors using structure-based drug design. J. Med. Chem. 1997;40:4030–4052. [PubMed: 9406595]
- Introduction
- Indirect Inhibitors and Metabolic Poisons
- Tunicamycin: Inhibition of DOL-PP-GlcNAc Assembly
- Plant Alkaloids: Natural Inhibitors of Glycosidases
- Substrate Analogs: Directed Synthesis of Inhibitors
- Glycoside Primers: Mimicking What Already Works
- Inhibitors of Glycolipids and GPI Anchors
- Neuraminidase Inhibitors: Rational Design from X-ray Crystal Structures
- Future Directions
- References
- Natural and Synthetic Inhibitors of Glycosylation - Essentials of GlycobiologyNatural and Synthetic Inhibitors of Glycosylation - Essentials of Glycobiology
- glycosyl transferase [Acinetobacter nosocomialis M2]glycosyl transferase [Acinetobacter nosocomialis M2]gi|1644143974|gnl|PRJNA541289|FDQ49 5|gb|QCP64608.1|Protein
- hypothetical protein B7L44_03805 [Acinetobacter nosocomialis]hypothetical protein B7L44_03805 [Acinetobacter nosocomialis]gi|1175719481|gnl|PRJNA380857|B7L44 5|gb|ARG15801.1|Protein
- integrase [Streptococcus pneumoniae]integrase [Streptococcus pneumoniae]gi|1314833926|gnl|PRJNA421796|CXP32 5|gb|AUF85492.1|Protein
- putative membrane protein [Streptococcus pneumoniae ATCC 700669]putative membrane protein [Streptococcus pneumoniae ATCC 700669]gi|220675000|emb|CAR69577.1|Protein
Your browsing activity is empty.
Activity recording is turned off.
See more...