By agreement with the publisher, this book is accessible by the search feature, but cannot be browsed.
NCBI Bookshelf. A service of the National Library of Medicine, National Institutes of Health.
Siegel GJ, Agranoff BW, Albers RW, et al., editors. Basic Neurochemistry: Molecular, Cellular and Medical Aspects. 6th edition. Philadelphia: Lippincott-Raven; 1999.
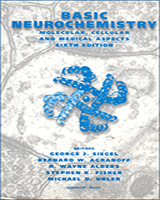
Basic Neurochemistry: Molecular, Cellular and Medical Aspects. 6th edition.
Show detailsSecondary active-transport systems mediate diverse neural functions
A variety of molecules and ions are regulated by specific symporters or antiporters linked to the Na+ gradient (Fig. 5-1). Examples are discussed in the following sections.
The clearance of amine and amino acid neurotransmitters from the synaptic cleft and their storage in cytoplasmic vesicles is accomplished by the tandem action of plasma-membrane and vesicular-transport systems. Symporters in the plasma membranes of neurons and glia mediate neurotransmitter reuptake from synaptic clefts, whereas antiporters function in neurons to concentrate neurotransmitters from neuronal cytoplasm into synaptic vesicles (Fig. 5-7).

Figure 5-7
Processes involved in neurotransmitter uptake at nerve endings. Concentration into the cytoplasm is achieved by means of a Na+ symporter with high affinity for the neurotransmitter. Synaptic vesicle membranes contain an ATP-dependent H+ pump and Cl− (more...)
Neurotransmitter symporters. All neurotransmitter reuptake is driven by the Na+ gradient created by the Na+, K+ pump. Symporters have been identified and partially characterized for the major neurotransmitters. While their demands on cerebral energy metabolism are unknown, it has been proposed that they could contribute substantially to the increased metabolism associated with neuronal activity (Chap. 31).
There are two distinct subfamilies of Na+-dependent symporters that function in plasma membranes. One subfamily includes the (Na+ + Cl−)-dependent transporters for GABA, glycine, norepinephrine, dopamine and serotonin. Their topology includes 12 traverses of the plasma membrane. The other subfamily includes the (Na+ + Cl− + K+)-dependent glutamate transporters, which traverse the plasma membrane ten times.
Five glutamate-symporter isoforms have been identified in human brain. Two of these isoforms, glutamate—aspartate transporter (GLAST) and glutamate transporter-1 (GLT-1) (Chap. 15), are selectively expressed in astrocytes, while others display selective localization in certain neuronal cell types. The different isoforms probably have different regulatory interactions. For example, two of them have been shown to respond oppositely to exposure to arachidonic acid [33]. Astrocytes appear to play an important role in glutamate clearance from synaptic clefts. Rendering mice [34] and rats [35] deficient in GLAST or GLT-1 produces elevated extracellular glutamate concentrations and leads, in the case of mice, to lethal seizures.
Glutamate symporters have a relatively complex ion requirement, which has been investigated in a study of glutamate uptake by salamander retinal glia: one glutamate and two Na+ are taken into the cell in exchange for the extrusion of one K+, HCO−3 or OH−. Another study has concluded that three Na+ enter with each glutamate [36]. In either case, there will be a net depolarizing influx of positive charge. The anion efflux produces intracellular acidification and extracellular alkalinization. The regulation of glutamate concentration is of special interest because the accumulation of extracellular glutamate is a probable factor in various pathologies (Chap. 34). While brain glutamate concentration is about 10 mM, “extracellular” glutamate as measured by in vivo microdialysis is normally only 3 to 4 μM. Glutamate within the synaptic cleft must be maintained at still lower concentrations in the resting state. With “normal” membrane potentials and ion concentrations, the glutamate transporter can theoretically reduce this value to 0.6 μM or less. However, under depolarizing or anoxic conditions, the membrane potential and the Na+ and K+ gradients can be reduced to levels that cause the symporter to fail or to operate in reverse to produce 100- to 1,000-fold increases in extracellular glutamate. Glutamate accumulated by astrocytes is converted to glutamine, which is, at least partly, shunted back into neurons.
Studies on the mechanisms of secondary transport. Voltage-clamp techniques (Chap. 6) can provide some insights into the mechanisms of ion-coupled secondary transport. Charge movements that are direct reflections of transport events can be detected in voltage-clamped membranes containing transport proteins. For example, capacitative currents occur in Xenopus oocyte membranes containing GABA transporters when the external medium is rapidly changed between Na+-containing and Na+-free Ringer's solutions. These currents can be related to transport because they are suppressed by specific GABA transport-blocking agents. Several deductions regarding the transport mechanism are inferred from such measurements: the apparent affinity of the transport sites for Na+ is voltage-dependent; the amount of charge movement is a cooperative function of [Na+], indicative of two Na+-binding sites per transporter; external Cl− increases Na+ affinity without changing the capacitative current. These are all events that involve the extracellularly available transporter domains when measured in the absence of external GABA. Accordingly, they are considered to be “priming reactions” that prepare the transporter to rapidly bind and remove GABA as it appears in a synaptic cleft [37].
Ionic currents through transporters have been observed in the presence of neurotransmitters but in the absence of energizing ion gradients. Voltage-clamp recordings of several of the neurotransmitter symporters have exhibited brief, 2 to 3 msec, electrical events that are comparable to discrete ion-channel openings. For example, serotonin symporters exhibit Na+ conductances of 6 to 13 pS, whereas glutamate symporters exhibit Cl− conductances of ~0.7 pS. In both cases, these channel-like events can be blocked by selective transport inhibitors and they occur at much lower frequencies than the turnover rates of the symporters. Although their physiological relevance is unknown, they are undoubtedly associated with these ion-coupled symporters [38].
Vesicle neurotransmitter antiporters. As discussed above, proton-dependent antiporters concentrate neurotransmitters into presynaptic vesicles (Fig. 5-7). Protons are transported into the vesicles by a vacuolar-type ATPase, as discussed above.
Two closely related vesicular membrane antiporters (VMAT), VMAT1 and VMAT2 (Chap. 12), are broadly selective for the monoamine neurotransmitters dopamine, norepinephrine and serotonin. VMAT1 is expressed in peripheral neuroendocrine cells, VMAT2 in neurons and both in adrenal chromaffin cells [39]. VMAT2 expression is restricted to the membranes of dense-core vesicles. It also transports histamine and is expressed in histamine-storing cells. Selective inhibitors of both VMAT1 and VMAT2 include reserpine and ketanserin, whereas tetrabenazine inhibits only VMAT2. The related vesicular acetylcholine transporter (VAChT) is present in small cholinergic synaptic vesicles of the CNS. The membrane topology of all of these synaptic vesicle antiporters seems to consists of 12 transmembrane segments, beginning and ending on the cytoplasmic side.
Uptake of neurotransmitters into synaptic vesicles can be blocked by nigericin, by proton ionophores or by other agents which dissipate the proton gradient and by N-ethylmaleimide, which inhibits V-type ATPases. Uptake into synaptic vesicles is not affected by agents that act on other transport systems, such as Na+, Ca2+, ouabain, vanadate or oligomycin. A low concentration of extravesicular Cl− stimulates the uptake of glutamate and catecholamines into their respective vesicles but does not affect glycine or GABA vesicular uptake.
Na+, H+ antiporters. Five isoforms of mammalian Na+, H+ antiporters (NHE) have been identified that catalyze an electroneutral exchange of protons for Na+. The membrane topology is similar in all isoforms and includes ten to twelve putative transmembrane segments within the first 500 N-terminal residues. This fragment has been shown to be sufficient to mediate exchange of protons for Na+. The more variable C-terminal cytoplasmic segment of about 300 residues appears to contain isoform-dependent regulatory domains. NHE1 is the most widely distributed and intensively studied isoform. It can be activated by a variety of stimuli, including neurotransmitters, hormones, growth factors and cell volume decrease. These factors act through second messengers to increase the affinity for protons, resulting in cytoplasmic alkalinization. Functions ascribed to Na+, H+ antiporters include roles in pH and volume regulation, cell adhesion, cell proliferation and ion and water transport. The NHE1 and NHE3 isoforms are expressed in the basolateral and apical membranes, respectively, of epithelia. The NHE5 isoform is primarily expressed in brain, spleen and testes.
Na+, Ca2+ antiporters are present in plasma membranes and endoplasmic reticulum and act in concert with ATP-coupled Ca2+ pumps. The exchange process is electrogenic since the stoichiometry is three Na+ per Ca2+. The antiporters have lower affinities for Ca2+ than the P-type pumps, but because in some tissues the antiporter pumping capacity may be up to ten times that of the Ca2+-ATPases, they may be important for rapidly lowering high cytoplasmic Ca2+.
Anion Antiporters. Because CNS energy production derives almost entirely from aerobic glycolysis, the rate of metabolic CO2 production is essentially equal to the rate of oxygen consumption. In adult human brain, this is about 2.5 mM per minute (Chap. 31). Most neurons express only low concentrations of carbonic anhydrase, and most carbonic anhydrase in brain is non-neuronal. Thus, most CO2 diffuses unhydrated out of neurons and is rapidly converted to HCO−3 before it enters the blood. A major component of erythrocytes is a Cl−/HCO−3 antiporter, known as band 3 protein, which mediates rapid uptake of HCO−3 in exchange for Cl− in peripheral tissues and releases HCO−3 in exchange for Cl− in the lungs. A neuron-specific isoform of the Cl−/HCO−3 antiporter has been identified, which suggests that significant anion exchange may occur across neuronal and/or glial membranes. Since some neurons express significant concentrations of cytoplasmic carbonic anhydrase, Cl−/HCO−3 exchange may have unrecognized functions. Its operation in neurons could influence both Cl− potentials and cytoplasmic pH.
Rapid clearance of K+ from the extracellular space is critical because high extracellular K+ depolarizes neurons
Neural activity discharges K+ into the extraneuronal space. If not rapidly removed, this K+ lowers the membrane potential of adjacent cells. Normal neuronal activity can lead to elevations of 1 to 3 mM in extracellular K+ ([K+]e), and during epileptogenesis, concentrations can be three to four times higher. Usually, the upper limit of axonal impulse-conduction frequency is set by a conduction block that occurs when extracellular K+ accumulates to a concentration of 25 to 30 mM (Chap. 37). Both neurons and astroglia are involved in K+ uptake, and both active and passive transport are probably important. The relative contributions of the two cell types and of the two cell processes remain controversial. It is clear that neurons accumulate K+ almost exclusively by means of active transport, but the Na+,K+ pump is slow relative to the channel-mediated K+ release from neurons and to the rates of K+ increase in the extracellular space. Moreover, the neuronal Na+,K+ pump is saturated at low [K+]e, making it an ineffective regulator for this purpose.
Glial processes invest nearly all extrasynaptic neuronal surfaces (Chap. 1), and one of their principal functions is to regulate [K+]e. The extracellular space of the brain consists primarily of the 150 to 250 Å clefts separating glia and neurons. Astrocytes have higher K+ permeability than most neurons, and they are extensively interconnected by gap junctions (Chap. 2). K+ efflux from a neuron can diffuse into the local glial cytoplasm, and compensatory K+ efflux can occur from more distal reaches of the glial syncytium, where the [K+]e is lower (Fig. 5-8A). It should be noted that this “spatial buffering” is a diffusion process that requires no energy input. Measurements on retinal Müller cells have provided relevant data. These cells are specialized forms of astrocytes which do not form syncytia. Rather, they extend from the deep layers of the retina to form endfeet at the retinal interface with the vitreous body (see Fig. 47-1). The K+ conductance of these cells is about 30 times higher at the endfeet in contact with the vitreous fluid than elsewhere. Moreover, K+ currents through the plasma membranes other than endfeet are carried by inward-rectifying channels, whereas the K+ channels at the endfeet are high-conductance and non-rectifying [40]. This seems designed to facilitate diffusive currents of K+ from the retina into the Müller cell, which are balanced by K+ efflux into the vitreous space and constitute a relatively “infinite” sink, equivalent to the syncytium of the generalized astrocytes.

Figure 5-8
A: Spatial buffering by astrocytes. This conceptual diagram indicates the pathways available for potassium ions to diffuse through the glial syncytium (light orange) subsequent to their release from neuronal membranes (dark orange) during neural activity. (more...)
Although spatial buffering can effectively prevent accumulation of moderate concentrations of extracellular K+, this diffusion process may be inadequate at very low and very high rates of neuronal K+ efflux. Several K+ transporters occur in astrocytes and probably broaden the range of effective [K+]e regulation. Glial swelling accompanies neural activity. This reflects the operation of a (Na+,K+,2Cl−) symporter in response to the volume-regulation mechanism discussed below. This symporter is driven by the Na+ gradient generated by the Na+ pump and may clear extracellular K+ when an adequate K+ sink is not present.
An outwardly directed Cl− pump is necessary for the inhibitory, that is, hyperpolarizing, functions of GABA and glycine-gated ion channels
The principal inhibitory neurotransmitters, GABA and glycine, hyperpolarize mature neurons by opening Cl− channels (Chap. 16). This occurs because their internal Cl− concentration is maintained at less than its equilibrium value and Cl− flows inward. The most likely mechanism for reducing internal Cl− involves a KCl symporter, a neuron-specific isoform of which occurs in brain [41]. Although indirect evidence for ATP-dependent primary Cl− transport has been reported, a corresponding ATPase protein has not been identified [42]. The importance of an outward Cl− pump for the inhibitory function of GABA-gated channels is made evident by observations that at early developmental stages GABA-gated channels in cortical neurons are depolarizing. They become hyperpolarizing only with maturation as a Cl− transport system appears and the internal Cl− concentration decreases [43].
Intracellular pH in brain is regulated by Na+,H+ antiporters, anion antiporters and Na+, HCO−3 symporters
The products of cerebral energy metabolism, primarily water and CO2, could in principle leave the brain and enter the circulation without disturbing the ionic balance. However, to the extent that oxidation is incomplete, that is, to the extent that lactate or ketoacids are produced (Chap. 31), or that CO2 equilibrates to H+ + HCO−3, local pH will decrease. Other processes, such as local influx of Ca2+, can also produce local pH changes. The cerebral metabolic rate is sensitive to such changes and decreases with decreasing pH. Without adequate pH control, local metabolic deficits can be intensified and propagated [44]. In addition to the increase of [K+]e discussed above, intracellular acidification and extracellular alkalinization accompany neuronal activity [45]. The pH response of astrocytes to membrane depolarization is opposite to the neuronal pH response: intracellular alkalinization and extracellular acidification occur [46]. The neuronal acidification appears to involve the anion antiporter exchange of HCO−3 for Cl−, whereas astrocytic alkalinization is probably mediated by a Na+, HCO−3 symporter. This is an oversimplification of CNS pH regulation in view of the fact that additional secondary transporters are involved in various aspects of local pH regulation.
Na+, H+ antiporters occur in synaptosomes, glia and neuroblastoma cells (Fig. 5-8B). They are relatively inactive at neutral pH, but with a decrease in intracellular pH they produce an efflux of protons at the expense of the Na+ gradient. The Na+, H+ transport stoichiometry is 1:1. Activation by an internal pH decrement apparently results from protonation of a cytoplasmic site, which allosterically increases the affinity of the proton ionophoric site. In some cells, the Na+, H+ antiporter is under additional control by receptor mechanisms. Several growth factors and hormones produce transient cytoplasmic alkalinization, probably by mediating a protein kinase phosphorylation of the antiporter, which increases its internal proton affinity.
Cell-volume regulation involves control of the content of osmotically active impermeant molecules and ions
Cell volume will change because of an altered internal solute content, termed isotonic response, or because of altered extracellular solute concentration, termed hyper- or hypotonic responses (Fig. 5-8B). Impairment of brain energy metabolism, such as may ensue from ischemia, anoxia or trauma, produces an isotonic cellular swelling called “cytotoxic edema” and further effects by the coincident release of glutamate (Chaps. 34 and 38). Cellular swelling is also produced by extracellular hypotonicity. This is most often caused by hyponatremia, which can result acutely from postsurgical fluid therapies. Chronic hyponatremia can arise from congestive heart failure, Addison's disease or a “syndrome of inappropriate vasopressin release.” A general reduction in brain size resulting from acute extracellular hypertonicity may produce neurological disturbances. This condition may be a component of pathology originating outside the brain, such as kidney disease (uremia) and diabetes mellitus (hyperglycemia). Central pontine myelinolysis, which is produced experimentally by acute infusion of hypertonic saline, can occur as a complication of liver transplantation.
Cells have short-term mechanisms that tend to restore their volume toward “normal” primarily by shifting the distributions of Na+, K+ and Cl−. The details of the mechanisms appear to vary with cell type. Subsequent to cell swelling, regulatory volume decrease usually involves opening ion channels. Subsequent to hyperosmotic shrinking, regulatory volume increase frequently involves activation of Na+, H+ antiporters and Na+, K+, 2Cl− symporters. The primary detectors of volume and osmotic changes are presently unknown. Although cell membranes can readily change shape, they do not stretch significantly. However, cytoskeletal proteins attached to membrane proteins could form tensile elements that could mediate volume changes (Fig. 5-8B). Cell shape is determined by interactions of cytoskeletal elements, membranes and components of the extracellular matrix (Chaps. 2 and 8). Stretch-activated cation channels have been detected both in neuroblastoma cells and in cultured astrocytes (see Box 47-1). Activation of Cl− channels by cellular swelling is an identified characteristic of both the MDR and CFTR proteins [47], at least one of which occurs in astrocytes.
Altered extracellular tonicity can induce compensatory changes in the concentrations of certain intracellular osmolytes through mechanisms involving gene regulation. Glioma cells in culture have been shown to respond after a few hours of exposure to hypertonic medium by transcribing high levels of the Na+, inositol symporter. In brain, active osmolytes include taurine, glutamine, glutamate, myo-inositol, creatine, glycerophosphorylcholine and choline. Na+-dependent symporters for most of these compounds have been identified [48].
Unclassified energy-requiring pumps. Three different but closely related mammalian proteins, called ZnT-1, -2 and -3, have been related to Zn2+ transport. The best evidence indicates that ZnT-1 mediates Zn2+ efflux across cell membranes, whereas ZnT-2 mediates vesicular sequestration of Zn2+. ZnT-3 is selectively expressed in brain and testes. In brain it may be associated with presynaptic vesicles in certain glutamatergic neurons [49]. The ZnT proteins are structurally related to a family of yeast proteins that confer resistance to Zn2+ and Co2+ toxicity. At present, there is no information about the energy source for Zn2+ transport in eukaryotes. An E. coli Zn2+-resistance gene has been shown to encode a P-type ATPase.
- Secondary active-transport systems mediate diverse neural functions
- Rapid clearance of K+ from the extracellular space is critical because high extracellular K+ depolarizes neurons
- An outwardly directed Cl− pump is necessary for the inhibitory, that is, hyperpolarizing, functions of GABA and glycine-gated ion channels
- Intracellular pH in brain is regulated by Na+,H+ antiporters, anion antiporters and Na+, HCO−3 symporters
- Cell-volume regulation involves control of the content of osmotically active impermeant molecules and ions
- Secondary Transport Systems - Basic NeurochemistrySecondary Transport Systems - Basic Neurochemistry
Your browsing activity is empty.
Activity recording is turned off.
See more...