By agreement with the publisher, this book is accessible by the search feature, but cannot be browsed.
NCBI Bookshelf. A service of the National Library of Medicine, National Institutes of Health.
Siegel GJ, Agranoff BW, Albers RW, et al., editors. Basic Neurochemistry: Molecular, Cellular and Medical Aspects. 6th edition. Philadelphia: Lippincott-Raven; 1999.
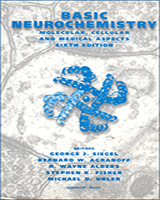
Basic Neurochemistry: Molecular, Cellular and Medical Aspects. 6th edition.
Show detailsThe availability of some nutrients can have immediate effects on behavior, especially on the ability to respond to stimulation. Several studies suggest that brain function, including cognitive processing, responds to changes in nutrients.
Nutrition can influence neurotransmitter concentrations and associated behaviors
Important neurotransmitters are synthesized from compounds which are essential dietary constituents. For instance, norepinephrine (NE) and serotonin are formed from the essential amino acids tyrosine and tryptophan, respectively. However, elevation of a precursor in the blood does not necessarily elevate its concentration in the brain. For example, increasing the blood concentration of large neutral amino acids such as phenylalanine, as occurs in phenylketonuria (see Chap. 44), reduces tryptophan uptake into the brain because these two compounds share a common carrier across the blood—brain barrier (see Chap. 32). Furthermore, the response to an increased concentration of precursor often depends on the demand, such as firing frequency of the neurons. Enhanced precursor availability may matter only when physiological demand is increased.
Choline for acetylcholine (ACh) synthesis can be obtained from either brain choline, the phosphatidylcholine in the membranes or serum choline (Table 33-1). It is taken up by a high-affinity choline-uptake system at the synapse (see Chap. 11). Although choline can be made in the body, its synthesis can be limited by the availability of “single-carbon” units in the diet. Ingestion of choline together with phosphatidylcholine can increase brain choline and ACh concentrations and enhance the ability of ACh synthesis to increase upon demand. For example, increased dietary choline permits the brain to make excess ACh following stimulation with atropine. Dietary phosphatidylcholine simultaneously increases memory and the ACh content of the brains of “demented” mice, which normally have reduced brain ACh concentrations [1]. Increasing choline prenatally and postnatally improves the working and reference memory of young rats.
Table 33-1
Dietary Manipulation of Neurotransmitters: Increases in Precursor Availability Increase the Ability of Many Neurotransmitters to Respond to Increased Demand.
Glucose normally provides the acetyl moiety of ACh. Extensive evidence indicates that relatively modest increases in circulating glucose concentrations can also increase ACh release and has been claimed to enhance learning and memory. The relative safety of glucose administration has permitted tests of its effects on cognitive functions in humans. Glucose enhances learning and memory in healthy aged humans and improves several other cognitive functions in subjects with severe cognitive pathologies, including individuals with Alzheimer's disease and Down's syndrome. Thus, moderate increases in circulating glucose concentrations may have robust and broad influences on brain functions that span many neural and behavioral measures and cross readily from rodents to humans. Considerable evidence suggests that these effects are mediated via ACh. Increasing glucose availability can increase the amount of ACh released during conditions of increased demand [2] (Fig. 33-1) (see also Chaps. 11 and 31).

Figure 33-1
The relation of glucose levels to the release of acetylcholine (ACh) and performance of a behavioral task. Increasing glucose availability by intraperitoneal injection can increase the release of ACh during conditions of increased demand. A glucose-stimulated (more...)
Tryptophan, like tyrosine, crosses the blood—brain barrier predominantly by the carrier system for long-chain neutral amino acids. As a result, a protein-rich meal can actually increase blood tryptophan but reduce the passage of tryptophan into the brain by elevating at the same time the concentrations of other amino acids, such as phenylalanine, that compete for that carrier. Serotonin (5-hydroxytryptamine, 5-HT) synthesis depends on brain tryptophan, which in turn depends on blood tryptophan concentrations, which can be manipulated by varying the diet (Table 33-1). Elevating tryptophan in the brain produces physiologically important changes in the serotonergic system (see Chap. 13). Animals that are poor in brain tryptophan have a heightened sensitivity to painful stimuli that can be reversed with tryptophan ingestion, which rapidly elevates brain serotonin. Therapeutically, tryptophan has been reported to be useful in treating subgroups of patients with depression, sleeplessness or hyperactive behaviors.
Tyrosine is the precursor of NE and epinephrine (Table 33-1). Increasing tyrosine reduces blood pressure in both normotensive and hypertensive animals. The action of tyrosine on blood pressure occurs via CNS mechanisms since co-administering other large neutral amino acids that reduce the uptake of tyrosine into the brain blocks the effect. The antihypertensive action of tyrosine appears to be mediated by an acceleration in NE or epinephrine release within the CNS; injection of tyrosine produces a concurrent increase in brain concentrations of 3-methoxy-4-hydroxyphenylethylglycol sulfate, a catecholamine metabolite [3]. Tyrosine induces increased NE and alters NE and α and β receptor densities in hippocampus, providing further evidence of its physiological role. Furthermore, dietary restriction to 40% of normal food intake diminishes maze performance, and this effect can be reversed by administration of tyrosine.
Nutrition can influence brain energy reserve
Brain energy is more resistant to changes in fasting or overfeeding than that in liver or muscle. For example, severe fasting decreases liver ATP concentrations and ATP: phosphocreatine ratios, while brain energy metabolism is preserved. However, brain energy metabolism can be manipulated by diet. A high-fat (90% of caloric value), carbohydrate-free ketogenic diet low in protein (10%) does not significantly alter regional brain glucose utilization or cerebral concentrations of glucose, glycogen, lactate or citrate. However, a high-carbohydrate diet (78%) low in fat (12%) and low in protein (10%) markedly decreases brain glucose utilization and increases cerebral concentrations of glucose 6-phosphate. These findings indicate that long-term, moderate ketonemia does not significantly alter brain glucose phosphorylation. However, even marginal protein dietary deficiency when coupled with a carbohydrate-rich diet depresses cerebral glucose utilization to a degree often seen in metabolic encephalopathies (see Chap. 38) [4].
Carnitine participates in mitochondrial reactions. Like choline, it can be synthesized by mammals if dietary sources of one-carbon groups are adequate. It participates in the transfer of acyl groups across mitochondrial membranes (Chap. 42). These include acetyl groups for ACh synthesis. Both carnitine and acetylcarnitine cross the blood—brain barrier (BBB), but the more lipid-soluble acetyl-l-carnitine has been described as having a variety of effects on the nervous system in experimental animals not seen with carnitine.
Hereditary deficits in the ability to transport carnitine or to synthesize its acyl derivatives have been associated with diseases of skeletal and cardiac muscle and, to a variable extent, with metabolic encephalopathy (see Chap. 34). Secondary deficiency of carnitine has been described in a number of disorders of mitochondrial oxidation, due in part to the detoxification and urinary excretion of potentially damaging short-chain acids as the carnitine derivatives [5]. The anticonvulsant valproic acid can increase carnitine requirements in susceptible individuals [6]. Treatment with acetylcarnitine has been reported to slow the progression of Alzheimer's disease [7].
Vitamins can regulate normal neuronal activity
Many vitamins function as cofactors in fundamental pathways, such as glycolysis, the Krebs cycle, the respiratory chain and amino acid metabolism. Although all tissues have these vitamin-dependent pathways, they take on increased importance in the brain because of its high metabolic rate and dependence on continuous metabolism. In fact, the discovery of vitamins was closely linked to the sensitivity of the brain to deficiency, specifically that of thiamine [8]. Furthermore, in the brain these pathways are linked to neurotransmitter synthesis.
Vitamin B1 (thiamine) is critical to normal brain function. Thiamine pyrophosphate (TPP) functions as a cofactor of key enzymes of the Krebs cycle: the pyruvate and α-ketoglutarate dehydrogenase complexes (PDHC and KGDHC, respectively), the branched-chain dehydrogenase complex (BCDHC) and the pentose shunt enzyme transketolase (TK) (Table 33-2). These dehydrogenase complexes share a common enzyme component, lipoamide dehydrogenase. TK rearranges sugars (see Chap. 31). A kinase can convert a membrane-bound form of TPP to thiamine triphosphate (TTP), and a specific phosphatase hydrolyzes TTP to the diphosphate. TTP appears to play a role in nerve membrane function, notably in Na+ gating. The cDNAs for a number of TPP-requiring enzymes have been obtained, and a TPP-binding motif has been proposed that is partially conserved in yeast, rat and human.
Table 33-2
Role of Vitamins in Brain Function: These Vitamins Are Critical for Normal Brain Function and Are Required in the Diet.
Thiamine deficiency is a classical and well-studied example of the interaction of nutrition with brain function. Research on thiamine deficiency continues to attract considerable interest. In developed countries, clinically significant thiamine deficiency is rare except as a complication of severe alcoholism or other conditions that impair nutrition [9]. It is more common in developing countries in which polished rice is the staple grain. It can be detected by measuring the “TPP effect,” the percentage increase in red cell TK activity upon addition of exogenous TPP in vitro, and has been widely used in laboratory as well as in epidemiological studies of thiamine deficiency.
After 5 to 6 days of a diet deficient in thiamine, healthy young men developed a nonspecific syndrome of lassitude, irritability, muscle cramps and electrocardiographic changes, which were reversed by dietary thiamine.
Prolonged thiamine deficiency frequently leads to damage to peripheral nerves (see Chap. 36). This neuropathy tends to be worse distally than proximally, involves myelin more than axons and is often painful. The neuropathy may be linked to deficiencies in multiple water-soluble vitamins known for historical reasons as the vitamin B complex.
Wernicke-Korsakoff syndrome consists of an acute (Wernicke) phase and a chronic (Korsakoff) phase [9]. The acute syndrome consists of staggering gait, paralysis of eye movements and confusion, associated with small hemorrhages along the third and fourth ventricles and with reduced cerebral metabolic rate as measured by cerebral blood flow. Injections of thiamine can be lifesaving, with clinical improvement often evident within minutes. It is believed that prompt treatment with thiamine can prevent the onset of the chronic Korsakoff phase. In Korsakoff's syndrome, a striking loss of working memory accompanies relatively little loss of reference memory (see Chap. 50). Affected patients characteristically make up stories, or confabulate, in response to leading questions. In this phase, patients do not respond to thiamine treatment. The neuropathological lesions responsible for Korsakoff's syndrome have been debated; severe damage to the cholinergic neurons of the nucleus basalis complex has been reported.
Thiamine requirements can be altered genetically or environmentally. Among genetic disorders, thiamine-dependent maple syrup urine disease is due to a reduced affinity of BCDHC for TPP (see Chap. 44). A rare form of lactate acidosis is due to reduced affinity of PDHC for TPP. Both disorders respond to treatment with large doses of thiamine. Wernicke-Korsakoff syndrome is associated with a variant form of TK having a decreased affinity for TPP [9]. This variation, which may be more common in chronic alcoholics, puts patients at risk when on a diet marginal or deficient in thiamine. Subacute necrotizing encephalomyelopathy (SNE) of Leigh is an uncommon, autosomal recessive disorder in which the neuropathology resembles Wernicke-Korsakoff syndrome. Patients with SNE in whom a defect in PDHC has been documented at the cDNA level have been described. The role of thiamine in this disorder is controversial.
Environmentally, a number of dietary constituents are known to impair the absorption of thiamine, including ethanol. Severe illness or injury also has been reported to increase thiamine requirements. Rarely, patients have been found who are intolerant to very large doses of thiamine. Thiamine-dependent enzymes are reduced in the brains of patients with a variety of neurodegenerative diseases, including Alzheimer's, Huntington's and Parkinson's diseases.
Thiamine-deficient animals model many aspects of human thiamine deficiency [10]. Experimentally, thiamine deficiency is frequently induced by the combination of a thiamine-deficient diet and a thiamine antagonist, either pyrithiamine or oxythiamine. However, pyrithiamine can directly inhibit action potentials and oxythiamine does not enter the brain efficiently. In the pyrithiamine model in mice, abnormal neuropsychological responses develop within 5 to 7 days, gross neurological abnormalities in 8 to 9 days and death usually by 10 to 11 days. Strain significantly modifies the response to experimental thiamine deficiency in mice (Fig. 33-2). In rats, abnormalities of motor performance occur by day 3, additional neurological symptoms by day 12 and death within 2 weeks. Thiamine deficiency leads to a selective cell death that is accompanied by accumulation of amyloid precursor protein in surrounding neurons. It causes severe memory disruption and loss of cholinergic function. The activities of thiamine-dependent enzymes decline in early stages of thiamine deficiency, but surprisingly, selective cell death is not related to the cellular or regional distribution of thiamine-dependent enzymes or to their response to thiamine deficiency. Instead, the general reduction in thiamine-dependent enzymes predisposes particular brain regions to other insults. The earliest known change that reflects selective vulnerability is an alteration in the BBB that is accompanied by oxidative stress, which causes increased ferritin and iron deposition, and induction of nitric oxide synthase. The results suggest that cerebrovascular endothelial cells of these brain regions may be particularly vulnerable to thiamine deficiency [10].

Figure 33-2
Strain and age differences in response to thiamine deficiency in mice. A: Transketolase activity in the brains of thiamine-deficient and pair-fed control Balb/C and CD-1 mice. B: β-Ketoglutarate dehydrogenase complex activity in the brains of (more...)
Vitamin B3 (niacin) deficiency in humans leads to pellagra, which is characterized by dementia, dermatitis, diarrhea and eventually death. The deficiency was recognized in the eighteenth century, shortly after the introduction of American corn (maize) into Europe [8].
Niacin and niacinamide refer to nicotinic acid and its amide, respectively. Although these pyrimidine derivatives can be synthesized from tryptophan in mammals, perhaps at least in part by intestinal bacteria, 60 mg of dietary tryptophan are required to synthesize 1 mg of the vitamin. Niacin is considered to be a vitamin because most human diets do not contain enough tryptophan to fulfill the normal human requirement for the vitamin of 10 to 30 mg/day.
Hartnup's syndrome is a hereditary disorder in which tryptophan transport is impaired and requirements for dietary niacin increase. Phenylketonuria and hyperphenylalaninemia can increase niacin requirements by increasing the concentrations of amino acids that compete with tryptophan for transport systems (see also Chap. 44). A high-corn diet predisposes to niacin deficiency since the major storage protein of American corn (zein) has relatively little tryptophan relative to other amino acids that compete for the same carrier. Addition of purified niacin to the diet has largely abolished pellagra, which was once a common disease in areas where corn was a dietary staple.
Niacin is incorporated into the coenzymes NAD+ and NADP+ and their reduced forms. These cofactors are involved in numerous oxidation/reduction reactions, including the coupling of the Krebs cycle to the respiratory chain. Antimetabolites, particularly 6-aminonicotinamide and 3-acetylpyridine, have been particularly useful in determining the role of niacin deficiency in the brain in experimental animals. Newborn mice that received a single intraperitoneal injection of 6-aminonicotinamide consistently developed lesions in the CNS, the skin and the intestinal tract. Anterior horn cells in the spinal cord as well as motor neurons in the brain exhibit the ultrastructural features of neuronal chromatolysis, while glial and ependymal cells show edematous changes. 3-Acetylpyridine administration leads to selective neuropathological lesions in the brainstem. Although the pathological features of antimetabolite-treated mice are not identical to those of human pellagra, possible contributory mechanisms in the development of pellagra lesions, including dementia and selective cell loss, may be elucidated with this experimental model [11].
Vitamin B6 (pyridoxine) is necessary for the biosynthesis of several neurotransmitters. It is a pyridine derivative that can exist as an alcohol, amine or aldehyde. The concentration in brain is normally about 100-fold higher than that in the blood. The active coenzyme is the phosphorylated derivative pyridoxal phosphate, which readily forms Schiff bases. This coenzyme participates in decarboxylation reactions, including those that form GABA from glutamate, 5-HT from 5-hydroxytryptophan and probably DOPA from dihydroxyphenylalanine. It is also involved in transaminations, including that converting α-ketoglutarate to glutamate. The conversion of tryptophan to nicotinamide requires pyridoxyl phosphate as a cofactor, and the excretion of xanthurenic acid after a tryptophan load is widely used to test the adequacy of pyridoxine nutriture. In vitamin B6 deficiency in rats, biochemical and morphological abnormalities, including decreased dendritic arborization and reduced numbers of myelinated axons and synapses, are associated with behavioral changes, such as epileptiform seizures and movement disorders. Reduced seizure threshold and delayed neuronal recovery are related to the significantly reduced brain regional GABA and elevated glutamate concentrations in pyridoxine-deficient rats [12]. In addition, vitamin B6 deficiency during gestation and lactation alters the function of N-methyl-d-aspartate (NMDA) receptors.
Pyridoxine deficiency has occurred in human infants fed a formula from which vitamin B6 had been inadvertantly omitted. The prominent finding was intractable seizures which responded promptly to injections of the vitamin. Deficiency of pyridoxine can contribute to the polyneuropathy of B-complex deficiency. However, very large doses of pure pyridoxine can lead to a persistant sensory neuropathy [13] (Chap. 36).
Like those of other nutrients, requirements for pyridoxine can be altered by genetic or environmental factors and are increased in a number of disorders of the nervous system [8,14,15]. Treatment of “pyridoxine-deficient” infants may require doses of pyridoxine several hundredfold the normal daily requirement. Maintenance with doses at least tenfold the normal requirement typically permits normal development if irreversible brain damage has not yet occurred. It has been suggested that mild forms of pyridoxine dependence may be a relatively common cause of intractable seizures and mental retardation, but neurochemical studies of these patients are limited. In homocystinuria and cystothioninuria, two disorders of amino acid metabolism, some patients respond to large doses of pyridoxine. In these patients, the mutations appear to reduce the affinity of the relevant enzymes for pyridoxal phosphate (see Chap. 44).
Environmentally, hydrazides and oximes can increase pyridoxine requirements. Large doses of pyridoxine are routinely given with the antituberculous medication isonicotinic hydrazide, to prevent drug-induced neuropathy.
Vitamin B12 (cobalamin) deficiency is commonly associated with neurological syndromes. The cobalamins are a series of porphyrin-like compounds [16]. The active forms contain a cobalt ion linked to one of the methylene groups. The cobalamins are synthesized by many microorganisms but not by higher plants or animals. A rich dietary source is meat, particularly liver. Effective absorption requires a series of transport proteins, including a glycoprotein intrinsic factor secreted by gastric parietal cells. Conversion to the active coenzymes adenosylcobalamin and methylcobalamin requires at least two reductase reactions and an adenosyltransferase step. The reductases are flavoproteins that require NAD+ as a cofactor. Thus, B12 metabolism involves at least three vitamins: B12 itself, niacin and riboflavin. Body stores of cobalamins are normally large enough to maintain health for over 2 years without a dietary source of the vitamin.
Cobalamins have two well-established biochemical functions. Adenosylcobalamin is the cofactor for the mutase that converts methylmalonyl CoA to succinyl CoA. This reaction is part of the pathway of metabolism of propionic acid, which itself derives from the metabolism of odd-chain fatty acids and from certain amino acids. Methylcobalamin is the cofactor for the methyltransferase that converts homocysteine to the amino acid methionine. This reaction is important in folate metabolism as well. Its impairment appears to foster folate deficiency by an accumulation of N5-methyltetrahydrofolate in a “folate trap.” Deficiency of cobalamins or of folate or of both can restrict the supply of metabolically available one-carbon groups for metabolic pathways, including those of nucleic acid synthesis.
Cobalamin deficiencies are relatively common clinically [16]. Pure dietary deficiency responding promptly to treatment with oral cobalamins has been described in a few children of strict vegan mothers. A more common syndrome is caused by failure of absorption due to an inadequate supply of the glycoprotein intrinsic factor, usually on an autoimmune basis. The most characteristic abnormality is pernicious anemia, characterized by enlarged erythrocytes, called megaloblasts, and abnormal leukocytes. Neurological symptoms occur in many of these patients and can precede the hematological changes [17].
Combined system disease is the most common B12-mediated neurological syndrome. Affected patients develop unpleasant tingling sensations (paresthesias), followed by loss of vibratory sensation, particularly in the legs, and spastic weakness. The characteristic neuropathology is a spongy demyelination in the long tracts of the spinal cord, particularly prominent in the posterior columns as well as corticospinal tracts. Combined system disease responds poorly to treatment with cobalamins.
Cobalamin deficiency is characteristically associated with malaise that does respond dramatically to treatment, even before the hematological response is evident. Relatively low serum concentrations of B12 have been reported in subgroups of psychiatric patients, including patients with Alzheimer's disease, but responses to treatment with the vitamin have, in general, not been dramatic. Recent studies indicate that elevated concentrations of serum or cerebrospinal fluid methylmalonate can identify patients whose neuropsychiatric manifestations benefit from B12 treatment, even though the amounts of vitamin in serum are not in the deficient range [17].
Whether the damage to the nervous system relates to decreased activity of the methylmalonyl mutase or of the methyltransferase or of both remains unsettled. Increased excretion of methylmalonate has been reported to be a marker for patients whose neuropsychiatric manifestations will improve with B12 treatment, but clinically normal children with a mutase deficiency are known. Children with homocystinuria and related disorders do not develop the clinical or pathological stigmata of combined system disease (see Chap. 44). An infant with an apparent reduction in methyltransferase activity was clinically normal when reported at age 1 year. Patients with severe inherited deficiencies in the activities of both enzymes secondary to a defect in the metabolism of the cobalamins do develop profound disease of the nervous system, with some characteristics of combined system disease.
As with other nutrients, requirements for cobalamin can be modified by genetic and environmental influences. Genetic factors apparently predispose to intrinsic factor deficiencies with resultant cobalamin deficiency. Furthermore, at least six different inherited methylmalonic acidurias have been described [16]: absence of the mutase, decreased affinity of the mutase for adenosylcobalamin, deficiency of mitochondrial cobalamin reductase, deficiency of a mitochondrial cobalamin adenyltransferase and two distinguishable defects associated with abnormal cytosolic metabolism of cobalamin (see Chap. 44). Other conditions leading to increased cobalamin requirements include surgical removal of the stomach, excessive destruction of cobalamins in the gut by bacteria in a blind loop or destruction by certain kinds of intestinal tapeworm.
Folic acid contains a pterin moiety linked to para-aminobenzoic acid, which is linked to one or more glutamate residues [18]. It plays a key role in the transfer of one-carbon (active methylene) groups, including the conversion of serine to glycine and the cobalamin-dependent transfer from homocysteine to methionine. Dietary deficiency of folate with normal cobalamin leads to anemia without significant neurological signs. However, both genetic and environmental disorders of folate metabolism have been associated with disease of the nervous system. Genetic defects in the relevant enzyme reactions are discussed further in Chapter 44.
Genetic disorders of folate absorption, intraconversion and utilization are rare [18]. They have occasionally been associated with phenocopies of well-known psychiatric syndromes. A boy with apparent deficiency of hepatic dihydrofolate reductase was treated with folate and developed a sociopathic personality in his teens. A folate-responsive form of mental retardation with catatonia has been described in an adolescent girl with N5,10-methylenetetrahydrofolic acid reductase deficiency. Her younger sister was mentally impaired with “psychosis”; an unrelated boy with a defect of the same enzyme had seizures and proximal muscle weakness without notable psychiatric problems. Most patients with glutamate formiminotransferase deficiency have had a syndrome of psychomotor retardation in infancy, but a few have been entirely normal clinically.
Environmentally, a number of common medications, including phenytoin and certain antitumor agents, increase requirements for dietary folate. Treatment with folate can mask the hematological signs of cobalamin deficiency without affecting the progressive damage to the nervous system.
Pantothenic acid is a substituted hydroxybutyric acid that is a constituent of CoA [19]. Experimental induction of pantothenic acid deficiency leads to signs of peripheral nerve damage, for example, demyelination in laboratory animals and paresthesias in humans. Late signs of CNS damage in animals may relate as well to the adrenal failure that is a prominent part of the syndrome.
The brain depends on select vitamins and closely related compounds as antioxidants to control potentially damaging free radicals
The main antioxidants in brain are vitamin E (tocopherol), vitamin C (ascorbic acid) and glutathione (Table 33-2). The first two can be easily manipulated by diet, whereas the latter is more difficult to control. Dietary α-lipoate appears to be a useful compound to regenerate the antioxidant capacity of these other compounds (see below). Dietary manipulation of antioxidants has practical implications for brain function. Aging has been associated with free radical damage in the brain (see Chap. 34). In aged patients tested over a 22-year period, free recall, recognition and vocabulary correlated positively with ascorbic acid and β-carotene in blood, even after controlling for possible confounding variables, such as age, education and gender. These results indicate the important role played by antioxidants in brain aging and may have implications for prevention of progressive cognitive impairments [20].
Vitamin E (α-tocopherol) deficiency produces a characteristic neurological syndrome. It presumably results from increased oxidative stress arising from a reduction in antioxidant capacity. Vitamin E deficiency in neural tissues increases endogenous lipid peroxidation, as evidenced in brain tissues by the appearance of thiobarbituric acid-reactive substances such as malondialdehyde. The brain is more susceptible to the deficiency than muscle. Within the brain, the cortex, striatum and cerebellum are the most sensitive regions. Isolated fractions from myelinated nerve tracts show that the axoplasmic membranes and organelles are particularly vulnerable to oxidative stress [21]. Vitamin E deficiency reduces tyrosine hydroxylase-immunopositive neurons in the substantia nigra but not in the adjacent ventral tegmental area. The enhanced sensitivity of the nigrostriatal pathway to oxidative stress could have important implications for the pathogenesis of Parkinson's disease (see Chap. 45). A diet deficient in vitamin E increases glutamate and GABA and decreases tryptophan concentrations in the substantia nigra. The increase of nigral glutamate suggests possible links to degenerative processes through glutamatergic excitotoxicity. These results suggest that vitamin E may play a significant role in the degeneration of the substantia nigra and that this tissue may be particularly sensitive to oxidative stress. Furthermore, these findings support the widely held view that oxidative stress in the substantia nigra is important in the pathophysiology of Parkinson's disease.
Vitamin C (ascorbate) deficiency leads to extensive oxidative damage of proteins and protein loss in the microsomes, as evidenced by accumulation of carbonyl groups on proteins as well as tryptophan loss. This oxidative damage is reversed by ascorbate therapy. Ascorbate deficiency also leads to lipid peroxidation in microsomes, as evidenced by accumulation of conjugated dienes, malondialdehyde and fluorescent pigment. Lipid peroxides disappear after ascorbate therapy but not after treatment with vitamin E. These results indicate that vitamin C may exert a powerful protection against degenerative changes in the brain associated with oxidative damage [22].
Oxidation of vitamin E and C is maintained by glutathione, the predominant thiol antioxidant in the brain. Glutathione cannot be directly manipulated by diet, whereas the metabolic antioxidant α-lipoate can be absorbed from the diet and cross the BBB to reduce oxidized glutathione and vitamins A and C (Fig. 33-3). α-Lipoate is taken up and reduced in cells and tissues to dihydrolipoate, which is also exported to the extracellular space; hence, protection is afforded to both intracellular and extracellular environments. Both α-lipoate and dihydrolipoate are potent antioxidants that regenerate other antioxidants, like vitamins C and E, and raise intracellular glutathione concentrations. Protective effects by antioxidants have been reported in cerebral ischemia—reperfusion, excitotoxic amino acid brain injury, mitochondrial dysfunction, diabetes and diabetic neuropathy, inborn errors of metabolism and other causes of acute or chronic damage to the brain or neural tissue. Thus, α-lipoate administration may prove to be an effective treatment in numerous neurodegenerative disorders [20].

Figure 33-3
Interaction of various aspects of the oxidative status of the brain that depend on diet. The elaborate mechanisms of the brain to minimize free radical formation depend on many dietary constituents, including vitamin C, vitamin E, α-lipoate, the (more...)
Trace nutrients in the diet have a vital role in maintaining normal brain function
Zinc (Zn2+) influences numerous cellular functions, including immune mechanisms, actions of several hormones and enzyme activities. More than 200 enzymes are known to be Zn2+-dependent, including mRNA-editing enzymes, superoxide dismutase, metalloproteins and a “Zn2+-finger” family of sequence-specific DNA-binding proteins that regulate transcription. Metallothionein binds excess Zn2+, thus maintaining its steady-state concentration and preventing inhibition of an extensive number of sulfhydryl-containing enzymes and receptor sites; hence, it protects against metal-related neurotoxicity. Metallothionein donates Zn2+ to an extensive number of Zn2+-activated, pyridoxal phosphate-mediated biochemical reactions. The complex nature of the interactions of Zn2+ with multiple enzymes is exemplified by the observation that epileptic seizures that are blocked by GABA can be blocked by either deficiency or excess of either Zn2+ or pyridoxal phosphate. A proposed explanation of these observations is that at physiological concentrations Zn2+ stimulates the activity of hippocampal pyridoxal kinase, enhancing the formation of pyridoxal phosphate and of GABA via glutamate decarboxylase formation, whereas at higher doses Zn2+ inhibits the activity of glutamate decarboxylase by preventing the binding of pyridoxal phosphate [23]. Severe Zn2+ deficiency during the period of rapid brain growth has effects similar to that seen with protein-calorie malnourishment, including altered regulation of emotions; food motivation; lethargy (reduced activity and responsiveness), and deficits in learning, attention and memory. In addition to the many deficits produced by Zn2+ deficiency in the brain, the severe effect of Zn2+ deficiency on other tissues leads to additional peripheral mechanisms that alter brain function [24]. Although Zn2+ is essential at low concentrations, higher concentrations are toxic. For example, high Zn2+ concentrations enhance and prolong the firing rate of neurons, significantly depress paired-pulse potentiation, block the action of NMDA on cortical neurons, enhance quisqualate receptor-mediated injury and inhibit the Ca2+-dependent release of transmitter by inhibiting the entry of Ca2+ into nerve terminals.
Copper is an integral component of multiple cellular enzymes, including the cytochromes and superoxide dismutase. Copper deficiency produces clinical signs analogous to those of Par-kinson's disease and results in low dopamine concentration in the corpus striatum. Neuropathology in experimental animals occurs in only part of the copper-deficient population and is dam- and litter-related, suggesting the presence of a genetic component that alters the response to copper deficiency. Insight into the role of copper in brain function is provided by two genetic diseases.
Wilson's disease is an inherited disorder that leads to copper accumulation, causing damage primarily to the liver and the brain (see Box 45-1). Psychiatric and behavioral abnormalities occur in 30 to 100% of Wilson's disease patients and are often the initial symptoms. The most common of the psychiatric and behavioral manifestations include personality changes, such as irritability and low anger threshold; depression, sometimes leading to suicidal ideation and attempts; and deteriorating academic and work performance, which is present in almost all neurologically affected patients [25].
Menke's disease is caused by a genetic deficiency of serum copper and of copper-dependent enzymes and is characterized by neurological degeneration and mental retardation, connective tissue and vascular defects, brittle and depigmented hair and death in early childhood (see Box 45-1). Despite excessive accumulation of the metal in various tissues, a functional copper deficiency is evident, caused by a defective intracellular copper-transport protein. A large amount of copper accumulates in the organelle-free cytoplasm, whereas mitochondria are in a state of copper deficiency, indicating that the Menke's mutation probably affects copper transport from the cytosol to the intracellular organelles [26]. Brindled is a murine mutation that produces similar copper-transport deficits, and studies of this animal model show that the copper deficit in organelles causes reductions in critical copper-dependent enzymes, such as cytochrome oxidase. It has been hypothesized that the Wilson's disease gene is a copper-transporting ATPase with homology to the Menke's disease gene. Dietary copper deficiency can affect brain development [27] (see below).
Selenium is vital in maintaining the antioxidant capacity of the brain. Glutathione peroxidase is a selenium-dependent enzyme that is important for maintaining the antioxidant capacity of brain glutathione (Fig. 33-2) and is reduced in selenium-deficient animals. Selenium supplementation significantly elevates Na,K-ATPase activity and significantly decreases lipid peroxide formation. Since Na,K-ATPase activity is known to be inhibited by oxygen free radicals, selenium supplementation appears to exert its beneficial effect on the Na,K-ATPase activity by preventing free radical-induced damage. Selenium significantly reduces the production of thiobarbituric acid-reactive substances, a measure of lipid peroxidation, in response to an oxidative challenge in blood and different regions of the brain [28]. Selenium deficiency increases dopamine turnover in the substantia nigra but not in the striatum. These results suggest that dietary selenium protects the brain, particularly the substantia nigra, against oxidative damage.
Trace compounds are also important in brain function. Chromium-deficient patients develop severe diabetic symptoms, including glucose intolerance, weight loss, impaired energy utilization and nerve and brain disorders. Low dietary boron is reported to cause significantly poorer performance on various cognitive and psychomotor tasks. Additional research is likely to reveal additional trace components of the diet that may be critical to normal brain function.
- Nutrition can influence neurotransmitter concentrations and associated behaviors
- Nutrition can influence brain energy reserve
- Vitamins can regulate normal neuronal activity
- The brain depends on select vitamins and closely related compounds as antioxidants to control potentially damaging free radicals
- Trace nutrients in the diet have a vital role in maintaining normal brain function
- Nutrition and Functional Neurochemistry - Basic NeurochemistryNutrition and Functional Neurochemistry - Basic Neurochemistry
Your browsing activity is empty.
Activity recording is turned off.
See more...