NCBI Bookshelf. A service of the National Library of Medicine, National Institutes of Health.
Toxicological Profile for Nitrophenols. Atlanta (GA): Agency for Toxic Substances and Disease Registry (US); 2023 Apr.
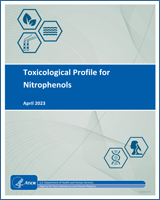
Toxicological Profile for Nitrophenols.
Show details3.1. TOXICOKINETICS
Information on the toxicokinetics for nitrophenols is available from several animal studies and mostly focuses on 4-nitrophenol.
- Absorption of 4-nitrophenol following both dermal and oral exposure is rapid. For oral exposure, limited data indicate that the extent of oral absorption is 36%. The extent of dermal absorption appears to be species-dependent, ranging from 11% in dogs to ~70% in rats and pigs. No inhalation data are available.
- Upon absorption, 4-nitrophenol is widely distributed in the body after oral exposure. The highest distribution is to the gastrointestinal tract. 4-Nitrophenol is also found in the kidneys, liver, plasma, placenta, and maternal brain within 30 minutes of exposure. The levels steadily decrease across all tissues over a period of 24 hours. Dermal application of 4-nitrophenol in animals results in very minimal body burden of 4-nitrophenol. No inhalation data are available.
- Both 2- and 4-nitrophenol undergo metabolic transformation by hepatic and extrahepatic phase I and phase II metabolism. Phase I reactions mediated by cytochrome P450 include oxidation to form nitroquinone and 4-nitrocatechol, and reduction to yield 2- and 4-aminophenol for 2- and 4-nitrophenol, respectively. The resulting metabolites and the parent compounds undergo phase II biotransformation reactions, which include conjugation with glucuronic acid to form glucuronides, inorganic sulfates to form sulfates, and for 4-nitrophenol, glutathione to form mercapturic acid derivatives.
- 4-Nitrophenol is excreted rapidly in the urine following oral or dermal exposure, with very small amounts excreted in the feces; elimination half-life values were approximately 4 hours. Following oral exposure, the administered dose was excreted as 4% glucuronides, 8% sulfates, 11% hot-acid hydrolysates, 16% non-conjugated compounds, and 61% water-soluble metabolites. Characterization of metabolites following dermal exposure is limited but indicates that >50% of the excreted dose is the conjugate form.
3.1.1. Absorption
No studies were identified regarding the rate and extent of absorption in humans following exposure to nitrophenols via any route. In animals, available data on the rate and extent of absorption are limited to 4-nitrophenol following oral or dermal exposure.
4-Nitrophenol is rapidly absorbed in animals after oral exposure. Abu-Qare et al. (2000) observed that 36% of a single gavage dose of 4-nitrophenol in Sprague-Dawley rats was absorbed in the gastrointestinal tract after 30 minutes. The study authors attributed this absorption of an oral dose of 4-nitrophenol in the gastrointestinal tract to the lipid solubility of the chemical’s non-ionized form (Abu-Qare et al. 2000). Abu-Qare et al. (2000) also showed that metabolites detected in urine can be used as a proxy measure for absorption of 4-nitrophenol after oral exposure in animals. Other oral studies that measured metabolites in the excreta indicated absorption of 4-nitrophenol (Robinson et al. 1951a; Williams 1938). Single oral doses between 182 and 264 mg/kg resulted in detection of sulfate-conjugates of 4-nitrophenols in the urine of rabbits (Williams 1938). Robinson et al. (1951b) reported the excretion of nitro compounds and conjugates in the urine of rabbits after gavage doses of 2-nitrophenol (200–330 mg/kg), 3-nitrophenol (150–200 mg/kg), and 4-nitrophenol (150–200 mg/kg). Lawford et al. (1954) showed that in a monkey, oral absorption of 4-nitrophenol was rapid, since peak blood concentrations of the compound were achieved within minutes after a gavage dose of 20 mg/kg.
Dermal application of 4-nitrophenol in animals showed species-dependent absorption. In rabbits and dogs, 35 and 11%, respectively, of the total dose of 14C-labeled 4-nitrophenol was recovered in the urine over a 7-day period following dermal exposure (U.S. Army 1983). The calculated absorption rate, determined by quantifying the radiolabeled 4-nitrophenol in the urine over 2 days, was 16% of the dose/day for 2 days in rabbits and 3% of the dose/day for 2 days in dogs. Thus, absorption was more extensive and more rapid in rabbits than in dogs. Unabsorbed 4-nitrophenol accounted for 53 and 86% of the applied dose in the rabbits and dogs, respectively (U.S. Army 1983). In rats, ~70% of an applied dose of 14C-4-nitrophenol was recovered in the urine and feces within 120 hours of dermal exposure; nearly 30% of unabsorbed 4-nitrophenol was washed off of the skin 24 hours after dermal application (Hughes and Hall 1997).
In another study, 4-nitrophenol was topically applied to the shaved abdomen of female weanling pigs at a concentration of 300 μg (150 μg cold 4-nitrophenol + 150 μg 14C4-nitrophenol) in 100 μL ethanol vehicle over a 7.5-cm2 area, yielding a final concentration of 40 μg/cm2 (Qiao et al. 2000). The skin was non-occlusively covered and 4-nitrophenol was left in place for 96 hours. Approximately 71% of the applied dose was absorbed. The reported topical bioavailability was 57%, the mean absorption time was 27.05 hours, and the absorption half-life was 18.75 hours (Qiao et al. 2000). Following a single dermal dose of 160 mg/kg 4-nitrophenol in male and female mice, the estimated absolute bioavailability was 21% in male mice and 19% in female mice, with a maximum plasma concentration occurring 1 hour after dosing for males and 2 hours after dosing for females (Eichenbaum et al. 2009).
3.1.2. Distribution
No studies were identified that quantitatively described distribution in humans following exposure to nitrophenols via any route. In animals, available data on the distribution are limited to 4-nitrophenol following oral or dermal exposure.
One study in rats evaluated measured tissue concentrations at various time points after a single oral dose of 100 mg/kg 14C-labeled 4-nitrophenol in pregnant Sprague-Dawley rats (Abu-Qare et al. 2000). This study found that >50% of the radioactivity was recovered in the gastrointestinal tract 30 minutes after exposure, and that the highest tissue concentration of radioactivity 30 minutes after exposure was the kidney, followed by the liver with about half the concentration of the kidney, followed by the plasma with about a third the concentration of the kidney (Abu-Qare et al. 2000). Abu-Qare et al. (2000) also observed distribution of 4-nitrophenol into the tissue of the fetus, suggesting that 4-nitrophenol crosses the placental barrier after oral exposure in rats.
Studies indicate that 4-nitrophenol is minimally distributed in animals after dermal exposure. Following a dermal application of 14C-4-nitrophenol, the body burden of 4-nitrophenol in rats measured 120 hours post-exposure was minimal (0.4%), with the majority of 4- nitrophenol being excreted via urine (63%) and feces (3%) during this time period (Hughes and Hall 1997). Similarly, only 2% of 4-nitrophenol was detected in the body of weanling piglets 96 hours after dermal exposure (Qiao et al. 2000).
Evidence suggests that there is also minimal body burden following intravenous or intraperitoneal exposure. Following intravenous exposure, <0.04% 4-nitrophenol remained in the body of female weanling piglets 96 hours after dosing (Qiao et al. 2000). Intravenous injection of 14C-labeled 4-nitrophenol to rabbits (0.12 mg/kg) or dogs (0.06 mg/kg) resulted in undetectable levels of radioactivity in all major tissues and organs 7 days after treatment (U.S. Army 1983). Similarly, the body burden of 4-nitrophenol in rats after intraperitoneal administration was approximately 0.4% after 120 hours of exposure (Hughes and Hall 1997). Eichenbaum et al. (2009) reported a drastic drop in plasma concentration of 4-nitrophenol just 15 minutes after daily intravenous administration in male and female mice for 2 days; the study authors suggested that this is evidence of extensive distribution of 4-nitrophenol outside the plasma.
3.1.3. Metabolism
4-Nitrophenol is readily metabolized in the body. The primary metabolic pathway for 4-nitrophenol is conjugation with the formation of glucuronide or sulfate conjugates. Metabolism also occurs through reduction or oxidation. Figures 3-1 and 3-2 depict the metabolism pathway for 2- and 4- nitrophenol after oral administration in rats, respectively, as adapted from Robinson et al. (1951a). A metabolic pathway figure for the metabolism of 3-nitrophenol has not been identified but is expected to be similar to 2- and 4-nitrophenol. As metabolic information on nitrophenols comes exclusively from animal studies, some differences may be anticipated regarding how this information generalizes to metabolism pathways in humans.

Figure 3-1
Proposed Metabolic Pathway of 2-Nitrophenol Following Oral Administration in Rats.

Figure 3-2
Proposed Metabolic Pathway of 4-Nitrophenol Following Oral Administration in Rats.
No studies regarding metabolism in humans following exposure to nitrophenols were identified. Studies regarding metabolism in animals include a few studies evaluating 2-, 3-, and 4-nitrophenols after oral exposure and 4-nitrophenol after dermal exposure.
4-Nitrophenol is rapidly metabolized in animals after oral exposure. Robinson et al. (1951a) studied the metabolism of orally administered 2-, 3-, and 4-nitrophenol (200–330 mg/kg) in rabbits. In these rabbits, conjugation was almost complete, with 70% of the dose excreted in urine being in the form of nitrophenol glucuronides (Robinson et al. 1951a). Eighty percent of the nitro group of the nitrophenols was excreted in urine and unchanged; the rest underwent reduction ranging from 6 to 14% of the dose. Oxidation accounted for <1% of the dose. Figure 3-1 shows that 2-nitrophenol undergoes phase I metabolism by oxidation to form nitroquinone and reduction to form 2-aminophenol. The two metabolites and the parent compound undergo phase II metabolism through conjugation with glucuronic acid to from glucuronides and inorganic sulfates to form sulfates (Robinson et al. 1951a). Figure 3-2 shows that 4-nitrophenol undergoes metabolic transformation by hepatic and extrahepatic phase I and phase II metabolism (Machida et al. 1982). Phase I reactions mediated by cytochrome P450 include oxidation to form 4-nitrocatechol and reduction to yield 4-aminophenol (Machida et al. 1982). The two polar metabolites and the parent compound undergo phase II biotransformation reaction, which includes conjugation with glucuronic acid to form glucuronides, sulfuric acid to form sulfates, and glutathione to form mercapturic acid derivatives (Machida et al. 1982). 4-Nitrophenol hydroxylation to 4-nitrocatechol is mediated by the CYP2E1 enzyme (Abu-Qare et al. 2000).
4-Nitrophenol is rapidly metabolized in dogs, rabbits, and pigs after dermal exposure. 4-Nitrophenol is metabolized by conjugation with glucuronic (60–80%) and sulfonic acid (10–20%) and reduction to aminophenols (10%); <1% of the dose is excreted unchanged as 4-nitrophenol (U.S. Army 1983). While the acid conjugates are excreted rapidly, reduction to aminophenols may take 48 hours (U.S. Army 1983). The observed absence of labeled 14C-4-nitrophenol in tissue specimens after topical exposure in dogs and rabbits supports an efficient metabolic clearance (U.S. Army 1983). Qiao et al. (2000) reported a metabolic half-life of 27.15 hours in pigs for the formation of 4-nitrophenol-glucuronide from 4-nitrophenol, with a metabolic rate constant of 0.037 L/hour (Qiao et al. 2000).
Parenteral exposure to 4-nitrophenol showed rapid metabolism in animals. Intravenous administration of 4-nitrophenol showed that the glucuronide and sulfate conjugates could be detected in the plasma within 1 minute after the injection of doses between 1.6 and 8.0 mg/kg (Machida et al. 1982). Machida et al. (1982) also demonstrated that rat liver homogenates had the greatest amount of glucuronidation activity, followed by the kidney, lung, and small intestine homogenates, in decreasing order. Sulfation, however, was detected almost exclusively in the liver. No differences in conjugation mechanisms for 4-nitrophenol between male and female rats have been reported (Meerman et al. 1987).
3.1.4. Excretion
No studies regarding excretion in humans following exposure to nitrophenol were identified. Studies in animals are limited to studies evaluating excretion following oral or dermal exposure to 4-nitrophenol. Although the information is limited, bioaccumulation of 2-, 3-, and 4-nitrophenol in organisms is not expected to occur due to the rapid excretion of the more polar metabolites.
4-Nitrophenol is rapidly excreted in animals after oral exposure. 4-Nitrophenol was rapidly excreted after a single oral dose of 100 mg/kg in rats, with more than half of the dose excreted through urine and feces after only 4 hours, and >95% of the dose having been excreted after 96 hours (Abu-Qare et al. 2000).
This dose of 4-nitrophenol was excreted as 4% glucuronides, 8% sulfates, 11% hot-acid hydrolysates, 16% non-conjugated compounds, and 61% water-soluble metabolites (Abu-Qare et al. 2000). Single oral doses between 182 and 264 mg/kg in another study resulted in detection of sulfate-conjugates of 4-nitrophenol in the urine (Williams 1938). Robinson et al. (1951b) reported the excretion of nitro compounds and conjugates in the urine of rabbits after gavage doses of 2-nitrophenol (200–330 mg/kg), 3-nitrophenol (150–200 mg/kg), and 4-nitrophenol (150–200 mg/kg).
Excretion of 14C-labeled 4-nitrophenol following dermal exposure was rapid in animals. In rabbits, 78% of the absorbed dose was recovered in urine obtained within 1 day of exposure and virtually complete elimination within 3 days (U.S. Army 1983). In dogs, 61% of the absorbed dose was recovered in urine obtained within 2 days of exposure, with virtually complete elimination within 5 days (U.S. Army 1983). Fecal elimination accounted for <1% of the applied dose in both species. In mice, the calculated elimination half-life values for 4-nitrophenol were 4.31 and 4.92 hours for male and female mice, respectively (Eichenbaum et al. 2009). In female weanling pigs, almost all of the administered 4-nitrophenol was recovered in the urine 96 hours following dermal administration (Qiao et al. 2000). More than half of the amount measured in urine was the conjugate form. As seen in rabbits and dogs, <1% of the dermal dose was recovered in the feces of pigs (Qiao et al. 2000).
Additional information regarding excretion can be found in intravenous injection studies. Within the first day after exposure in rabbits and dogs, 78 and 92%, respectively, of intravenously administered 4-nitrophenol was excreted (U.S. Army 1983). These results suggest a rapid excretion of 4-nitrophenol after intravenous exposure with little to no accumulation in the body. In mice, 4-nitrophenol was rapidly eliminated following a single intravenous dose of 25 mg/kg, with estimated half-life values of 1.09 and 0.687 hours in male and females, respectively (Eichenbaum et al. 2009). In female weanling pigs, approximately 90% of an intravenous dose was excreted in urine in the first 12 hours, with excretion half-lives for 4-nitrophenol and the conjugate of 0.84 and 0.86 hours, respectively (Qiao et al. 2000). The conjugate form comprised two-thirds of the total amount in urine and 4-nitrophenol comprised the other one-third (Qiao et al. 2000). As seen with dermal exposure, <1% of the administered dose was recovered in the feces (Qiao et al. 2000).
3.1.5. Physiologically Based Pharmacokinetic (PBPK)/Pharmacodynamic (PD) Models
PBPK models use mathematical descriptions of the uptake and disposition of chemical substances to quantitatively describe the relationships among critical biological processes (Krishnan et al. 1994). PBPK models are also called biologically based tissue dosimetry models. PBPK models are increasingly used in risk assessments, primarily to predict the concentration of potentially toxic moieties of a chemical that will be delivered to any given target tissue following various combinations of route, dose level, and test species (Clewell and Andersen 1985). Physiologically based pharmacodynamic (PBPD) models use mathematical descriptions of the dose-response function to quantitatively describe the relationship between target tissue dose and toxic endpoints.
No PBPK models have been published for nitrophenols.
3.1.6. Animal-to-Human Extrapolations
The metabolism of nitrophenols has not been studied in humans. The lack of this information precludes a non-speculative attempt to discuss potential interspecies differences or similarities in the toxicity of nitrophenols, as well as a determination of which animal species is the most appropriate model for humans. Extrapolations of nitrophenols oral toxicity data from animals to humans should consider the type of exposure because some of the differences in toxic and carcinogenic responses in animal studies can be explained based on saturation of the detoxification/excretion mechanism due to bolus (gavage) administration.
While it is unclear if methemoglobinemia is a health effect associated with 4-nitrophenol exposure based on no data in humans and low evidence in animals (see Appendix C for details), it is noted that humans could be more sensitive to this potential effect since it is estimated that rats have 2–5 times as much methemoglobin reductase activity, which controls the amount of methemoglobin in blood, compared to humans (Bloom and Brandt 1999).
3.2. CHILDREN AND OTHER POPULATIONS THAT ARE UNUSUALLY SUSCEPTIBLE
This section discusses potential health effects from exposures during the period from conception to maturity at 18 years of age in humans. Potential effects on offspring resulting from exposures of parental germ cells are considered, as well as any indirect effects on the fetus and neonate resulting from maternal exposure during gestation and lactation. Susceptibility to health effects from exposure to hazardous substances may differ between children and adults, and the relationship may change with developmental age.
This section also discusses unusually susceptible populations. A susceptible population may exhibit different or enhanced responses to certain chemicals than most persons exposed to the same level of these chemicals in the environment. Factors involved with increased susceptibility may include genetic makeup, age, health and nutritional status, and exposure to other toxic substances (e.g., cigarette smoke). These parameters can reduce detoxification or excretion or compromise organ function.
Populations at greater exposure risk to unusually high exposure levels to nitrophenols are discussed in Section 5.7, Populations with Potentially High Exposures.
Human populations that have experienced health effects from exposure to 2-, 3-, or 4-nitrophenol have not been identified, as little research has been conducted on this subject. Based on results from a study of ethanol-treated rats, it is possible that individuals who consume ethanol may have slower rates of clearance of 4-nitrophenol due to the presence of ethanol causing rapid metabolization of 4-nitrophenol into 4-nitrocatechol, which competes with 4-nitrophenol for the formation of sulfate and glucuronide conjugates (Reinke and Moyer 1985). This subpopulation, if exposed to 4-nitrophenol, may be considered potentially susceptible (Reinke and Moyer 1985).
While there is low evidence of hematological effects in animal studies following exposure to nitrophenols, a few studies report methemoglobinemia. The underlying cause for methemoglobinemia is the oxidation of ferrous (Fe2+) to ferric (Fe3+) iron within the hemoglobin molecule creating the dysfunctional methemoglobin molecule (Price 2011). Cells have an innate mechanism to protect themselves from oxidative stress with the help of systems like cytochrome b reductase, nicotinamide adenine dinucleotide (NADH) methemoglobin reductase, nicotinamide adenine dinucleotide phosphate (NADPH) methemoglobin reductase, reduced glutathione, and ascorbic acid (Price 2011). Depletion of the reducing power of these systems could potentially lead to methemoglobinemia. Xenobiotics and pharmaceuticals that have nitrites or nitrates in them can potentially act as powerful oxidizing agents, as they actively convert ferric to ferrous ions resulting in oxidative stress, which can give rise to methemoglobinemia (Price 2011).
Methemoglobinemia can be hereditary or acquired. Hereditary reasons include a rare dominant disorder where glutamate replaces valine in position 67 on the beta chain of the hemoglobin molecule (Price 2011). This permanently increases the methemoglobin levels to 15–30% (Price 2011). People affected are cyanotic but do not exhibit any other symptoms (Stucke et al. 2006). Cytochrome b5 reductase deficiency is another hereditary disorder that gives rise to methemoglobinemia. This is caused by an enzymatic lesion associated with the glycolysis pathway in red blood cells and is associated with cyanosis from birth (Percy and Lappin 2008). In addition to being a genetic disorder, this enzyme does not fully activate until 4 months after birth even in genetically normal infants, leaving them more susceptible to oxidative stress and subsequently to methemoglobinemia than adults (Price 2011). Newborn infants utilize fetal hemoglobin until they are 2–4 months old and have reduced oxygen-carrying capacity (Schechter 2008). Infants also have low levels of NADPH, which continuously reduces methemoglobin. Therefore, infants (as well as individuals congenitally deficient in this enzyme), and potentially pregnant women and their fetuses, may represent unusually susceptible subpopulations, as there is some evidence to suggest that 4-nitrophenol crosses the placental barrier (Abu-Qare et al. 2000; Naoum 2012). However, more research is needed to determine if children are especially susceptible to the health effects of exposure to nitrophenols.
External factors including medications and exposure to xenobiotics also cause methemoglobinemia. Angina and other cardiac-related incidents that are commonly treated using nitrite-based medications cause methemoglobinemia and are reported as a complication of the therapeutic use of these drugs (Bojar et al. 1987; Marshall and Ecklund 1980). Self-administration of local anesthetic drugs like benzocaine have also been known to cause this condition, and especially in children (Nappe et al. 2015).
Dapsone, a commonly used anti-inflammatory for treating infections has severe side effects including methemoglobinemia; it is recommended that patients use pulse oximeter to monitor blood oxygen levels regularly (Ashurst et al. 2010; Mahmood et al. 2019; Toker et al. 2015). Drugs to treat malaria (quinines) also cause methemoglobinemia (Kudale et al. 2014). For methemoglobinemia due to drug exposure, traditional first-line therapy is generally infusion of methylene blue.
Exposure to xenobiotics like aniline, chlorobenzene, fires, organic nitrites, and nitrites and nitrates from well water and food, respectively, are all implicated in causing acquired methemoglobinemia. As discussed in Section 2.7, exposure to 4-nitrophenol by inhalation for 6 hours/day, 5 days/week for 2 weeks caused an in increase in methemoglobin by 665% after 10 days at 2,470 mg/m3. After 14 days of recovery, erythrocytes, hemoglobin, and methemoglobin continued to be elevated by 7, 7.5, and 250%, respectively. One rat showed cyanosis after the first exposure. At a lower dose of 130 mg/m3, the rats showed a 200% increase in methemoglobin compared to control after 10 exposures and returned to normal after a 14-day recovery period. These results suggest that inhalation exposure to 4-nitrophenol could exacerbate any increase in methemoglobin that would occur in the subpopulations described above that have existing hereditary or acquired methemoglobinemia.
3.3. BIOMARKERS OF EXPOSURE AND EFFECT
Biomarkers are broadly defined as indicators signaling events in biologic systems or samples. They have been classified as biomarkers of exposure, biomarkers of effect, and biomarkers of susceptibility (NAS/NRC 1989).
A biomarker of exposure is a xenobiotic substance or its metabolite(s) or the product of an interaction between a xenobiotic agent and some target molecule(s) or cell(s) that is measured within a compartment of an organism (NAS/NRC 1989). The preferred biomarkers of exposure are generally the substance itself, substance-specific metabolites in readily obtainable body fluid(s), or excreta. Biomarkers of exposure to nitrophenols are discussed in Section 3.3.1. The National Report on Human Exposure to Environmental Chemicals provides an ongoing assessment of the exposure of a generalizable sample of the U.S. population to environmental chemicals using biomonitoring (see http://www.cdc.gov/exposurereport/). If available, biomonitoring data for nitrophenols from this report are discussed in Section 5.6, General Population Exposure.
Biomarkers of effect are defined as any measurable biochemical, physiologic, or other alteration within an organism that (depending on magnitude) can be recognized as an established or potential health impairment or disease (NAS/NRC 1989). This definition encompasses biochemical or cellular signals of tissue dysfunction (e.g., increased liver enzyme activity or pathologic changes in female genital epithelial cells), as well as physiologic signs of dysfunction such as increased blood pressure or decreased lung capacity. Note that these markers are not often substance specific. They also may not be directly adverse, but can indicate potential health impairment (e.g., DNA adducts). Biomarkers of effect caused by nitrophenols are discussed in Section 3.3.2.
A biomarker of susceptibility is an indicator of an inherent or acquired limitation of an organism’s ability to respond to the challenge of exposure to a specific xenobiotic substance. It can be an intrinsic genetic or other characteristic or a preexisting disease that results in an increase in absorbed dose, a decrease in the biologically effective dose, or a target tissue response. If biomarkers of susceptibility exist, they are discussed in Section 3.2, Children and Other Populations that are Unusually Susceptible.
3.3.1. Biomarkers of Exposure
No studies were identified regarding levels of 2-, 3-, or 4-nitrophenol in human tissues, fluids, or excreta that were associated with exposure to nitrophenols. While the presence of 4-nitrophenol in the urine may be due to exposure to 4-nitrophenol itself, it may also be the result of exposure to other chemicals such as methyl parathion and nitrobenzene, of which 4-nitrophenol is a metabolite (Barr et al. 2002; EPA 2009a; Li and Kannan 2018; Li et al. 2019), confounding its use as a specific reliable biomarker of exposure.
Due to the rapid excretion of 2- and 4-nitrophenol conjugates in the urine, their use as biomarkers of exposure may be limited to recent exposures only. Based on the current body of literature, it is not known if urinary excretion of 2- or 4-nitrophenol (or their conjugates) can be associated quantitatively with exposure to these chemicals. National Health and Nutrition Examination Survey (NHANES) data identifies the levels of 4-nitrophenol in urine; however, this could be due to exposure to 4-nitrophenol itself or to a chemical that is metabolized to 4-nitrophenol.
Hair has been used as a biomarker of exposure to 4-nitrophenol that captures cumulative exposure over a longer period, but these levels could also be due to exposure to 4-nitrophenol or those for which 4-nitrophenol is a metabolite, and more research is needed to understand the correlation of hair measurements with serum or urine concentrations of 4-nitrophenol (Béranger et al. 2018).
As discussed in Section 3.1.3, the pathways to metabolize 2- or 4-nitrophenol have only been identified in rats. 2-Nitrophenol is metabolized to 2-aminophenol, nitroquinone, sulfate conjugates, and glucuronyl conjugate. 4-Nitrophenol is metabolized to 4-aminophenol, 4-nitrocatechol, sulfate conjugates, and glucuronyl conjugates. All of the identified metabolites could be potentially used to detect exposure to 2-and 4-nitrophenol; however, none of them have been used as such in the literature. Additionally, while identification of metabolites of 2- or 4-nitrophenol in human tissues, fluids, or excreta could reflect exposure to nitrophenols specifically, it could also reflect exposure to alternate parent compounds that metabolize via the same pathways (e.g., nitrobenzene, methyl parathion). There is no literature on metabolism of 3-nitrophenol or biomarkers that could be potentially used to indicate exposure.
3.3.2. Biomarkers of Effect
No biomarkers of effect that are specific to nitrophenols have been identified. Ocular effects, including cataracts, are suspected health effects of nitrophenol exposure; however, these findings are not unique to nitrophenols. Evidence for other potential health effects, including methemoglobinemia and decreased body weight, is low and can also be observed following exposure to several different chemicals. More research is needed in this area to identify biomarkers of effect after exposure to nitrophenols.
3.4. INTERACTIONS WITH OTHER CHEMICALS
No studies were identified regarding interactions of 2-, 3-, and 4-nitrophenol with other chemicals in vitro or regarding interactions of 2-, 3-nitrophenol with other chemicals in vivo. There are in vivo studies that detail the interactions of 4-nitrophenol with other chemicals.
In ethanol-treated rats, 4-nitrophenol is rapidly metabolized to 4-nitrocatechol, which competes with 4-nitrophenol for the formation of sulfate and glucuronide conjugates (Reinke and Moyer 1985). This reduction in the conjugation of 4-nitrophenol may lead to the formation of amino derivatives.
The interaction between arginine, a feed additive, with antioxidant activities and parenteral (subcutaneous injections) exposure of 4-nitrophenol was investigated in Sprague-Dawley rats (Xu et al. 2016). The study showed that the changes in body weight and liver weight caused by 4-nitrophenol were significantly attenuated when treated with arginine orally (Xu et al. 2016). Additionally, the follicular deformation, irregularities in the granulosa arrangement and the increase in oxidative stress in female rat ovaries caused by parenteral exposure to 4-nitrophenol was ameliorated by arginine (Xu et al. 2016). Arginine is a known precursor to nitric oxide (Stuehr 2004) and nitric oxide is involved in the intracellular signaling to modulate folliculogenesis and atresia, steroidogenesis, prostaglandin biosynthesis, ovulation, luteolysis, and oocyte maturation (Hattori and Tabata 2006). A human study concluded that oral arginine supplementation resulted in some improvement in ovarian response, endometrial receptivity, and pregnancy rate in patients with follicular deficiencies (Battaglia et al. 1999). Based on this evidence, arginine may be able to mitigate potential effects of exposure to 4-nitrophenol in the ovarian tissues.
Another animal study, which subjected male ICR mice to parenteral (intraperitoneal injections) exposure to 4-nitrophenol, provides evidence that quercetin, a flavonoid, mitigates the effects of 4-nitrophenol on male reproduction (Mi et al. 2013). Quercetin is a polyphenolic compound that is present in foods of plant origin. The antioxidant properties of these flavonoids are crucial in the inhibitory role that they play in reducing reactive oxygen species (Hollman et al. 1996). The authors hypothesized that 4-nitrophenol produces toxicity in the reproductive system by causing lipid peroxidation and production of free radicals (Mi et al. 2013). This in turn causes increased oxidative stress and mitochondrial dysfunction resulting in cell apoptosis (Mi et al. 2013). The study authors also implicated the role of endoplasmic reticulum in the response to reproductive toxicity (Mi et al. 2013). The disruption of protein folding in endoplasmic reticulum altered the homeostasis, thus changing the downstream signaling cascades (Wu and Kaufman 2006). 4-Nitrophenol causes oxidative stress in the target organ system; quercetin, with its antioxidant properties, helps assuage this condition. In the study by Mi et al. (2013), quercetin supplementation is shown to repair the damage to seminiferous tubule epithelium and restore the damaged antioxidant status to normal levels by acting on multiple endpoints including Bcl-x1, XBP-1, and HO-1. Quercetin is an antioxidant that has shown the potential to attenuate the possible reproductive effects of 4-nitrophenol (Mi et al. 2013).
Chen et al. (2016) used phytosterol, a combination of plant sterols and stanols that are known to have antioxidant properties, to study its protective effects against 4-nitrophenol-induced effects in the liver using male Sprague-Dawley rats. In this study, oral phytosterol mitigated the hepatotoxicity caused by parenteral (subcutaneous injection) 4-nitrophenol suggesting that phytosterol has protective effects. This study continued to provide evidence to the mechanism through which 4-nitrophenol impaired normal physiology (i.e., it generated reactive oxygen species, increased oxidative stress, which led to peroxidation of lipids, and eventually led to membrane damage) (Chen et al. 2016).
- TOXICOKINETICS, SUSCEPTIBLE POPULATIONS, BIOMARKERS, CHEMICAL INTERACTIONS - Tox...TOXICOKINETICS, SUSCEPTIBLE POPULATIONS, BIOMARKERS, CHEMICAL INTERACTIONS - Toxicological Profile for Nitrophenols
Your browsing activity is empty.
Activity recording is turned off.
See more...