By agreement with the publisher, this book is accessible by the search feature, but cannot be browsed.
NCBI Bookshelf. A service of the National Library of Medicine, National Institutes of Health.
Siegel GJ, Agranoff BW, Albers RW, et al., editors. Basic Neurochemistry: Molecular, Cellular and Medical Aspects. 6th edition. Philadelphia: Lippincott-Raven; 1999.
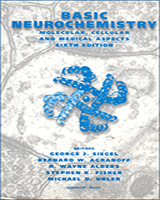
Basic Neurochemistry: Molecular, Cellular and Medical Aspects. 6th edition.
Show detailsThe brain has a number of characteristics which make it especially susceptible to free radicalmediated injury. Brain lipids are highly enriched in polyunsaturated fatty acids (PUFA), and many regions of the brain, for example, the substantia nigra and the striatum, have high concentrations of iron. Both of these factors increase the susceptibility of brain cell membranes to lipid peroxidation (see also Chap. 45). Because the brain is critically dependent on aerobic metabolism, mitochondrial respiratory activity is higher than in many other tissues, increasing the risk of free radical “leak” from mitochondria; conversely, free radical damage to mitochondria in brain may be tolerated relatively poorly because of this dependence on aerobic metabolism.
Oxygen free radicals are required intermediates in many biological reactions but may damage macromolecules during oxidative stress
Free radicals are molecules which posses an outer electron orbital with a solitary unpaired electron; these include the hydrogen atom (H•); the diatomic oxygen molecule O2, which possesses two unpaired electrons with the same spin in two separate orbitals; NO•; superoxide (O•−2); hydroxyl radical (•OH); and transition metals, such as copper and iron. While O2 qualifies as a radical by having two unpaired electrons, its reactivity with nonradical compounds is limited because the unpaired electrons in O2 have the same spin state. The two electrons in a covalent bond have opposite spins, so in order for O2 to react with a nonradical, one of the electrons must undergo “spin inversion” so that both are anti-spin to the electrons on O2, an extremely slow process. O2 does react readily with radicals, accepting one electron at a time to form the very reactive superoxide radical O•−2, which has one unpaired electron [21].
Although some radical species may persist for prolonged periods, most are generally unstable and will attempt to donate their unpaired electron to a nearby molecule or to remove or “abstract” a second electron, usually in the form of a hydrogen atom, from a neighboring molecule to pair with their free electron. Free-radical reactions are intrinsic to a majority of the metabolic and synthetic reactions carried out by eukaryotic cells and, as such, are required for life. ATP production by the mitochondrial electron chain, for example, uses a controlled set of oxygen radical reactions to couple the reduction of free-radical electrons with the movement of protons across the mitochondrial membrane. Cytochrome oxidase, complex IV of the mitochondrial electron transport chain (Chap. 42), catalyzes transfer of these free electrons to molecular oxygen as the final acceptor with water as the end product.
The addition of oxygen to macromolecules, such as in the metabolism of arachidonic acid to the eicosanoids or the oxidation of small molecules by P450 enzymes, requires “activation” of molecular O2 to permit transfer of atomic oxygen (O•) from O2 to the biological compound. Most enzymes which catalyze biological radical reactions bind a metal ion (Fe, Cu, Co or the group VI element Se), which destabilizes the O2 molecule. These reactions also involve cofactors such as flavin adenine dinucleotide (FAD) or flavin mononucleotide (FMN) to help stabilize the resulting oxygen atoms until the reaction is complete.
Although such reactions are generally very efficient, there is often some small amount of leak of ROS encompassing radicals such as O•−2, its acid HO2, hydroxyl radical (•OH) and NO•, as well as nonradicals such as hydrogen peroxide (H2O2), singlet oxygen (1OΔg) and hypochlorous acid (HOCl). While H2O2 is not a free radical, it can be rapidly decomposed via the Fenton reaction:
In addition, superoxide, hydrogen peroxide and hydroxyl radicals can be interconverted via the so-called Haber-Weiss reaction:
Cuprous and cupric ions may substitute for ferrous and ferric ions in the Haber-Weiss reaction [21]. Peroxynitrite can be formed from the reaction of NO with superoxide:
Oxidative stress generally describes a condition in which cellular antioxidant defenses are inadequate to completely detoxify the free radicals being generated, due to excessive production of ROS, loss of antioxidant defenses or, typically, both [22]. This condition may occur locally, as antioxidant defenses may become overwhelmed at certain subcellular locations while remaining intact overall, and selectively with regard to radical species, as antioxidant defenses are radical-specific, for example, SOD for superoxide and catalase or glutathione peroxidase for H2O2.
A major consequence of oxidative stress is damage to cellular macromolecules. Addition of the free “radical” electron to a fatty acid causes fragmentation of the lipid or alteration of its chemical structure (Fig. 34-6). For example, the unconjugated cis double bonds in unsaturated fatty acids may be shifted to produce a conjugated trans double bond system (see Chap. 2). Peroxyl or hydroxyl groups may be added to the unsaturated fatty acid, or the fatty acid carbon chain may be cleaved during reaction with the free electron to generate a fatty aldehyde, both processes termed lipid peroxidation. Fatty aldehydes such as 4-hydroxynonenal can then react with free thiol groups such as cysteines on proteins to produce thioesters, which may affect protein function and stability [21]. Free-radical damage to proteins may cause cross-linking, carbonyl formation and protein denaturation. DNA bases may be modified by oxidation, resulting in single- and double-strand breaks or mispairing of purine and pyrimidine during DNA replication.

Figure 34-6
Lipid peroxidation leads to fragmentation or oxidation of polyunsaturated fatty acids (PUFA). LOO•, lipid peroxyl radical; LO•, lipid alkoxylradical; HO•, hydroxyl radical; O•−2, superoxide radical; O•, (more...)
Reactive oxygen species generated during ischemia-reperfusion contribute to the injury
Evidence for free radical production and oxidative stress during ischemia-reperfusion comes from a variety of studies. While free radicals may be generated to a small extent during ischemia, far greater production of reactive oxygen intermediates occurs after reintroduction of oxygen during reperfusion. Early studies with animal models of ischemia-reperfusion injury showed decreased brain concentrations of antioxidants such as ascorbic acid, vitamin E and glutathione, as well as production of aldehydic lipid peroxidation products, detected as thiobarbituric acid-reactive substances. Additional evidence for ROS generation has been obtained with intraischemic microdialysis with solutions containing salicylic acid or spin-trapping agents, which react with ROS to form relatively stable products that can be detected in the microdialysis solution. Oxidative damage to macromolecules has provided another index of ROS-mediated injury in ischemia-reperfusion. Lipid- and protein-oxidation products as well as DNA-oxidation products have been detected in brain tissue after ischemia-reperfusion.
Treatment with antioxidants, such as vitamin E; 21-aminosteroids; and spin-trapping agents, such as phenyl tert-butyl N-nitrone (PBN), can reduce ischemic brain infarction. In addition, transgenic mice which overexpress the cytosolic antioxidant enzyme CuZn-SOD (SOD1) (see below) [23] or the extracellular SOD3 [24] are resistant to ischemic brain injury compared to wild-type controls. Conversely, reduction of cellular antioxidant defenses can potentiate ischemic injury. Transgenic mice that are sod1—/— exhibit enhanced susceptibility to ischemic brain injury, even though they have a grossly normal CNS phenotype under unstressed conditions, for example, in the absence of ischemia or nerve transection. This suggests that other antioxidant defenses are adequate to handle physiological concentrations of cytosolic superoxide anion. In contrast, mice lacking the mitochondrial antioxidant enzyme Mn2+-SOD (SOD2) die soon after birth [3]. This suggests that extracellular SOD3 or other antioxidant systems may be able to compensate partially for loss of cytosolic, but not mitochondrial, SOD activity.
Mitochondria, nitric oxide synthase and arachidonic acid metabolism are sources of reactive oxygen species during ischemia-reperfusion injury
ROS generation during ischemia-reperfusion may come from several sources, including NOS activity, mitochondrial electron transport, multiple steps in the metabolism of arachidonic acid and, in some species, xanthine oxidase, which is produced by hydrolysis of xanthine dehydrogenase. In addition, the decreased intracellular pH accompanying ischemia may alter the binding of transition metals such as iron, increasing their participation in the Haber-Weiss reaction [21]. P450 enzymes and NAD(P)H oxidase are two additional potential sources of oxygen radicals whose contribution to enhanced radical production during CNS ischemia has not been systematically explored.
Mitochondria were among the earliest sites of cellular ROS production to be identified. Studies on isolated mitochondria have suggested that as much as 3% of oxygen utilization by resting mitochondria may be lost as leak of ROS, although this estimate may be high. Mitochondrial production of ROS can be enhanced by increased electron-transport activity, as well as by disturbances in electron transfer down the transport chain [25]. The lipid electron-transport molecule ubiquinone (Q9, Q10) is the major site of free radical leak from mitochondria via the ubiquinone cycle. Ubiquinone undergoes a two-electron reduction, first to a semiquinone and then to a diol, at mitochondrial complex I (NADH dehydrogenase, NADH:ubiquinone oxidoreductase) or complex II (succinate dehydrogenase) and subsequently delivers the two electrons to the iron—sulfur center of complex III (cytochrome bc1 in mammalian mitochondria). Inhibition of either complex I or complex III will impair the efficiency of electron transfer and may allow a free semiquinone to be produced. The semiquinone can then interact with O2 to generate O•−2 (Fig. 34-7). Elevated intracellular Ca2+, exposure to fatty acids or other molecules which alter the physical properties of the mitochondrial membrane and inhibition of mitochondrial respiratory components may enhance this leak of ROS from mitochondria.

Figure 34-7
Sources of free radical formation which may contribute to injury during ischemia-reperfusion. Nitric oxide synthase, the mitochondrial electron-transport chain and metabolism of arachidonic acid are among the likely contributors, LOO•, lipid peroxyl (more...)
Mitochondria appear to be an important and, perhaps, even dominant source of free radicals in brain tissue subjected to ischemia-reperfusion. Microdialysis studies using salicylate to detect ROS release from ischemic brain cortex show that mitochondrial inhibitors such as rotenone eliminate ROS production during ischemia-reperfusion. Elevated concentrations of intracellular Ca2+ and Na+, a consequence of energy failure and excitotoxic glutamate receptor stimulation, can be expected to inhibit complex I as well as overproduction of superoxide anion. The resultant oxidative stress may lead to further inhibition of mitochondrial respiratory components, promoting further free radical production in a vicious, feed-forward cycle.
Nitric oxide and peroxynitrite contribute to oxidative damage
NOS has been identified as another source of ROS with special relevance to pathological conditions. NOS is homologous to P450 cytochrome c reductase; cofactors in the reaction are FMN, FAD, tetrahydrobiopterin and NAD(P)H (Fig. 34-7). NOS normally converts arginine and molecular oxygen to citrulline and NO•, a free radical gas; this process is itself leaky and associated with free radical generation [13]. NO•, originally identified as endothelial-derived relaxing factor (EDRF), is a potent vasodilator. NO• also binds to guanylyl cyclase to increase GTP production and can nitrosylate the small G protein Ras on a specific cysteine to cause constitutive activation. Three family members have been identified: Ca2+/calmodulin-activated brain NOS (nNOS), endothelial NOS (eNOS) and inducible NOS (iNOS). High concentrations of nNOS are found in a subpopulation of neurons previously identified as NAD(P)H-diaphorase cells.
NO•, which has limited radical reactivity, can combine readily with O•−2 and possibly H2O2 to produce a highly oxidizing, nonradical compound, peroxynitrite [13]. When protonated, peroxynitrite has reactivity similar to that of •OH. Cell culture studies suggest that while NO• itself is relatively nontoxic, peroxynitrite is quite neurotoxic. Peroxynitrite reacts with protein tyrosine residues to produce nitrotyrosine. Potential targets of tyrosine nitrosylation include many important cellular proteins, including Cu,Zn-SOD, aconitase, Mn-SOD and cytochrome oxidase (mitochondrial complex IV), all of which are inactivated by nitrosylation. Peroxynitrite may cause lipid peroxidation but may also generate nitrosolipids, which decrease lipid peroxidation by acting as peroxidation “chain-breakers.” In addition, peroxynitrite targets DNA, leading to chain breaks and DNA base oxidation. Involvement of nNOS as a mediator of ischemic injury is suggested by the ability of nNOS inhibitors to reduce ischemic injury and by the neuroprotective effect of nNOS gene deletion in knockout mice.
Peroxynitrite-mediated DNA damage can activate poly(ADP-ribose) polymerase (PARP or PARS), an enzyme involved in DNA repair. It has been suggested that activation of PARP, which catalyzes extensive hydrolysis of ATP to produce polyADP ribosylation of damaged DNA, contributes to ischemic injury by hastening energy depletion. Pharmacological studies in vitro and experiments with PARP knockout mice have suggested that inhibition of PARP provides substantial neuroprotection against ischemic neuronal injury.
Production of eicosanoids from polyunsaturated fatty acids such as arachidonic acid may generate reactive oxygen species
Arachidonic acid metabolism may also be a source of ROS production [26,27]. As discussed in Chapters 3 and 35, arachidonic acid undergoes an extensive array of reactions to biologically active lipids, the eicosanoids. These reactions may be accompanied by free-radical production. In particular, cyclooxygenase isoforms and 5-lipoxygenase contain heme iron and may generate a low concentration of superoxide anion constitutively. 12-Lipoxygenases, which possess nonheme iron, may not release superoxide anion but may induce lipid peroxidation after translocation to membranes.
The role of xanthine oxidase in human stroke is unclear
In rodent models of ischemia, xanthine oxidase inhibitors, such as allopurinol or oxypurinol, decrease ROS formation; but since these drugs also can serve as hydroxyl radical scavengers, it has been difficult to determine which of these effects is responsible for neuroprotection. Analyses of human tissues have shown high concentrations of xanthine dehydrogenase in liver and intestine but neither xanthine dehydrogenase nor xanthine oxidase in brain parenchyma and little in cerebral endothelial cells.
Brain antioxidant defenses modify ischemia-reperfusion injury
The high metabolic rate of brain cells implies a high baseline ROS production, and brain cells possess high concentrations of both enzymatic and small molecule antioxidant defenses. SOD1 may represent as much as 1% of total protein in brain; it converts O•−2 to H2O2, which is then further metabolized to water and oxygen by catalase and glutathione peroxidase. The SOD1 gene is located on chromosome 21 and codes for a 16- to 18-kDa subunit, which binds one Cu2+ and one Zn2+; the active enzyme is a homodimer. SOD2 is a homotetramer and the gene is on chromosome 6. An extracellular, glycosylated form, SOD3, has been shown in rodents to overlap in activity with SOD1. Several other enzymes unrelated to CuZn-SOD, for example, the atx1-HAH gene product, are also capable of acting as SODs. It remains to be determined to what extent SOD3 and these alternative dismutases complement SOD1 and SOD2 as antioxidant systems in humans.
Catalase and glutathione peroxidase provide two important cellular systems for eliminating H2O2. Catalase, a 56-kDa cytosolic hemeprotein homotetramer that can act without a cofactor, although it may bind NAD(P)H, functions as a peroxidase to convert H2O2 to water. It can be irreversibly inactivated by oxidation and demonstrates decreased activity after ischemia-reperfusion. Catalase is more abundant in astrocytes than in neurons and in white matter than in gray matter, but it can be induced in neurons by neurotrophins. There is substantially less catalase activity in brain than in other tissues, such as liver.
The human genes have been identified for four members of the glutathione peroxidase family of selenoproteins. Classical glutathione peroxidase (GPx1) is a complex of four 23-kDa subunits. Plasma GPx (GPx3), GPx2 and a fourth enzyme, phospholipid hydroperoxide glutathione peroxidase (PHGPx), are monomers with extensive homology to classical GPx1. All four enzymes contain one selenium atom per subunit in the form of a selenocysteine and all use glutathione (GSH) as a cofactor to convert H2O2 to water. PHGPx is unique in its ability to detoxify not only H2O2 but fatty acid and cholesterol lipid hydroperoxides directly. In addition, it has a cytosolic isoform and an isoform with a mitochondrial targeting sequence. Both GPx1 and PHGPx proteins are present in the brain. The components of the glutathione peroxidase system, GPx, GSH, glutathione reductase and NAD(P)H, are present in the mitochondria as well as the cytoplasm. Several other proteins, including glutathione S-transferase, may contribute minor peroxidase activity.
Small-molecule antioxidants include glutathione, ascorbic acid (vitamin C), vitamin E and a number of dietary flavenoids. Because humans, in contrast to most other animals, are unable to synthesize vitamin C, this important antioxidant must be supplied entirely from dietary intake (see Chap. 33). Other proteins, such as thioredoxin and metallothionine, may also contribute to some extent to the cellular antioxidant pool.
Reactive oxygen species may modify both the excitotoxic and the apoptotic components of ischemic brain damage
In addition to direct effects of oxidative injury during ischemia-reperfusion, ROS may modify ischemic excitotoxicity by downregulating current through NMDA receptors. However, exposure to oxidative stress can be expected to enhance NMDA receptor-mediated neurotoxicity by depleting intracellular antioxidant defenses. Free radicals also contribute to apoptosis at several points in the apoptotic cascade, serving as initiators, early signals and possibly late effectors of apoptotic neuronal death. It may be this interaction of injury mechanisms, including excitotoxicity, ischemic apoptosis, oxidative injury, inflammation and impaired metabolism, which, in part, makes the brain so vulnerable to ischemic damage.
- Oxygen free radicals are required intermediates in many biological reactions but may damage macromolecules during oxidative stress
- Reactive oxygen species generated during ischemia-reperfusion contribute to the injury
- Mitochondria, nitric oxide synthase and arachidonic acid metabolism are sources of reactive oxygen species during ischemia-reperfusion injury
- Nitric oxide and peroxynitrite contribute to oxidative damage
- Production of eicosanoids from polyunsaturated fatty acids such as arachidonic acid may generate reactive oxygen species
- The role of xanthine oxidase in human stroke is unclear
- Brain antioxidant defenses modify ischemia-reperfusion injury
- Reactive oxygen species may modify both the excitotoxic and the apoptotic components of ischemic brain damage
- Free Radicals in Hypoxia-Ischemia - Basic NeurochemistryFree Radicals in Hypoxia-Ischemia - Basic Neurochemistry
- GSM8172214[Accession] (3)GEO DataSets
Your browsing activity is empty.
Activity recording is turned off.
See more...