NCBI Bookshelf. A service of the National Library of Medicine, National Institutes of Health.
Ackerman S. Discovering the Brain. Washington (DC): National Academies Press (US); 1992.
The making of the human brain from the tip of a 3 millimeter neural tube is a marvel of biological engineering. To arrive at the more than 100 billion neurons that are the normal complement of a newborn baby, the brain must grow at the rate of about 250,000 nerve cells per minute, on average, throughout the course of pregnancy. But it is not the volume of growth alone that makes the production of a human brain staggering to consider. The great number of functions that the brain reliably carries out and the specificity with which these are assigned to one or another type of cell or small location in the whole assembly are stunning in their complexity; yet the feat of growing a human brain occurs in hundreds of millions of individuals each year. The brain's 100 trillion or so interconnections provide the physical basis for its speed and sophistication. But how is such an intricate network constructed in the first place? Does the genetic material of the fertilized egg already contain a full set of building specifications for the human brain, in which every cell is created as a minute increment in the overall design? And if the set of instructions is indeed so closed and specific, how could chance or random mutations or the influence of the environment have played a role—as they so evidently have done and continue to do—in the emergence of the first human brains?
From these questions, it is easy to see that any scientific account of the development of the human brain has to meet a formidable challenge. For such an account must not only explain a sequence of development of great orderliness and efficacy but also allow room for the creative effects of chance—in the form of random mutations and the ensuing natural selection—that have led to the propagation of this particular form of brain in the first place. The majority of developmental neuroscientists today respond to this challenge by proposing a series of stages in which built-in instructions and the effects of arbitrary external events are mingled to an intriguing degree.
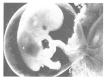
FIGURE 6.1.
The development of the human brain during gestation is a highly complex project on a tight schedule. In this 12- to 14-week-old embryo, nerve cells are proliferating at the rate of about 15 million per hour. The physical bases for perception are beginning (more...)
According to this scheme, the essential stages are (1) proliferation of a vast number of undifferentiated brain cells; (2) migration of the cells toward a predetermined location in the brain and the beginning of their differentiation into the specific type of cell appropriate to that location; (3) aggregation of similar types of cells into distinct regions; (4) formation of innumerable connections among neurons, both within and across regions; and (5) competition among these connections, which results in the selective elimination of many and the stabilization of the 100 trillion or so that remain. These events do not occur in rigid sequence but overlap in time, from about 5 weeks after conception onward. After about 18 months of age, no more neurons are added, and the aggregation of cell types into distinct regions is roughly complete. But the pruning of excess connections—clearly a process of great importance for the shape of the mature brain—continues for years.
This model of the sequence of brain development has led toward many fruitful lines of investigation in neuroscience. Among other things, it can explain well-known birth defects of the brain or the nervous system in terms of the stage at which development was disturbed. If, for instance, at a very early stage the neural tube fails to close properly, the cells that should form the forebrain and its overlying skull and scalp may not be generated; this condition, anencephaly (''without brain"), almost always results in stillbirth or in survival for only a few hours. Less severe defects of the neural tube may give rise to varying degrees of spina bifida ("split spine"), with the spinal column missing the bony protection of some of its vertebrae. Exposure to x-rays, high levels of alcohol, and some drugs can impair development at crucial early stages, as can the mother's infection with certain diseases such as rubella (German measles).
At about the midpoint of pregnancy, from about 15 to 20 weeks after conception, the number of brain cells in the cerebral cortex increases rapidly; by the seventh month, the fetus is emitting its own brain waves, which can be detected through the mother's abdomen. Several lines of evidence suggest that proper nutrition is of greatest importance for the development of the brain at this stage, although it continues to be crucial until birth and for some time afterward. Yet even when the developing brain suffers environmental insults such as malnutrition, it shows a remarkable capacity to recover and go on to develop normally, provided the harmful circumstances are corrected within the first 3 months or so after birth.
One of the problems facing neuropathologists interested in congenital neurological defects is that they usually examine the brain long after the abnormal events have passed. Another problem is that the "normal" data in this area may cover a wide range of variation. A basic understanding of normal brain development is essential for identifying and addressing those factors that can interfere with the making of a healthy brain.
Studying the Brain in Development
Short of technology that would shrink a remote video camera down to the size of a cellular implant, how can neuroscientists study brain development as an ongoing process in the living animal? The multistage model is useful here, too, as it was in explaining various brain disorders, because it suggests several points at which researchers can alter the process of development in a highly controlled way and learn a great deal by observing the outcome. Currently, three techniques are working especially well for researchers who want to know more about the making of the cerebral cortex, in particular. One technique is based on the knowledge that all the cells destined to become part of the cerebral cortex are first generated near the center of the brain, at the fluid-filled ventricles that have formed from the original three bulges in the neural tube. If a small number of ventricular cells are removed and labeled with various neutral dyes, then reinjected while cell proliferation is still going on, it is possible to follow a particular group of dyed cells as they migrate to their eventual positions in the cortex. So far, this approach has suggested that a cell may already contain the information of its eventual "address" in the cortex when it is generated; for example, a cell that is removed when the ventricle is producing layer 3 of the cortex and then injected back into the ventricle during the generation of layer 4 will nonetheless migrate to layer 3. Hence, genetic information may strongly control this aspect of development.
A second technique works at the genetic level by inserting an innocuous retrovirus into ventricular cells. The retrovirus does not affect normal functioning, but its genetic information is incorporated into the living cells' DNA and faithfully replicated in the cells of future generations. The genetic code of the retrovirus can thus be used as a marker, much as dyes were used in the preceding technique. Here, though, the technique allows an investigator to observe successive generations of cells rather than the spatial distribution of cells that are all from one generation.
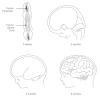
FIGURE 6.2.
The human brain develops from the tip of a 3-millimeter-long neural tube. At three to four weeks after conception, the neural groove closes into a tube, and three distinct regions—a hindbrain, midbrain, and forebrain—begin to take form. (more...)
The third technique gives dramatic results by knocking out one generation of cells altogether. For example, exposing a pregnant monkey to x-ray irradiation at a particular point in its pregnancy will interfere with cell division at a discrete stage, so that the cells, say, for layer 3 of the limbic cortex are not generated. Subsequent layers are generated and laid down normally, but with a particular population of cells missing in the middle layer, the connections from one part of the brain to another may falter. Thus, the individual may find it difficult to bring together different types of information or to respond appropriately to a stimulus. Disorders of this kind, which have less to do with overall anatomical structure than with the brain's ability to form and use synaptic connections, may play a role in some psychiatric illnesses for which there is no obvious physical cause.
The cerebral cortex is a fascinating object of study from many perspectives. It comprises by far the largest portion of the human brain—about three-quarters, in the adult—and is arguably the single anatomical structure that most sets us apart from other animals, even from other hominoids such as the chimpanzee (with whom we share well over 95 percent of our genetic makeup). Yet apparently no single transmitter or type of cell is unique to the human cerebral cortex; the molecules found in the cortex can also be found elsewhere, in our muscles, heart, and intestines and in the brains of other animals as well. The molecules, and even the cells, may be the same; it is the patterns of connectivity that make a difference. The connections, or synapses, among neurons in the human brain are not only more numerous but also more intricately patterned than anything that has ever been constructed to process information, including the most sophisticated supercomputer.
A structure so complex must be considered in smaller units if it is to be understood at all, and up to now neuroscience has managed to get along quite well with two mutually incompatible systems. One system for subdivision was devised by the German psychiatrist K. Brodmann at the turn of the century. Brodmann distinguished 57 areas of the cortical surface on the basis of their tissue composition, and the reference numbers he assigned are still widely used today. A researcher giving a talk before an international audience of neuroscientists can mention "area 44" and be understood without further explication. The other system subdivides the cortex into areas of specialized function—which do not correspond well to Brodmann's physically discrete areas, unfortunately. Thus, in terms of function, one can refer to Broca's area, which controls the ability to translate thoughts into speech (but not the ability to understand when someone else speaks; that function is housed in Wernicke's area, which is nearby, but not adjacent). The area is defined quite clearly in terms of its function, but its physical extent is harder to outline. In Brodmann's scheme, Broca's area occupies some of area 44 and some of area 45, as well as a little of area 4.
Current techniques of magnification and imaging permit the analysis of different tissues at the molecular level and have added another order of information to an already complicated picture. But this new information may ultimately be the bridge between the functional map of the cerebral cortex and the physical map, because it offers finer distinctions within functional areas and reveals the differential distribution of certain molecules along the lines of function. The molecules being considered are often receptor sites and the particular neurotransmitters that go with them; and (as we have seen in Chapter 5) it is through the neurotransmitters and their receptor sites that the brain translates its countless functions into chemical terms and back again into function. In this regard, the research team of Pasko Rakic at the Yale University School of Medicine has worked extensively with areas 17 and 18, which roughly correspond to the primary visual cortex—the part of the brain that must receive sensory impulses from the eyes before the visual association cortex (located nearby) can tell us what we see or how we feel about it.
In experimenting with differential development, Rakic and his colleagues have found that neighboring areas can be related in function and yet compete for some of the same resources—namely, territory and energy—so that if chance or environmental circumstances favor it, one area may develop at the expense of another. Clearly, such contests have implications for the shaping of the brain on an evolutionary time scale as well as over the course of an individual's development. Even in the short evolutionary interval from monkeys to humans this kind of reapportionment can be found: for example, the primary visual cortex, which makes up 15 percent of the cerebral cortex in the monkey, accounts for only 3 percent of our own cerebral cortex, while other areas have grown disproportionately larger. Among individuals of the same species, too, and even between the two hemispheres of an individual's brain, there can be variations in the size of a particular area, although nothing like the 3 to 15 percent difference just mentioned. Even when quite subtle, these variations can yield evidence of the intermingled effects on development of genetic information, random mutations, and environmental influences.
Mass Production of Brain Cells
The assembly of a human brain, a complex undertaking on a non-negotiable schedule, calls for a vast number of cells of suitable design, available at a convenient location. Cell proliferation therefore is a critical early stage of brain development, and one in which even small changes—in the timing of a cell-generating cycle, the duration of such a cycle, or the number of cycles altogether—can have major consequences for the final product.
Proliferation takes place largely under the control of regulatory genes, which act primarily to affect the operation of other, structure-building genes. The first structures laid down contain some of the specifications for the more advanced structures of the next stage, and so on. In this way, the genetic coding that sets a developmental process in motion need not contain all the information expressed in the final structure—only enough to move the process along to a point where a fresh element (such as a hormone or a neurotransmitter newly accessible to the developing structure) can provide further specifications.
Brain cells proliferate according to a scheme that combines order with enormous productivity. In the ventricular zone, a small number of precursor cells divide in two; then, in another cycle, each precursor cell divides again, perhaps several more times. The effect of each cycle at this stage is to double the number of cells; therefore, adding even a single cycle, for example by extending the duration of this early proliferative stage, could make a great difference in the overall size of the brain. As it happens, the difference in size between a monkey's cerebral cortex and that of a human can be accounted for by just a little more than three such cycles. And indeed, the entire neuron-generating stage, including these early cycles that immediately double the number of cells as well as later cycles in which multiplication proceeds more slowly, does seem to follow this rationale in its timing. The neuron-generating process in both monkeys and humans begins on about the 40th day after conception; the process ceases in monkeys on about the 100th day but continues in humans for about another 25 days.
By contrast, adding an extra few cycles at a later stage would have a much less striking effect, because by then most cells are dividing asymmetrically; that is, each cell produces one daughter (which does not divide) and one progenitor (which does). Furthermore, by the late stages, most of the cells have migrated to their eventual positions and are aggregating into the cerebral cortex. An extra couple of cell divisions at this point would produce not more surface area, which is the essential property of a larger cortex, but only an extra layer of cells on top of the surface that is already taking shape.
One of the reasons for this limitation, and a guiding principle in the construction of the brain, is that the proliferative ventricular zone apparently holds information about both the quantities of various cells needed and their eventual function or location. Pasko Rakic and his colleagues propose that somewhere in the mosaic of the ventricular zone is a protomap of the future regions of the brain, including the cerebral cortex. This map is actually better understood as a series of columns packed closely together on the surface of the cerebral ventricles. The precursor cells divide in two while at the ventricular surface and then move off to synthesize a full complement of DNA; afterward, they shuttle back to the surface to undergo another cell division. This process is repeated numerous times, until about the 100th day of gestation of a rhesus monkey, for example. But the end of cell proliferation does not mean that the columns have outlived their usefulness. On the contrary, they are essential for the accuracy of the next, and in some ways most remarkable, stage in the development of the cerebral cortex.
Migration To The Cerebral Cortex
The mammalian brain develops from the core outward. Long before the recognizably wrinkled surface of the cerebral cortex appears, the hollow, fluid-filled ventricles are present. These serve both as a connection back to the spinal cord (and a reminder of the still earlier neural tube) and as the site of origin for the new elements that will ultimately be assembled into the outermost surface of the brain, the cerebral cortex. Thus, in the course of development, the neurons and supporting glial cells of the cortex must somehow make their way there from the ventricular zone. This stage has been described as a massive migration of cells, and the distances involved are enormous, at least from the point of view of a single cell: some may travel as much as several millimeters to their eventual destination in the cortex.
But how does the cell "know" its eventual destination? Pasko Rakic suggests that the columns play an important role here. More specifically, the columns that make up the protomap at the ventricular surface could be seen as including a proliferative unit at the base and then a cellular pathway along which nerve cells travel when they have stopped dividing and begun to mature. As the neurons of each unit migrate along the pathway in a set order and settle into position in the cortex, they would reproduce faithfully the orderly arrangement of the units in which they originated—a feature termed cytoarchitectonic, for "architecture of the cells." According to this model, the surface area (even if convoluted) of each region of the cerebral cortex would be a function of the number of proliferative units contributing to it, whereas the thickness of the cortex at any particular spot would depend on the number of cell-division cycles that occurred within a unit. As an example, the primary visual cortex of the monkey comprises roughly 2.5 million such units, each containing about 100 to 120 cells. (These are arranged in the characteristic six layers of the cerebral cortex described in Chapter 2.)
A migrating neuron is guided along its set pathway by special adhesion molecules arrayed on a temporary framework of supporting (glial) cells. The glial cells composing the pathways for most neurons are extremely elongated and stringy in form, making a dense radial pattern from the ventricular zone to the outer layers of the developing brain. Once the stage of migration is accomplished, some of these glial cells degenerate; others undergo cell division and join the mature network of supporting cells, the "white matter," in the brain. Although occasionally a migrating neuron may transfer from one set of radial glial fibers to another en route, most of the time the adhesion molecules are strong enough to keep a neuron in the path to which it first became attached from the ventricular zone and to draw the neuron without entanglement through the dense arrangement of other cells, axons, and dendrites that are accumulating in the cortex.
A smaller number of cells are apparently uninfluenced by the radial glial pathways and instead follow a different set of paths by adhering to the surface of axons. These cells are likely to migrate along the outer margin of the brain, perpendicular to the radial glial pathways; they may migrate from one region to another or even across the midline that divides the two hemispheres. Clearly, a different set of adhesion molecules is responsible for this type of migration, which helps to form several important elements of the pons and the medulla in the brainstem, for example. Even more unusual is the sort of migration by which the cerebellum is formed: the granule cells that form a layer in the "little brain" at the back of the head show affinities for both axonal and glial surfaces and, in effect, combine the two forms of migration. The exceptional approach of the granule cell makes it a good case study for the mechanics and other properties at work in preferential cell-adhesion.
One other striking aspect of neuronal migration is the order in which the six layers of the cortex are built up: from the innermost to the outermost. Each migrating neuron, before arriving at its own predetermined site in the cortex, must travel outward through all the neurons that have migrated and settled in the cortex before it. As a result, each layer of the cortex, as it builds up, has the opportunity to carry an accretion of information from nearby cells that have preceded it—information that may help to lay the groundwork for the next developmental stage.
The Formation Of Synapses And Regions
After migration, the tendency of recently arrived neurons to cluster with similar cells into distinct regions determines the form and ultimately the function of each part of the brain. At the upper and outer surface, the cortical sheet becomes continuous at this stage and begins to compress into its characteristic folds and creases, as more cells from the proliferative units continue to add surface area to an already crowded space. The various types of cells also finish differentiating, so that each type has the biochemical properties, receptor sites, and other features appropriate to its region and layer. The cell body of the neuron grows longer and extends its axon (for transmitting signals to a target cell) and it also puts forth numerous branching dendrites (for receiving signals and conveying them back to the cell body).
The process of aggregation is highly ordered. Cells of the same type recognize one another and draw together; in many populations of neurons, cells may even arrange themselves with the same orientation. (For example, the large pyramidal neurons in the cerebral cortex that transmit impulses to other regions tend to align themselves with their axons extended toward the underlying white matter and their dendrites pointing toward the surface.) Additionally, in at least some contexts, axons tend to grow in bundles, or "fasciculations," closely associated with one another; they dissociate somewhat as they approach their target neurons, which suggests that there may be some form of recognition molecules, and possibly adhesion molecules as well, along the surface of axons.
Most important, the cells form synapses or connections with one another. As discussed in Chapter 2, a synapse may occur between the axon of one neuron and the dendrite of another, between the axon of one neuron and the cell body of another, or between axons or dendrites themselves. Synapses also form between cell bodies directly, for the exchange of signals by electrical impulse rather than through neurotransmitters (see Chapter 2). Whatever the nature of the synapses, it is their universality—the degree to which they connect everything with everything—that makes the human brain such a superb integrator of information. The burgeoning of synapses in all directions is at least partly directed by several messenger molecules, which are also to be found in the adult nervous system. In the mature brain they may act, for example, as second messengers broadcasting a signal within the cell, or as neuromodulators influencing the way a signal is received at a synapse. But at the aggregation stage of the developing brain, these compounds have other effects, such as enhancing the site recognition that may precede the forming of a synapse, or supplying nutrition to the nerve cell as it is forming a synapse.
The target cell toward which an axon is growing can also help with synapse formation by providing some of the chemical compounds needed by the axon. The best known of these compounds is nerve growth factor, which the axon takes up by means of specific receptors and transports back to its cell body. A cell nourished with nerve growth factor may have an advantage at the next stage of development, when large numbers of cells will be eliminated. Conversely, cells sensitive to nerve growth factor tend to retract their axons from target cells that do not supply it.
Outside the nervous system, other factors contribute to the development of the brain by their influence on the forming of synaptic connections. The fetus itself, in kicking, turning, and (by the fifth month) even sucking its thumb, stimulates the growth of synapses. In addition, at this stage, some conditions of the environment can act directly on the fetal senses: temperature, pressure, and even a rudimentary kind of hearing (although the auditory association cortex is not yet equipped to make sense of what the fetus ''hears").
Cellular Competition
Taken together, all these growth-enhancing factors (and perhaps others that are not yet identified) give rise to an overproduction of neurons, as well as of everything associated with them: axons, dendrites, the signal-receiving spines on dendrites, and every form of synapse. For example, the developing brain of the rhesus monkey, midway through gestation, has more than twice as many axons as the brain of an adult monkey. In our own species, it is estimated that the newborn arrives with trillions of synapses in her teeming head, a great many of which will cease to exist over the next 12 years or so. Yet far from indicating a loss of function or a decline in brainpower in childhood, the long-drawn-out process of selection is the final essential stage in the development of a nervous system unique to each individual. This uniqueness is a physical fact: the full universe of synaptic connections that takes form in any given human brain reflects the sum of the influences—genetic, nutritional, toxic, environmental, social, psychological, educational, and even accidental—that have all converged, unpredictably and irreproducibly, during the development of this particular brain. The elimination of great numbers of synapses, along with some neurons themselves, is a process widely observed among mammals (and among some other vertebrates as well); thus it appears that the large quantity of synapses present in the brain at birth does not represent the optimum number for a lifetime but rather serves the purpose of providing some room for selection.
This is not to say, however, that some populations of cells and synapses are somehow destined to remain and become permanent while others are programmed to exist only briefly. Instead, in an enactment of Darwinian natural selection at the cellular level, synapses and even neurons compete for survival.
Once we recognize that the early quantities of neurons and synapses are larger than optimal, the outlines of such a competition are easier to see. The population is overly large (in humans, the period of excess synapses continues until about 18 months of age); the territory cannot be expanded (the skull poses definite boundaries); and therefore, individuals must compete for limited resources.
The resources at issue are probably relatively few: nourishment from the target cell (such as nerve growth factor, discussed above), available space on the membrane surface of a target cell, and nerve impulses themselves, which convey information back to the targeting cell body and thereby stimulate its growth. The type of resource that would be crucial for a given synapse depends, of course, on which type of synapse it is. The contest goes on at all levels: a single neuron may first establish communication with its target cell by means of several axons, only one of which will ultimately survive; or a single dendrite may initially receive signals from a neighboring neuron on many dendritic spines, some of which will be eliminated with the onset of maturity. Axons may be retracted (as mentioned earlier), broken down, and absorbed back into the cell for reuse. Alternatively, axons or dendrites, or even whole cell bodies, may simply be allowed to die (most likely from a lack of nourishing factors, rather than of space or stimulation). Eventually they are cleared from the system like other cellular debris.
The cells thus eliminated may include not only those that have lost out in the competition for resources but also some that have misread directional cues at the time of migration and have made their way into inappropriate settings. Some ectopias, as these abnormally situated cells are called, remain in place and can contribute to several recognizable disorders of brain development; most are eliminated. The stage of selective cell death thus provides an opportunity to correct errors as well as a means of sculpting a nervous system into its unique shape.
Although such sculpting may achieve quite precise ends as it eliminates excess cells and synapses, it also goes well beyond the level of slight adjustments here and there. The extent of this process is difficult to comprehend in the abstract. To reduce it to more concrete terms, the Rakic research group has looked at the rate of destruction specifically in the corpus callosum, the tough bundle of nerve fibers that connects the two hemispheres of the brain. In the adult macaque monkey, this bundle contains about 50 million axons. But the macaque brain at birth contains about 200 million axons in this same area. To reach the level at which it functions in adulthood, the corpus callosum apparently eliminates axons at a very high rate—about 60 per second in infancy, for example. Synapses are lost at a much higher rate, since each branching axon could form several points of contact with a target cell. For the human brain, each of these numbers should probably be multiplied by about 10; but the principle of competitive elimination is the same.
The stage of elimination is extensive in another way, too, affecting not only synapses, axons, dendrites, or whole neurons but also receptor sites for specific neurotransmitters—again, an effective way of regulating the functions of the cell. Pasko Rakic and his co-workers plotted development in three regions of the cerebral cortex—motor, visual, and association—in the monkey and found that the number of receptors for dopamine first rose sharply and then fell, in parallel with the synapses and cells and also in parallel with the levels of dopamine itself in the region. Clearly, this stage of widespread destruction—which involves great waste and yet is essential for proper functioning—still presents some puzzles for investigators.
Exploring New Areas
The sequence of development in the human brain is much better understood than it was even 20 years ago. Suitably, as the several developmental stages themselves have been found to overlap considerably in their timing, researchers are pursuing investigations into several aspects of the process simultaneously. In the Rakic laboratory, along with work on migration, aggregation, and the end-stage shaping of the nervous system, researchers are also inquiring into a very early stage. How are cells of, for example, the cortical plate (which will ultimately develop into the cerebral cortex) directed to differentiate into one of the six distinct cell types of the cortex and to migrate to the particular layer inhabited by that cell type? There are many hypotheses as to how this specificity comes about. One possible explanation is that all the cells of the cortical plate are equal and undifferentiated until axons from other areas of the brain form synapses with them, thereby leading them to develop into the appropriate target cells. An explanation almost the opposite of this has the development of a cell being prescribed, down to the last detail, in its genetic makeup. Between these two extremes is the possibility that genetic instructions and other developmental events each have some part in specifying the form and fate of a cell.
Tests to establish the roles of such tangled factors in brain development pose special problems because of the many orders of information present in highly condensed form. An experimental lesion in one area might effectively isolate the region to be studied, but it could also affect nearby or connected areas, adding unknown factors to the situation under study. Rakic's team, however, found that removing part or all of the optic nerve during development yields a predictable and precise result: the part of the thalamus that relays nerve impulses from the retina to the primary visual cortex grows to be smaller than usual, as does the specific part of the cortex to which it projects (area 17, according to Brodmann's scheme). In the cortical plate, however, everything develops as usual, and the border between areas 17 and 18 also appears to be intact; the number of cells per proliferative unit is its usual 120 or so. Yet although the overall territory of areas 17 and 18 shows no change, the two areas are no longer equal in size, as they would be under normal circumstances; instead, area 17 is proportionately smaller.
There are several ways to account for this. In the absence of live signals to area 17, cells that were originally set to become part of that area may have shifted their development to become part of area 18 instead. In this case, not only would area 18 be larger than the damaged area 17, it would also be larger than the normal area 18. However, what the investigators actually found was a smaller-than-usual area 17, a normal-sized area 18—and a new area that was neither one nor the other. This "area x" is distinct from both its neighbors in many respects, including the number of cells in some of its six layers; nevertheless it appears to be something of a hybrid.
Significantly, the synaptic connections formed between area x and any other region of the brain are, by definition, novel. This statement, bland though it may sound, actually holds exciting possibilities—for it offers an explanation of how entirely new cortical regions could have formed during the course of evolution. New cortical areas can be created by a mutation that controls cell proliferation when radial units are being formed. Such areas have the chance for specialization, and whole new sets of synapses transmitting information to and from these areas could prove advantageous now and again; and, if inheritable, they could spread through a population. In this way, the cortical map offering the greatest number of areas that are of use to a particular species would move to become the norm for that species. Although such a model for the emergence of the human brain does not lend itself to replication in the laboratory, it can indeed be tested—for example, by comparing it with evidence from paleoanthropological fossils or by computer simulations. Within the span of an individual lifetime, too, the novel connections of an area x can have implications for the unique circuitry of one person's brain. For example, the cortex of someone who is congenitally blind might include a less-developed area 17 and, perhaps within it or nearby, a hybrid area with novel connections and the possibility of novel functioning.
In neuroscience, study of the formation and development of the human brain holds a special place; many lines of investigation converge here. New methods in molecular biology may now make it possible to uncover specialized genes, for example, that may control cell production in the ventricular zone or regulate the deployment of cell-adhesion or cell recognition molecules along migratory pathways. Another set of genes under investigation may initiate the synthesis of neurotransmitters, receptors, and second messengers, and fix the timing for their emergence. Scientists are working, too, on the genes responsible for programmed cell death.
The study of brain development poses its own constraints and appears at times to offer only the most tentative conclusions because of the large number of variables that may all be operating at once. Nevertheless, it also offers a unique vantage point from which to observe the interaction of these same variables—from nutrition all the way down to genetic coding—in their countless possible combinations. At the same time, through its multistage model, this field of inquiry seeks to explain well-known disorders of the brain or nervous system in terms of disturbances at particular points in development. Developmental neuroscience thus forms connections with all its neighboring areas and beyond, much like the system it is observing. No wonder that some of the researchers who specialize in this area think that learning about how the human brain takes shape is "the ultimate study of mankind."
Acknowledgment
Chapter 6 is based on presentations by Pasko Rakic.
- The Development and Shaping of the Brain - Discovering the BrainThe Development and Shaping of the Brain - Discovering the Brain
Your browsing activity is empty.
Activity recording is turned off.
See more...