By agreement with the publisher, this book is accessible by the search feature, but cannot be browsed.
NCBI Bookshelf. A service of the National Library of Medicine, National Institutes of Health.
Siegel GJ, Agranoff BW, Albers RW, et al., editors. Basic Neurochemistry: Molecular, Cellular and Medical Aspects. 6th edition. Philadelphia: Lippincott-Raven; 1999.
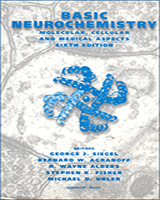
Basic Neurochemistry: Molecular, Cellular and Medical Aspects. 6th edition.
Show detailsLargely as a result of the studies of Langley, the possibility that biological tissues possess receptor molecules that are specific for each neurotransmitter was first entertained in the early 1900s. Langley noted the high degree of specificity and potency with which some agents elicit a biological response and postulated the existence of “receptor” or “acceptor” molecules. This concept has subsequently been fully validated. Many receptors have been isolated and purified biochemically, and many have also been cloned and sequenced. In several cases, the activity of purified receptors has been reconstituted in artificial systems.
Three phases of receptor-mediated signaling can be identified
The first is the binding component in which an extracellular ligand, usually a polar molecule, forms a complex with a cell-surface receptor, which can be localized on either a pre- or a postsynaptic membrane. The second phase is that in which the activated receptor—ligand complex elicits an increase in either the formation of second messengers, the opening or closing of ion channels or the recruitment of cytoplasmic proteins. The third phase typically involves the activation of enzymes, typically protein kinases or phosphatases, which mediate the biological response. Thus, the initial interaction of a ligand with a receptor results in amplification of the signal by means of a cascade of responses.
Four distinct molecular mechanisms that link agonist occupancy of cell-surface receptors to functional responses have been identified
Traditionally, receptors have been classified according to the mediator to which they respond. The first example, proposed by Sir Henry Dale in 1914, was that the neurotransmitter ACh can interact with two types of AChRs, termed either nicotinic or muscarinic AChRs based on the similarity of action of ACh to the plant alkaloids nicotine and muscarine. Similarly, Ahlquist proposed the division of adrenergic receptors into α and β subtypes, based on the potency of a series of natural and synthetic agonists to elicit a biological response. Although the pharmacological characterization of receptors provided a useful starting point, it rapidly became apparent that extensive subclassification of receptors would be required. For example, it is now recognized that there are both neural and non-neural forms of the nAChR, which can be distinguished pharmacologically and biochemically. In addition, multiple adrenergic receptor subtypes, including α1, α2, β1, β2 and β3, can be distinguished based on the ability of selective agonists and antagonists to bind to them. Although the pharmacological division of receptors remains that most commonly employed, an alternative classification is based on the effector mechanism to which receptors are linked.
This classification leads to four distinct groups based on the types of primary effector to which they couple [33] (Fig. 10-9). The first group is comprised of receptors which possess intrinsic ion channels that are composed of multiple subunits. Upon the binding of an agonist to these ligand-gated ion channels, the receptors undergo a conformational change which facilitates opening of the intrinsic ion channel. The permeability to specific ions is a characteristic of the receptor; for example, both the neuronal nAChR and NMDA receptors are selectively permeable to Na+ and Ca2+ ions, whereas GABAA and glycine receptors are primarily permeable to Cl− ions. As a result of the changes in ion conductance, the membrane potential may become either depolarized, as in nAChR or NMDA receptors, or hyperpolarized, as in GABAA or glycine receptors. Receptors in this category include those that are activated by synaptically released neurotransmitter and occur on the cell surface. The responses to these cell-surface receptors are extremely rapid, occurring in milliseconds, and do not require either the subsequent generation of second-messenger molecules or protein phosphorylation events. Thus, ligand-gated ion channels mediate “fast synaptic” transmission events in the CNS. The intracellular ligand-gated receptor for IP3 also opens within milliseconds upon binding of IP3 (see Chap. 21).

Figure 10-9
Cell-surface receptors utilize four distinct molecular mechanisms for transmembrane signaling. I: Ligand-gated ion channels. II: Receptors which possess intrinsic guanylyl cyclase activity. III: Receptors with intrinsic tyrosine kinase activity. IV: G (more...)
Receptors in the second group possess intrinsic guanylyl cyclase activity and generate cGMP upon receptor activation, for example, brain natriuretic peptide receptor. These receptors consist of an extracellular binding domain, a single transmembrane-spanning domain (TMD), a protein kinase-like domain and a guanylyl cyclase catalytic domain. Ligand binding results in a conformational change in the receptor and activation of the guanylyl cyclase catalytic region. Membrane-bound guanylyl cyclase activity does not require Ca2+ and can be modulated by ligand addition to cell-free preparations. A different, cytoplasmic form of guanylyl cyclase is activated by micromolar concentrations of Ca2+. Receptors with intrinsic guanylyl cyclase activity are often very highly phosphorylated in the absence of agonist and rapidly undergo dephosphorylation upon activation (see Chap. 24).
Receptors in the third group possess intrinsic receptor tyrosine kinase (RTK) activity. RTKs, such as epidermal growth factor receptor (EGFR) and platelet-derived growth factor receptor (PDGFR), are found in all multicellular eukaryotic organisms and are involved in the regulation of cellular growth and differentiation (see Chaps. 19 and 25). Structurally, RTKs possess an extracellular ligand-binding domain, a single TMD and an intracellular catalytic kinase domain. Three distinct events underlie signal transduction at RTKs. Initially, upon ligand binding to an RTK, the receptor undergoes a dimerization which results in the juxtaposition of the two cytoplasmic domains. Contact between these domains is thought to result in a stimulation of catalytic activity, which in turn results in an intermolecular autophosphorylation of tyrosine residues both within and outside of the kinase domain. The significance of the phosphorylation of tyrosine residues lies in the subsequent ability of the RTK to recruit cytoplasmic proteins which possess src homology 2 (SH2) domains. These regions of the molecule are 60 to 100 amino acids in length, are globular in structure, protrude from the surface of the protein and permit high-affinity protein—protein interactions to occur. In the case of the SH2 domain, a key arginine residue buried deep in a specific binding pocket interacts with the phosphate group of the tyrosine residue. The presence of an SH2 domain can increase the affinity of a peptide for a phosphorylated tyrosine residue by 1,000-fold. Once autophosphorylated, RTKs can recruit a number of cytoplasmic proteins and initiate a series of reactions involving protein—protein interactions. The best studied pathway of this type is the mitogen-activated protein kinase (MAPK) pathway. RTKs, via the recruitment of an adaptor protein complex such as growth factor receptor—binding protein 2 (Grb2)/son of sevenless (SOS) or SHC (see Chap. 25) can activate Ras, a low-molecular-weight, monomeric G protein. The role of Ras is to recruit and activate Raf (MAPKKK), a serine/ threonine kinase, which in turn activates MEK (MAPKK), a dual-specificity tyrosine/ threonine kinase. MEK subsequently activates MAPK, also known as ERK or extracellular signal—regulated kinase, which is a serine/threonine kinase with multiple substrates. ERK can enter the nucleus and regulate gene transcription by phosphorylating nuclear proteins (see Chap. 24).
The fourth group of receptors involves G proteins. Numerically, more diverse types of receptors have been demonstrated to operate via an intervening G protein than by any other mechanism. These G protein—coupled receptors (GPCRs), which have a characteristic seven TMD structure, can be further divided into three categories. Some GPCRs, such as GABAB, α2-adrenergic, D2-dopaminergic or muscarinic M2, regulate the changes in K+ conductance independently of second-messenger production (see Chap. 20). A second group of GPCRs is linked to the modulation of adenylyl cyclase activity. This regulation may be either positive, as in the case of activation of the β2-adrenergic receptor, or negative, as occurs following activation of the α2-adrenergic receptor. Changes in the concentrations of cAMP regulate the activity of protein kinase A (PKA) (Chap. 22). A third group of GPCRs is linked to the activation of PI-PLC with the attendant breakdown of PIP2 and formation of IP3 and DAG (see Chap. 21). These receptors are linked to changes in Ca2+ homeostasis and protein phosphorylation via the action of protein kinase C (PKC). Other effector enzymes that may be regulated by IP3-linked GPCRs include phospholipases A2 and D.
Cross-talk can occur between intracellular signaling pathways
Most cells possess receptors that operate through each of these four distinct effector mechanisms. Receptors within each class may be subject to further regulation during persistent agonist occupancy. One way that this can occur is via phosphorylation of the receptor, which results in its uncoupling from the effector enzyme, as has been demonstrated for the β-adrenergic receptor (see Chap. 12). This has been called homologous regulation. In addition, signaling pathways do not operate in isolation but may regulate and be regulated by one another, which has been termed heterologous regulation. In this way, the output of the cell is fine-tuned via subtle modulation of the relevant intracellular signaling mechanisms. There are numerous examples in which the activity of one receptor can regulate, either positively or negatively, the activity of a second; for example, increases in cAMP mediated by PKA can depress or potentiate PI-PLC activity (reviewed in [34]). Conversely, activation of PI-PLC can result in modulation of the activity of other pathways via the activation of PKC. An additional complexity is that the same ligand may activate multiple pathways in a given tissue. For example, NE can activate β2-adrenergic receptors, which increase adenylyl cyclase; α2-adrenergic receptors, which are coupled to inhibition of adenylyl cyclase; and α1-adrenergic receptors, which are linked to the activation of PI-PLC. Similarly, ACh can activate muscarinic cholinergic receptors, which either inhibit adenylyl cyclase, activate PI-PLC or activate K+ channels. In addition, ACh can directly activate nAChRs, which are linked to changes in Na+ and Ca2+ permeability. Thus, although individual signaling mechanisms are most frequently studied in isolation, their activity in vivo is likely to be highly regulated by other signal-transduction events.
A further consideration is that receptors which primarily activate one pathway may, on occasion, activate a second pathway (Fig. 10-10). An example is the ability of GPCRs, such as α2-adrenergic receptors or mAChRs, to activate the MAPK cascade. Activation of adenylyl cyclase-linked receptors results in the release of G protein βγ subunits, which, probably via an intermediary protein tyrosine kinase (PTK-X), stimulates phosphorylation of the adaptor protein SHC [35]. This in turn recruits the Grb2—SOS complex and activates the MAPK pathway.

Figure 10-10
Cross-talk between G protein—coupled receptors (GPCRs), ligand-gated ion channels (LGChs) and receptor protein tyrosine kinases (RTKs). GPCRs, via activation of PTK-X or PYK2, can activate the mitogen-activated protein kinase (MAPK) pathway. LGChs, (more...)
Activation of PI-PLC-linked receptors, such as the mAChR, results in increased PKC activity. Since the addition of phorbol esters, which are PKC agonists (Chap. 21), results in phosphorylation of Raf, this mechanism may provide an explanation for the ability of PI-PLC-coupled receptors to activate MAPK. A recently discovered protein tyrosine kinase PYK2, which is enriched in the CNS, is also activated by PKC. Like PTK-X, PYK2 phosphorylates SHC and recruits the Grb2—SOS complex, which results in activation of the MAPK cascade. PYK2 is also activated by Ca2+, raising the possibility that the activity of ligand-gated ion channels can also modulate the MAPK pathway. However, to date, there is no evidence to suggest that this regulation is reciprocal, that is, that activation of the MAPK pathway can modulate the activity of either GPCRs or ligand-gated ion channels.
Signaling molecules can activate gene transcription
In addition to their ability to elicit acute effects within cells, second-messenger molecules, such as cAMP, Ca2+ and DAG, can regulate gene transcription. The transcription factors cAMP response element—binding (CREB) protein, fos and jun, which respond to these signaling molecules, are members of the amphipathic helix family of proteins, and each contains a characteristic “leucine zipper,” which mediates dimerization (Chap. 26). The best studied of these transcription factors is CREB, which becomes phosphorylated on Ser133 in response to increases in the intracellular concentration of cAMP and subsequent activation of PKA. CREB can also be phosphorylated by Ca2+/calmodulin-dependent protein kinase IV. In its phosphorylated form, CREB binds to an eight-base cis-regulatory sequence called cAMP response element (CRE) and stimulates transcription. CREB has been implicated in long-term potentiation and memory (see Chap. 50). Increased concentrations of cytoplasmic Ca2+ and DAG activate PKC, which can also activate gene transcription via fos and jun. Activation of the MAPK pathway also leads to increased expression of jun and fos. Because increases in mRNA for fos and jun are observed very rapidly following a variety of stimuli, they have been termed immediate early genes. The fos—jun heterodimers, termed AP-1, bind to a seven-base pair cis-regulatory element called tetradecanoyl-phorbol acetate (TPA) response element (TRE). The latter has been demonstrated to control the transcription of several genes coding for neuropeptide modulators. Thus, despite major differences in the initial molecular mechanisms that underlie activation of RTKs or receptors coupled to either adenylyl cyclase or PI-PLC, these diverse groups of receptors share at least one common end point, that of gene regulation.
Nitric oxide acts as an intercellular signaling molecule in the central nervous system
Because cells rarely act in isolation, a signaling event in one cell may have a significant impact on the activity of neighboring cells. Initial evidence for intercellular, nonsynaptic signaling was obtained from experiments with blood vessels, in which it was observed that the addition of ACh increased cGMP concentrations and resulted in vasodilation and relaxation of the vascular smooth muscle. The increases in cGMP concentrations were dependent on the presence of Ca2+ and could not be demonstrated in tissue homogenates. A key observation was that removal of the endothelium abolished the relaxing effect of ACh, indicating the existence of an endothelium-derived relaxing factor (EDRF). Subsequently, it was discovered that EDRF was a short-lived gaseous molecule, nitric oxide (NO). NO is synthesized from arginine via the Ca2+ activation of nitric oxide synthase (NOS), an enzyme that requires NADPH as coenzyme and tetrahydrobiopterin as cofactor. Three distinct forms of NOS have been identified. An inducible form (iNOS), which is present in glia; a neuronal form (nNOS), which is widespread in distribution throughout the CNS; and an endothelial enzyme (eNOS) [36]. The activity of both nNOS and eNOS is Ca2+/calmodulin-dependent, whereas that of iNOS is Ca2+-insensitive. More recent evidence suggests that the activity of nNOS can be increased by brain lesions or ischemia (see Chap. 34). As a signaling molecule, NO differs from conventional neurotransmitters in that (i) it is not present in synaptic vesicles, (ii) it is not released by exocytosis and (iii) no specific extracellular synaptic receptors for NO exist. Rather, NO is released upon stimulation and diffuses from its site of production, which can be either neuronal or glial cells, to affect neurons up to 100 μm away. At these locations, NO activates a soluble form of guanylyl cyclase through electron transfer to the heme group on the enzyme. The increase in cGMP concentration subsequently activates cGMP-dependent protein kinases. In the CNS, NO has been speculated to play a role in both long-term potentiation (LTP) and long-term depression, although the precise mechanism remains to be defined. One counterargument for a role of NO in LTP is the observation that the latter persists in transgenic mice lacking nNOS. Recent evidence suggests that NO can also post-translationally modify sulfhydryl groups on synaptic vesicle proteins, thereby facilitating synaptic vesicle exocytosis [37].
It is conceivable that other gaseous molecules may also act as intercellular messengers. One putative candidate is carbon monoxide, which is generated from the conversion of heme to biliverdin, a reaction catalyzed by an oxygenase. One form of the enzyme, heme oxygenase-2, is constitutive and is found in high concentrations in various brain regions. Like NO, CO increases the production of cGMP.
Intercellular signaling may also occur via other mechanisms. For example, cell—cell propagation of Ca2+ signals can result from the gap junctional transfer of IP3 [38]. Alternatively, the release of humoral factors such as 5-HT, glutamate and nucleotides can initiate intercellular signaling. Thus, ATP and ADP released from human neuroepithelioma cells in response to a rise in the concentration of intracellular Ca2+ serves to further the propagation of intercellular Ca2+ signals [39]. Within the CNS, glutamate released from glia can activate receptors on neurons [40]. An additional consideration is that the secreted factor may act in an autocrine fashion. Thus, prostaglandins released from myenteric neurons in response to the addition of bradykinin can modulate the subsequent Ca2+ influx mediated by bradykinin receptors [40].
- Three phases of receptor-mediated signaling can be identified
- Four distinct molecular mechanisms that link agonist occupancy of cell-surface receptors to functional responses have been identified
- Cross-talk can occur between intracellular signaling pathways
- Signaling molecules can activate gene transcription
- Nitric oxide acts as an intercellular signaling molecule in the central nervous system
- Cellular Signaling Mechanisms - Basic NeurochemistryCellular Signaling Mechanisms - Basic Neurochemistry
Your browsing activity is empty.
Activity recording is turned off.
See more...