By agreement with the publisher, this book is accessible by the search feature, but cannot be browsed.
NCBI Bookshelf. A service of the National Library of Medicine, National Institutes of Health.
Siegel GJ, Agranoff BW, Albers RW, et al., editors. Basic Neurochemistry: Molecular, Cellular and Medical Aspects. 6th edition. Philadelphia: Lippincott-Raven; 1999.
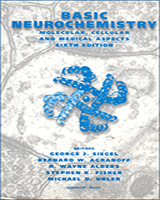
Basic Neurochemistry: Molecular, Cellular and Medical Aspects. 6th edition.
Show detailsNormally, the substrates are glucose and oxygen and the products are carbon dioxide and water
In contrast to most other tissues, which exhibit considerable flexibility with respect to the nature of the foodstuffs extracted and consumed from the blood, the normal brain is restricted almost exclusively to glucose as the substrate for its energy metabolism. Despite long and intensive efforts, the only incontrovertible and consistently positive arteriovenous differences demonstrated for the human brain under normal conditions have been for glucose and oxygen [16]. Negative arteriovenous differences, significantly different from zero, have been found consistently only for CO2, although water, which has never been measured, also is produced. Pyruvate and lactate production have been observed occasionally, certainly in aged subjects with cerebral vascular insufficiency but also irregularly in subjects with normal oxygenation of the brain.
In the normal in vivo state, glucose is the only significant substrate for energy metabolism in the brain. Under normal circumstances, no other potential energy-yielding substance has been found to be extracted from the blood in more than trivial amounts. The stoichiometry of glucose utilization and O2 consumption is summarized in Table 31-3. The normal, conscious human brain consumes oxygen at a rate of 156 μmol/100 g tissue/min. CO2 production is the same, leading to a respiratory quotient (RQ) of 1.0, further evidence that carbohydrate is the ultimate substrate for oxidative metabolism. O2 consumption and CO2 production are equivalent to a rate of glucose utilization of 26 μmol/100 g tissue/min, assuming 6 μmol of O2 consumed and of CO2 produced for each micromole of glucose completely oxidized to CO2 and H2O. The actual glucose utilization measured is, however, 31 μmol/100 g/min, which indicates that glucose consumption not only is sufficient to account for total O2 consumption but is in excess by 5 μmol/100 g/min. For complete oxidation of glucose, the theoretical ratio of O2: glucose utilization is 6.0; the excess glucose utilization is responsible for a measured ratio of only 5.5 μmol O2/μmol glucose. The fate of the excess glucose is unknown, but it probably is distributed in part in lactate, pyruvate and other intermediates of carbohydrate metabolism, each released from the brain into the blood in insufficient amounts to be detectable as significant arteriovenous differences. Some of the glucose must be utilized not for the production of energy but for synthesis of the chemical constituents of brain.
Table 31-3
Relationship Between Cerebral Oxygen Consumption and Glucose Utilization in a Normal Young Adult Man.
Some oxygen is used for oxidation of substances not derived from glucose, for example, in the synthesis and metabolic degradation of monoamine neurotransmitters, as mentioned above. The amount of O2 used for these processes is, however, extremely small and is undetectable in the presence of the enormous O2 consumption used for carbohydrate oxidation.
The combination of a cerebral RQ of unity, an almost stoichiometric relationship between O2 uptake and glucose consumption and the absence of any significant arteriovenous difference for any other energy-rich substrate is strong evidence that the brain normally derives its energy from the oxidation of glucose. Thus, cerebral metabolism is unique because no other tissue, except for testis [23], relies only on carbohydrate for energy.
This does not imply that the pathways of glucose metabolism in the brain lead, like combustion, directly and exclusively to production of CO2 and H2O. Various chemical and energy transformations occur between uptake of the primary substrates glucose and O2 and liberation of the end products CO2 and H2O. Compounds derived from glucose or produced through the energy made available from glucose catabolism are intermediates in the process. Glucose carbon is incorporated, for example, into amino acids, protein, lipids and glycogen. These are turned over and act as intermediates in the overall pathway from glucose to CO2 and H2O. There is clear evidence from studies with [14C]glucose that not all of the glucose is oxidized directly and that at any given moment some of the CO2 being produced is derived from sources other than glucose that enter the brain at the same moment or just prior to that moment. That O2 and glucose consumption and CO2 production are essentially in stoichiometric balance and that no other energy-laden substrate is taken from the blood means, however, that the net energy made available to the brain ultimately must be derived from the oxidation of glucose. It should be noted that this is the situation in the normal state; as discussed later, other substrates may be used in special circumstances or in abnormal states.
In brain, glucose utilization is obligatory
The brain normally derives almost all of its energy from the aerobic oxidation of glucose, but this does not distinguish between preferential and obligatory utilization of glucose. Most tissues are largely facultative in their choice of substrates and can use them interchangeably more or less in proportion to their availability. This does not appear to be so in the brain. Except in some unusual and very special circumstances, only the aerobic utilization of glucose is capable of providing the brain with sufficient energy to maintain normal function and structure. The brain appears to have almost no flexibility in its choice of substrates in vivo. This conclusion is derived from the following evidence.
Glucose deprivation is followed rapidly by aberrations of cerebral function. Hypoglycemia, produced by excessive insulin or occurring spontaneously in hepatic insufficiency, is associated with changes in mental state ranging from mild, subjective sensory disturbances to coma, the severity depending on both the degree and the duration of the hypoglycemia. The behavioral effects are paralleled by abnormalities in EEG patterns and cerebral metabolic rate. The EEG pattern exhibits increased prominence of slow, high-voltage δ rhythms, and the rate of cerebral oxygen consumption falls. In studies of the effects of insulin hypoglycemia in humans [24], it was observed that when arterial glucose fell from a normal concentration of 70 to 100 mg/100 ml to an average of 19 mg/100 ml, subjects became confused and cerebral oxygen consumption fell to 2.6 ml/100 g/min, or 79% of normal. When arterial glucose fell to 8 mg/100 ml, a deep coma ensued and cerebral O2 consumption decreased even further to 1.9 ml/100 g/min (Table 31-4).
Table 31-4
Effects of Insulin Hypoglycemia on Cerebral Circulation and Metabolism in Humans a.
These changes are not caused by insufficient cerebral blood flow, which actually increases slightly during coma. In the depths of coma, when the blood glucose content is very low, there is almost no measurable cerebral uptake of glucose from the blood. Cerebral O2 consumption, although reduced, is still far from negligible; and there is no longer any stoichiometric relationship between glucose and O2 uptakes by the brain, evidence that the O2 is utilized for the oxidation of other substances. The cerebral RQ remains approximately 1, however, indicating that these other substrates are still carbohydrate, presumably derived from the endogenous carbohydrate stores of the brain. The effects are clearly the result of hypoglycemia and not of insulin in the brain. In all cases, the behavioral, functional and cerebral metabolic abnormalities associated with insulin hypoglycemia are reversed rapidly and completely by the administration of glucose. The severity of the effects is correlated with the degree of hypoglycemia and not the insulin dosage, and the effects of insulin can be prevented completely by the simultaneous administration of glucose with the insulin.
Similar effects are observed in hypoglycemia produced by other means, such as hepatectomy. Inhibition of glucose utilization at the phosphohexose isomerase step with pharmacological doses of 2-deoxyglucose also produces all of the cerebral effects of hypoglycemia despite an associated elevation in blood glucose content.
In hypoglycemia, substrates other than glucose may be utilized. The hypoglycemic state provides convenient test conditions to determine whether a substance is capable of substituting for glucose as a substrate of cerebral energy metabolism. If it can, its administration during hypoglycemic shock should restore consciousness and normal cerebral electrical activity without raising the blood glucose concentration. Numerous potential substrates have been tested in humans and animals. Very few can restore normal cerebral function in hypoglycemia, and of these all but one appear to operate through a variety of mechanisms to raise the blood glucose concentration rather than by serving directly as a substrate (Table 31-5).
Table 31-5
Effectiveness of Various Substances in Preventing or Reversing the Effects of Hypoglycemia or Glucose Deprivation on Cerebral Function and Metabolisma.
Mannose appears to be the only substance that can be utilized by the brain directly and rapidly enough to restore or maintain normal function in the absence of glucose [25]. It traverses the BBB and is converted to mannose-6-phosphate. This reaction is catalyzed by hexokinase as effectively as the phosphorylation of glucose. The mannose-6-phosphate is then converted to fructose-6-phosphate by phosphomannose isomerase, which is active in brain tissue. Through these reactions mannose can enter directly into the glycolytic pathway and replace glucose.
Maltose also is effective occasionally in restoring normal behavior and EEG activity in hypoglycemia but only by raising the blood glucose concentration through its conversion to glucose by maltase activity in blood and other tissues [16]. Epinephrine is effective at producing arousal from insulin coma, but this is achieved through its well-known stimulation of glycogenolysis and the elevation of blood glucose concentration. Glutamate, arginine, glycine, GABA and succinate also act through adrenergic effects that raise glucose concentrations of the blood [16].
It should be noted, however, that failure to restore normal cerebral function in hypoglycemia is not synonymous with an inability of the brain to utilize the substance. Many of the substances that have been tested and found ineffective are compounds normally formed and utilized within the brain and are normal intermediates in its intermediary metabolism. Lactate; pyruvate; fructose-1,6-bisphosphate; acetate; β-hydroxybutyrate; and acetoacetate can be utilized by brain slices, homogenates or cell-free fractions; and the enzymes for their metabolism are present in brain. Enzymes for the metabolism of glycerol or ethanol, for example, may not be present in sufficient amounts. For other substrates, for example, d-β-hydroxybutyrate and acetoacetate, the enzymes are adequate but the substrate is not available to the brain because of inadequate blood levels or restricted transport through the BBB.
Nevertheless, nervous system function in the intact animal depends on substrates supplied by the blood, and no satisfactory, normal, endogenous substitute for glucose has been found. Glucose, therefore, must be considered essential for normal physiological behavior of the central nervous system.
The brain utilizes ketones in states of ketosis
In special circumstances, the brain may fulfill its nutritional needs partly, although not completely, with substrates other than glucose. Normally, there are no significant cerebral arteriovenous differences for d-β-hydroxybutyrate and acetoacetate, which are “ketone bodies” formed in the course of the catabolism of fatty acids by liver. Owen and coworkers [26] observed, however, that when human patients were treated for severe obesity by complete fasting for several weeks, there was considerable uptake of both substances by the brain. If one assumed that the substances were oxidized completely, their rates of utilization would have accounted for more than 50% of the total cerebral oxygen consumption, more than that accounted for by the glucose uptake. d-β-hydroxybutyrate uptake was several times greater than that of acetoacetate, a reflection of its higher concentration in the blood. The enzymes responsible for their metabolism, d-β-hydroxybutyrate dehydrogenase, acetoacetate-succinyl-CoA transferase and acetoacetyl-CoA-thiolase, are present in brain tissue in sufficient amounts to convert them into acyl-CoA and to feed them into the tricarboxylic acid cycle at a sufficient rate to satisfy the metabolic demands of the brain [27].
Under normal circumstances, that is, ample glucose and few ketone bodies in the blood, the brain apparently does not oxidize ketones in any significant amounts. In prolonged starvation, the carbohydrate stores of the body are exhausted and the rate of gluconeogenesis is insufficient to provide glucose fast enough to meet the requirements of the brain; blood ketone concentrations rise as a result of the rapid fat catabolism. The brain then apparently turns to the ketone bodies as the source of its energy supply.
Cerebral utilization of ketone bodies appears to follow passively their concentrations in arterial blood [27]. In normal adults, ketone concentrations are very low in blood and cerebral utilization of ketones is negligible. In ketotic states resulting from starvation; fat-feeding or ketogenic diets; diabetes; or any other condition that accelerates the mobilization and catabolism of fat, cerebral utilization of ketones is increased more or less in direct proportion to the degree of ketosis [27]. Significant utilization of ketone bodies by the brain is, however, normal in the neonatal period. The newborn infant tends to be hypoglycemic but becomes ketotic when it begins to nurse because of the high fat content of the mother's milk. When weaned onto the normal, relatively high-carbohydrate diet, the ketosis and cerebral ketone utilization disappear. Studies have been carried out mainly in the infant rat, but there is evidence that the situation is similar in the human infant.
The first two enzymes in the pathway of ketone utilization are d-β-hydroxybutyrate dehydrogenase and acetoacetyl-succinyl-CoA transferase. These exhibit a postnatal pattern of development that is well adapted to the nutritional demands of the brain. At birth, the activity of these enzymes in the brain is low; activity rises rapidly with the ketosis that develops with the onset of suckling, reaches its peak just before weaning and then gradually declines after weaning to normal adult rates of approximately one-third to one-fourth the maximal rates attained [27,28].
It should be noted that d-β-hydroxybutyrate is incapable of maintaining or restoring normal cerebral function in the absence of glucose in the blood. This suggests that, although it can partially replace glucose, it cannot fully satisfy the cerebral energy needs in the absence of some glucose consumption. One explanation may be that the first product of d-β-hydroxybutyrate oxidation, acetoacetate, is metabolized further by its displacement of the succinyl moiety of succinyl-CoA to form acetoacetyl-CoA. A certain rate of glucose utilization may be essential to drive the tricarboxylic cycle, to provide enough succinyl-CoA to permit the further oxidation of acetoacetate and, hence, to pull along the oxidation of d-β-hydroxybutyrate.
- Substrates of Cerebral Metabolism - Basic NeurochemistrySubstrates of Cerebral Metabolism - Basic Neurochemistry
Your browsing activity is empty.
Activity recording is turned off.
See more...