NCBI Bookshelf. A service of the National Library of Medicine, National Institutes of Health.
Toxicological Profile for Chlordane. Atlanta (GA): Agency for Toxic Substances and Disease Registry (US); 2018 Feb.
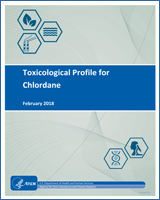
Toxicological Profile for Chlordane.
Show details3.1. TOXICOKINETICS
- Chlordane (a highly lipophilic substance) appears to be readily absorbed, regardless of the route of exposure.
- Initially, tissue levels are highest in the liver and kidneys; subsequently, chlordane and its metabolites are relocated to fat, where they persist for long periods of time.
- Metabolism results in a number of oxidation products, including oxychlordane, which persist in body fat as the predominant chlordane residues. Free radicals formed by reductive dehalogenation may play an important role in the toxicity of chlordane.
- Chlordane induces its own metabolism to toxic intermediates.
- Except for the rabbit, chlordane and its metabolites are excreted more readily in the bile than in the urine, due to the general lack of polarity and high lipophilicity of the terminal metabolites. Substantial amounts are also excreted via lactation.
3.1.1. Absorption
Data obtained from humans exposed to chlordane in termite-treated homes in Japan (Taguchi and Yakushiji 1988) or as the result of pesticide spraying (Kawano and Tatsukawa 1982; Saito et al. 1986; Takamiya 1987) indicate that blood or tissue levels of chlordane and/or its metabolites increase with exposure duration. These data indicate that chlordane can be readily absorbed across the respiratory tract.
Observations of chlordane residues in adipose tissue and systemic effects following inhalation exposure to chlordane technical confirm that absorption from the respiratory tract occurs (Asakawa et al. 1996; EPA 1987f; Khasawinah et al. 1989). An intratracheal study suggests that absorption of chlordane by the respiratory system of rats is rapid. A peak blood concentration of radioactivity equivalent to ≈4% of an intratracheal dose of radiolabeled chlordane was reached in <5 minutes (Nye and Dorough 1976). In reviewing the data from this study, Nomeir and Hajjar (1987) noted that ≈24% of the dose of radioactivity was present in the lungs 1 hour after treatment and concluded that ≈76% of the dose had been absorbed from the respiratory tract.
Information on the absorption of chlordane following oral exposure in humans comes largely from case reports involving accidental ingestion. A chlordane level of 2.71 mg/L was measured in the blood of a 20-month-old boy at 2.75 hours after he had ingested an unknown amount of technical-grade chlordane (Curley and Garrettson 1969). A chlordane concentration of 3.4 mg/L was measured in a serum sample taken from a 4-year-old girl at an unspecified time following the ingestion of an unknown amount of a 45% chlordane formulation (Aldrich and Holmes 1969). Chlordane was found in the blood plasma and in a variety of tissues from a 59-year-old male approximately 2 hours after he had ingested an unknown amount of chlordane (Kutz et al. 1983). A whole-blood chlordane concentration of 5 mg/L was measured in a 62-year-old male at 3.5 hours after he had ingested 300 mL of a 75% chlordane solution (Olanoff et al. 1983).
Chlordane appears to be rapidly absorbed from the gastrointestinal tract of rats; peak blood levels of radioactivity occurred 2–4 hours following administration of oral doses ranging from 0.1 to 1 mg/kg (Ewing et al. 1985; Ohno et al. 1986). Peak blood levels of radioactivity following a 1 mg/kg dose of 14C-chlordane were between 81 ng chlordane equivalents/mL for the cis isomer (Ewing et al. 1985) and 175 ng chlordane equivalents/mL for the trans isomer (Ohno et al. 1986). Absorption in the mouse was slower than in the rat; a peak blood level of 113 ng equivalents/mL of blood was observed in the mouse 8 hours after oral administration of a 1 mg/kg dose of 14C-cis-chlordane (Ewing et al. 1985). Absorption was not quantified following oral dosing in the mouse, but intraperitoneal studies indicated that a significant degree of biliary excretion also occurs in this species (Ewing et al. 1985). Administration of a mixture (1:1) of cis and trans isomers of chlordane (20 mg/kg for each of the isomers) to male mice resulted in peak absorption within 24 hours (Satoh and Kikawa 1992). In rabbits, the estimated absorption of chlordane following various repeated oral dosing regimens with radiolabeled cis and/or trans isomers has been estimated at 30–50%, based on radioactivity eliminated in the urine (Balba and Saha 1978; Barnett and Dorough 1974; Poonawalla and Korte 1971).
No quantitative data were located regarding absorption in humans after dermal exposure to chlordane. Derbes et al. (1955) reported a case of accidental death preceded by neurological signs typical of chlordane toxicity in a woman who was dermally exposed to a mixture of chlordane and other chemicals. This finding indicates that chlordane can be absorbed through human skin. Other studies indicate that absorption of chlordane through the skin is related to the application medium. Ambrose et al. (1953a) indicated that a topical dose of chlordane in rats (50 mg/kg) is more readily absorbed when the compound is administered in an oil vehicle instead of ethyl alcohol. In an in vitro study using diffusing cells in which 14C-chlordane was applied to human skin from cadavers for 24 hours, the amount of the applied dose of radioactivity recovered from the receptor fluid (human plasma) was 0.04% when the application medium was soil and 0.07% when the application medium was acetone (Wester et al. 1992). Much larger proportions (0.34% from soil and 10.9% from acetone) were retained within the layers of the skin. In an in vivo study, 14C-chlordane in soil or acetone was applied to the skin of monkeys for 24 hours (Wester et al. 1992). Absorption accounted for 4.2% of the dose in soil and 6% of the dose in acetone, based on recovery of 14C in the urine.
3.1.2. Distribution
Several studies report chlordane residues in the blood or fat of pest control operators, residents in homes treated for termites, or residents with no known mode of exposure other than background. Background exposures include inhalation of the material in ambient air and ingestion through food (Dearth and Hites 1991a; Sasaki et al. 1991a; Wariishi and Nishiyama 1989); dermal exposure also may be possible, although data regarding dermal exposure were not located. In pesticide spray applicators properly attired in protective clothing, the inhalation route is probably most important (Takamiya 1987). Nevertheless, most humans with any body burden of chlordane residues were probably exposed by multiple routes. Generally, levels of total chlordane residues in blood and milk fat increase as duration of exposure increases (Ogata and Izushi 1991; Saito et al. 1986; Taguchi and Yakushiji 1988; Takamiya 1987). Human milk fat contained total mean chlordane residues of ≤188 ppm, and blood levels were ≤0.015 ppm in exposed individuals (Ogato and Izushi 1991; Taguchi and Yakushiji 1988). Levels in fat and liver exceeded levels in the blood (Mussalo-Rauhamaa 1991).
Information on the distribution of chlordane and/or its metabolites in humans after oral exposure is from case reports involving ingestion of the compound. Approximately 2 hours after a 59-year-old male ingested a fatal dose of chlordane, autopsy revealed the following concentrations of chlordane: adipose tissue 22 μg, spleen 19.15 μg/g, brain 23.27 μg/g, kidney 14.10 μg, and liver 59.93 μg/g (Kutz et al. 1983). The level of chlordane in the adipose tissue of a 20-month-old boy who drank an unknown amount of technical-grade chlordane was 3.12 μg/g approximately 30 minutes after ingestion (Curley and Garrettson 1969). The concentration in adipose tissue peaked at 30–35 µg/g fat approximately 8 days following the incident. Fifty-eight days after a 62-year-old male ingested 215 g of chlordane, the reported levels of chlordane components and metabolites (oxychlordane, trans-nonachlor, and heptachlor epoxide) in the adipose tissue were 5 μg/g fat (Olanoff et al. 1983).
Other human data include reports of chlordane residues in blood, adipose tissue, and cord blood (Brock et al. 1998; Glynn et al. 2000; Kang et al. 2008; Rhainds et al. 1999; Tanabe et al. 1993), and adipose tissue and brain and liver autopsy samples (Dewailly et al. 1999).
The pattern of tissue distribution of chlordane and/or its metabolites in animals after oral exposure does not appear to depend on the size of the dose or whether exposure is to single or multiple doses. The tissue distribution patterns of radioactivity in rats, 1 day after exposure to a single oral dose of a 3:l mixture of radiolabeled cis- and trans-chlordane, were similar over a dose range of 0.05–1.0 mg/kg (Barnett and Dorough 1974). Levels of tissue radioactivity increased with increasing dose; at all dose levels, the highest concentrations of radioactivity were found in the fat followed, in order, by the liver and kidney. Lower concentrations were found in the brain and muscle. In this same study, oral administration of chlordane over a longer period of time did not change the distribution pattern of radioactivity from that observed following a single oral exposure. Rats fed the same mixture of chlordane for 56 days at dietary concentrations of 1, 5, or 25 ppm were observed to have high levels of radioactivity in the fat and much lower levels of radioactivity (in decreasing order) in the liver, kidney, brain, and muscle. The concentrations of radioactivity measured in these tissues were dose-dependent.
The accumulation of chlordane and its metabolites in fat appears to depend on exposure duration. Takeda et al. (1984) treated rats by gavage with technical chlordane at 10 μg/kg/day for 7 or 14 days. Chlordane and metabolites measured in the fat reached 30.4 μg/g wet tissue at the end of 7 days of treatment and 77.4 μg/g at the end of 14 days of treatment. Distribution to the liver and kidneys of rats after a single oral dose of chlordane is more rapid than distribution to adipose tissue. Levels of radioactivity peaked in the liver and kidneys of rats 2–4 hours after the administration of a single oral dose of radiolabeled γ-chlordane (0.05 or 10 mg/kg) (Ohno et al. 1986). In this same study, the level of radioactivity in the adipose tissue peaked at 16 hours (dose of 10 mg/kg) and 4 days (dose of 0.05 mg/kg) after administration of the compound. The concentrations of γ-chlordane equivalents in adipose tissue 10 days after the administration of either dose (0.05 or 10.0 mg/kg) were approximately 10 times higher than levels in the liver and kidney.
Dearth and Hites (1991b) measured the half-lives of depuration of 14 different chlordane components (e.g., cis- and trans-chlordane and cis- and trans-nonachlor) and metabolites (e.g., heptachlor epoxide, oxychlordane) from the fat of rats fed chlordane in the diet for 28 days. Half-lives ranged from 5.92 days (cis-chlordane) to 54.1 days (nonachlor III) and were apparently related to the metabolism rate of the various compounds. Structural characteristics associated with slowed depuration included an increasing number of chlorines on ring 1, the chlorine on Cl existing in an endo- (compared with an exo-) configuration, and the presence of two chlorines on C2. In mice treated once or every other day for 29 days, the whole-body content of cis- and trans-chlordane remained at very low levels; the content of cis- and trans-nonachlor and oxychlordane continued to increase with continued treatment (Hirasawa and Takizawa 1989). The investigators concluded that the chlordane isomers were readily metabolized, but that the nonachlor isomers were not. Oxychlordane, a metabolic intermediate of both chlordane isomers, is very slowly metabolized and tends to persist.
Elimination of radioactivity from the liver and kidney of rats treated with radiolabeled chlordane differs from elimination of radioactivity from peritesticular adipose tissue. Elimination of radioactivity from the kidneys and livers of rats treated with either high (10 mg/kg) or low (0.05 mg/kg) doses of γ-chlordane was biphasic; the initial rapid phase had half-lives in both organs ranging from 5.9 to 9.6 hours (Ohno et al. 1986). Half-lives for the slower, terminal phase of elimination in these organs ranged from 4.4 to 5.0 days. In contrast, elimination of radioactivity from peritesticular adipose tissue was monophasic and relatively slow (elimination half-lives of 9.1 days in the low-dose group and 8.4 days in the high-dose group). Skin retained radioactivity longer than any other tissue (elimination half-lives of 15.2 and 10.4 days for the low- and high-dose groups, respectively). Ewing et al. (1985) confirmed that peak concentrations of radioactivity were found in the livers of rats and mice 2–4 hours after administration of a single oral dose of radiolabeled cis-chlordane (1.0 mg/kg). The investigators observed that radioactivity was eliminated much more slowly from the livers of mice than from the livers of rats. They speculated that this may explain the susceptibility of mice to the development of hepatocellular carcinomas, whereas rats appear to be relatively insensitive to the formation of this tumor following chlordane administration (NCI 1977).
Several oral studies (Ambrose et al. 1953b; Barnett and Dorough 1974; Bondy et al. 2000; Street and Blau 1972) reported that female rats had higher levels of radioactivity in the fat than did the males. Bondy et al. (2000) observed dose-related increasing concentrations of oxychlordane, cis-nonachlor, trans-nonachlor, heptachlor, and trans-chlordane in adipose tissue of male and female rats administered technical chlordane by gavage at doses ranging from 0.25 to 25 mg/kg/day for 28 days. Oxychlordane and trans-nonachlor represented the majority of chlordane residues in adipose tissues and levels of these residues were >2-fold higher in dosed female rats compared with similarly dosed male rats. Levels of radioactivity in perirenal adipose tissue from female rats were as much as twice the levels observed in males (Ambrose et al. 1953b), and females tended to store a much larger proportion of this radioactivity in abdominal fat in the form of oxychlordane (Street and Blau 1972). Another recurrent observation in these distribution studies is an isomer effect on the amount of radioactivity that is distributed to the various tissues. Studies by Barnett and Dorough (1974) and Street and Blau (1972) indicated that significantly higher concentrations of radioactivity are stored in the tissues of rats following oral administration of the trans isomer, compared to the concentrations observed following administration of the cis isomer. This observation also holds true for rabbits. Balba and Saha (1978) administered four doses of either cis-chlordane (67 mg/kg body weight/dose) or trans-chlordane (30 mg/kg body weight/dose) to rabbits for 4 days. Although the administered dose of the cis isomer was more than twice the dose of the trans isomer, tissue levels of radioactivity were higher in animals given the trans isomer. When a 1:l mixture of cis- and trans-chlordane (total dose: 40 mg/kg) was administered to mice, higher concentrations of the cis- isomer were found in muscle liver, kidney, brain, and spleen (Satoh and Kikawa 1992). Concentrations of cis- and trans-chlordane were similar in blood. Oxychlordane concentrations peaked in the liver (1,918 ppb) at day 2, and peaked at day 1 in the following tissues: muscle (569 ppb), kidney (326 ppb), brain (226 ppb), spleen (126 ppb), and blood (103 ppb). The level of oxychlordane in adipose tissue was 2,890 ppb on week 8, but by week 52, it had decreased to 648 ppb. In addition, oxychlordane levels were higher in adipose tissue than any other tissue at 52 weeks.
3.1.3. Metabolism
Information on the metabolism of chlordane in humans is limited. Tashiro and Matsumura (1978) identified the metabolites of cis- and trans-chlordane following incubation of these compounds with human liver microsomal preparations. The following metabolites (in order of decreasing concentration) were identified: chlordene chlorohydrin, monohydroxylated dihydrochlordene, oxychlordane, and relatively smaller but similar amounts of 1,2-dichlorochlordene, 1-hydroxy-2-chlorochlordene, 1-hydroxy-2-chloro-2,3-epoxychlordene, 1,2-hydroxychlordene, trihydroxydihydrochlordene, and β-glucuronide-1-hydroxydihydrochlordene. Patterns of metabolites were similar whether the starting material was the cis or trans isomer. Kutz et al. (1976, 1979) reported the presence of oxychlordane in most adipose tissue samples taken at surgery or necropsy from humans. Data were not located regarding the levels of chlordane metabolites in the urine of exposed humans.
Tashiro and Matsumura (1978) reported that experiments with liver microsomal preparations from rats yielded results nearly identical to those for human preparations. These investigators noted, however, that rat microsomal preparations efficiently metabolized trans-nonachlor (a predominant component of technical chlordane) to trans-chlordane, but that human microsomal preparations did not. These data suggest that the metabolism of pure isomers of chlordane by humans and rats is similar, but that metabolism of components other than the pure isomers present in the technical product may differ.
Data regarding the nature of tissue residues in rats and monkeys following continual inhalation exposure for 90 days indicate that monkeys are less efficient metabolizers of chlordane than are rats (Khasawinah 1989). Oxychlordane is the predominant metabolite of trans-chlordane in rats and monkeys (Khasawinah 1989; Sasaki et al. 1992).
Chlordane has been known to undergo biotransformation in animals since the mid-1960s, when it was demonstrated by Poonawalla and Korte (1964) that 10–80% of the radioactivity found in the tissues and excreta of rats given an intravenous dose of radiolabeled cis-chlordane was in the form of water soluble metabolites. Subsequently, several metabolic schemes have been proposed based on information obtained from in vivo and in vitro studies in rats (Barnett and Dorough 1974; Brimfield et al. 1978; Tashiro and Matsumura 1978) and rabbits (Balba and Saha 1978; Poonawalla and Korte 1971). These proposed schemes lack consistency, and controversial issues include: differences in the identity of metabolites observed in in vivo versus in vitro experiments, possible isomer differences (i.e., cis versus trans) in the routes of metabolism followed, and whether metabolites thought to be terminal by some investigators (e.g., oxychlordane) are capable of undergoing further biotransformation by mammals. The metabolic scheme for chlordane in animals presented in Figure 3-1 was proposed as a synthesis of the available information by Nomeir and Hajjar (1987). This scheme involves four routes of metabolism for the chlordane molecule. No distinction is made between cis- and trans-chlordane in the qualitative nature of the metabolites formed. The first proposed metabolic route starts with hydroxylation at position three of the molecule to form 3-hydroxychlordane. This reaction is thought to be mediated by the microsomal mixed-function oxidase (MFO) system. Dehydration of 3-hydroxychlordane leads to 1,2-dichlorochlordene and eventually to other metabolites such as oxychlordane and 1-hydroxy-2-chlorochlordene. Alternatively, 3-hydroxychlordane may undergo replacement of chlorines by hydroxyl groups to form monochlorodihydroxylated and -trihydroxylated derivatives. The second pathway starts with dehydrochlorination to form heptachlor. The mechanism of this reaction is not completely understood but is thought to be mediated by the cytochrome P-450 system and/or by glutathione-S-transferase type enzymes. Further metabolism of heptachlor leads to 1-hydroxychlordene, heptachlor epoxide, or eventually to 1-chloro-2,3-dihydroxydihydrochlordene. The third pathway starts with dehalogenation of chlordane to form 1-chlorodihydrochlordene, probably mediated by microsomal MFO systems. Further reactions probably involve hydrolysis and conjugation with glucuronic acid. The fourth metabolic pathway, and probably the least understood, involves hydrolytic removal of a chlorine atom and its replacement by a hydroxyl group to form 1-chloro-2-hydroxychlordene chlorohydrin. This product may undergo further metabolism to form monochlorodihydroxy- and trihydroxy- derivatives of dihydrochlordene. Studies with rat hepatic microsomes suggest that cytochrome P-450 may be the most important enzyme to catalyze degradation of trans-chlordane (Kawano et al. 1989). Epoxide hydrolase is probably the predominant enzyme to catalyze degradation of oxychlordane. Reductive dehalogenation, with the production of free radicals, may also be important in the toxicity of chlordane (Brimfield and Street 1981; Kawano et al. 1989).

Figure 3-1
Proposed Metabolic Pathways for Chlordane.
The metabolic rate of various chlordane components appears to depend on three structural features (Dearth and Hites 1991b). First, compounds with two chlorines on ring 1 are metabolized 3 times as rapidly as those with three chlorines. Second, compounds with the chlorine on C2 in an exoconfiguration are metabolized 20–25% more quickly than compounds with an endo-configuration. Third, compounds with one chlorine on C2 are metabolized 3 times as rapidly as those with two chlorines. Dechlorination of compounds with three chlorines on ring one appears to be the first and rate-limiting step of metabolism of these compounds.
In mice treated orally every other day for 28 days with technical chlordane, cis- and trans-chlordane reached peak levels in the whole body on the first day and declined to lower levels in spite of repeated dosing; cis- and trans-nonachlor and oxychlordane increased during the entire study period (Hirasawa and Takizawa 1989). The ratio of cis-to trans-chlordane and cis-to trans-nonachlor in the test sample (6:7 and 1:4, respectively) and in the mouse body at termination of the experiment (5:3 and 1:7, respectively) suggests that trans-chlordane is metabolized more readily than cis-chlordane and that cis-nonachlor is metabolized more readily than trans-nonachlor. The decreasing content of the chlordane isomers and the increasing content of oxychlordane with repeated dosing suggests that chlordane induces its own metabolism.
3.1.4. Excretion
Information regarding excretion following inhalation exposure to chlordane is limited to the observation that recovery of radioactivity for 6 days following endotracheal administration of radiolabeled chlordane to rats was 52% in the feces and 12% in the urine (Nye and Dorough 1976).
Information regarding the excretion of chlordane and/or its metabolites from the human body after oral exposure is from case reports of accidental ingestion. These reports conclude that elimination from the plasma was biphasic in nature (Aldrich and Holmes 1969; Curley and Garrettson 1969; Olanoff et al. 1983). Marked differences existed, however, in the reported half-life of the terminal (slow) phase. Values reported for the terminal phase were 88 days (Aldrich and Holmes 1969), 21 days (Curley and Garrettson 1969), and 34 days (Olanoff et al. 1983). Small amounts of chlordane have been excreted in the urine of humans after oral ingestion of the compound. Aldrich and Holmes (1969) reported that the urinary concentration of chlordane decreased from 1.93 to 0.05 mg/L over the first 3 days following the ingestion of an unknown amount of chlordane by a 4-year-old girl. Curley and Garrettson (1969) reported a chlordane concentration in the urine of 0.309 mg/L at 24 hours after the ingestion of an unknown amount of chlordane by a 20-month-old boy. Fecal chlordane concentrations of 719 and 105 ppm have been reported on days 2 and 3, respectively, following chlordane ingestion by a 4-year-old girl (Aldrich and Holmes 1969).
The excretion of chlordane and/or its metabolites has been studied in animals following oral administration. In rats, 70–90% of the radioactivity administered (depending on the isomer) was eliminated within 7 days following a single oral dose of the radiolabeled pesticide (Barnett and Dorough 1974; Ewing et al. 1985; Tashiro and Matsumura 1977). In these studies, the cis isomer was eliminated more quickly than the trans isomer. From 70 to 90% of the radioactivity orally administered to rats was excreted in the feces; excretion of radioactivity in the urine ranged from 2 to 8% of the administered dose (Barnett and Dorough 1974). In another study, cis- and trans-chlordane were cleared from the blood within 7 days after male mice received a total dose of 40 mg/kg chlordane mixture (1:1) of both isomers (Satoh and Kikawa 1992). Although the cis isomer tended to accumulate more than the trans isomer, no difference in the half-lives in tissues (0.6–2 days) was observed between the two isomers. Both isomers were rapidly metabolized to oxychlordane. Oxychlordane was eliminated very slowly compared to the isomers and its half-life in blood was determined to be 25 days.
Studies by Ohno et al. (1986) and Ewing et al. (1985) indicate that biliary excretion of chlordane and/or its metabolites is significant in both rats and mice and is the source of fecal excretion by these species. Ewing et al. (1985) administered a 1 mg/kg dose of radiolabeled cis-chlordane to rats and mice by intraperitoneal injection and recovered 47% (rats) and 67% (mice) of the dose in the feces within 7 days. By using bile duct-cannulated rats, Ohno et al. (1986) showed that biliary excretion occurred more rapidly after oral administration than after intravenous administration, probably as a result of the first pass of blood from the digestive tract through the liver via hepatic portal circulation. The relative proportions of fecal and urinary excretion of radioactivity after oral administration of radiolabeled chlordane in rats do not appear to change significantly with dose over ranges of 0.05–10.0 mg/kg (Barnett and Dorough 1974; Ohno et al. 1986). In addition, longer-term administration of chlordane in the diet (1, 5, or 25 ppm for 56 days) did not change the excretion pattern significantly in rats from that observed following single oral dosing (Barnett and Dorough 1974).
In contrast to the excretion pattern of radioactivity observed in rats following oral exposure to radiolabeled chlordane, rabbits tend to excrete larger percentages of the administered dose in the urine. The percentage of the administered radioactivity excreted in the urine of rabbits following multiple oral doses ranged from 28 to 47% (Balba and Saha 1978; Poonawalla and Korte 1971). In these same studies, fecal excretion in the rabbit ranged from 22 to 48% of the administered dose. The greater urinary excretion of radioactivity in rabbits compared with rats may be due to the greater ability of rabbits to form water-soluble conjugates of chlordane metabolites. Biliary excretion was not studied in these experiments.
3.1.5. Physiologically Based Pharmacokinetic (PBPK)/Pharmacodynamic (PD) Models
PBPK models use mathematical descriptions of the uptake and disposition of chemical substances to quantitatively describe the relationships among critical biological processes (Krishnan et al. 1994). PBPK models are also called biologically based tissue dosimetry models. PBPK models are increasingly used in risk assessments, primarily to predict the concentration of potentially toxic moieties of a chemical that will be delivered to any given target tissue following various combinations of route, dose level, and test species (Clewell and Andersen 1985). Physiologically based pharmacodynamic (PBPD) models use mathematical descriptions of the dose-response function to quantitatively describe the relationship between target tissue dose and toxic endpoints.
No PBPK models were identified for chlordane.
3.1.6. Animal-to-Human Extrapolations
Data in rats, mice, and rabbits following oral exposure to chlordane indicate that there are some species differences in absorption, distribution, and excretion (Balba and Saha 1978; Barnett and Dorough 1974; Bondy et al. 2000; Ewing et al. 1985; Ohno et al. 1986; Poonawalla and Korte 1971; Satoh and Kikawa 1992). The available data on metabolites in human tissues and in vitro studies both indicate qualitatively that metabolism of chlordane in rats and humans is similar (Tashiro and Matsumura 1978).
3.2. CHILDREN AND OTHER POPULATIONS THAT ARE UNUSUALLY SUSCEPTIBLE
This section discusses potential health effects from exposures during the period from conception to maturity at 18 years of age in humans. Potential effects on offspring resulting from exposures of parental germ cells are considered, as well as any indirect effects on the fetus and neonate resulting from maternal exposure during gestation and lactation. Children may be more or less susceptible than adults to health effects from exposure to hazardous substances and the relationship may change with developmental age.
This section also discusses unusually susceptible populations. A susceptible population may exhibit different or enhanced responses to certain chemicals than most persons exposed to the same level of these chemicals in the environment. Factors involved with increased susceptibility may include genetic makeup, age, health and nutritional status, and exposure to other toxic substances (e.g., cigarette smoke). These parameters can reduce detoxification or excretion or compromise organ function.
Populations at greater exposure risk to unusually high exposure levels to 1,2-dibromo-3-chloropropane are discussed in Section 5.7, Populations with Potentially High Exposures.
Humans with chronic liver disease or impaired liver function may be unusually susceptible to chlordane toxicity. Infante et al. (1978) speculated on the existence of an unusually susceptible population prone to the development of blood dyscrasia (i.e., aplastic anemia and leukemias) following exposure to chlordane. Such a population was thought to have some sort of idiosyncratic response to chlordane exposure, but identification of this population was not thought to be possible. Although data are not available, humans exposed to other chemicals that induce hepatic microsomal enzymes may be unusually susceptible to chlordane, because the induced enzymes may enhance the transformation of chlordane to more highly toxic metabolites. Studies with rats show that males generally respond more than females to xenobiotic-induced enzyme induction (Kinoshita et al. 1966), but information regarding sex differences in enzyme induction in humans was not located.
Evidence in mice indicates that the fetus may be particularly susceptible to compromised immunocompetence due to altered stem cell populations of key immunoactive cells (Barnett et al. 1990a, 1990b). Infants may be unusually susceptible to a chronic seizure disorder following exposure to chlordane, particularly if they have a hereditary predisposition, such as a positive familial history of febrile convulsions (Bernad 1989).
3.3. BIOMARKERS OF EXPOSURE AND EFFECT
Biomarkers are broadly defined as indicators signaling events in biologic systems or samples. They have been classified as biomarkers of exposure, biomarkers of effect, and biomarkers of susceptibility (NAS/NRC 1989).
A biomarker of exposure is a xenobiotic substance or its metabolite(s) or the product of an interaction between a xenobiotic agent and some target molecule(s) or cell(s) that is measured within a compartment of an organism (NAS/NRC 1989). The preferred biomarkers of exposure are generally the substance itself, substance-specific metabolites in readily obtainable body fluid(s), or excreta. Biomarkers of exposure to 1,2-dibromo-3-chloropropane are discussed in Section 3.3.1. The National Report on Human Exposure to Environmental Chemicals provides an ongoing assessment of the exposure of a generalizable sample of the U.S. population to environmental chemicals using biomonitoring (see www.cdc.gov/exposurereport/). If available, biomonitoring data for 1,2-dibromo-3-chloropropane from this report are discussed in Section 5.6, General Population Exposure.
Biomarkers of effect are defined as any measurable biochemical, physiologic, or other alteration within an organism that (depending on magnitude) can be recognized as an established or potential health impairment or disease (NAS/NRC 1989). This definition encompasses biochemical or cellular signals of tissue dysfunction (e.g., increased liver enzyme activity or pathologic changes in female genital epithelial cells), as well as physiologic signs of dysfunction such as increased blood pressure or decreased lung capacity. Note that these markers are not often substance specific. They also may not be directly adverse, but can indicate potential health impairment (e.g., DNA adducts). Biomarkers of effect caused by 1,2-dibromo-3-chloropropane are discussed in Section 3.3.2.
A biomarker of susceptibility is an indicator of an inherent or acquired limitation of an organism’s ability to respond to the challenge of exposure to a specific xenobiotic substance. It can be an intrinsic genetic or other characteristic or a preexisting disease that results in an increase in absorbed dose, a decrease in the biologically effective dose, or a target tissue response. If biomarkers of susceptibility exist, they are discussed in Section 3.2, Children and Other Populations that are Unusually Susceptible.
3.3.1. Biomarkers of Exposure
It is possible to measure chlordane and/or a number of its metabolites in a variety of human tissues and fluids (i.e., blood, adipose tissue, brain, liver, kidney, milk, sebum [or skin lipids], urine, and feces). Generally, total chlordane residue levels are higher in fat and liver than in the blood (Mussalo-Rauhamaa 1991). There is no information in the literature, however, correlating the levels found in these tissues and fluids with the environmental chlordane concentrations to which the individual was exposed. Furthermore, the data do not reveal how long after exposure residues may be detected in the various body tissues and fluids.
Kawano and Tatsukawa (1982) measured “total” chlordane (cis-and trans-chlordane, heptachlor epoxide, oxychlordane, and trans-nonachlor) residues in the blood of pest control operators and nonexposed workers in Japan. Not all of these residues (e.g., heptachlor epoxide) are specific for exposure to chlordane. Levels in the blood of four of five unexposed workers were below 0.10 ng/g, the limit of detection. A level of 0.13 ng/g was reported for the fifth unexposed worker, who had lived for 2 years in a termite-treated home. Levels in the blood of 21 pest control operators ranged from 0.57 to 83 ng/g, with an average of 12 ng/g, approximately two orders of magnitude greater than levels in unexposed workers. There was no mention or indication of signs of chlordane toxicity in either the unexposed workers or pest control operators. Kutz (1983) reported mean levels in human serum for each of four components or metabolites of chlordane at <l ppb. It was not possible, however, to estimate the mean level of “total” chlordane from these data. Kutz et al. (1976, 1979) reported mean levels of heptachlor epoxide of approximately 0.1 ppm in adipose tissue samples collected from the U.S. population. Levels of oxychlordane in these samples also averaged 0.1 ppm. There appeared to be no significant change in the concentration of either chlordane metabolite over a 5-year period from 1970 to 1975. The individuals sampled by Kutz (1983) and Kutz et al. (1976, 1979) are assumed to be asymptomatic, so the reported levels were not associated with effects of chlordane toxicity. Oxychlordane has been measured at concentrations ranging from 0.002 to 0.005 mg/L in human breast milk (whole milk basis) in samples taken at random from subjects with infants assumed to be asymptomatic (Barnett et al. 1979; Strassman and Kutz 1977). In Finnish human milk samples, total chlordane residues in positive samples averaged 0.41 mg/kg of milk fat (Mussalo-Rauhamaa et al. 1988).
Several components of chlordane (trans-and cis-chlordane, trans-and cis-nonachlor, heptachlor, gamma-chlordene) were detected in the skin lipids of humans (Sasaki et al. 1991b). The samples were taken by swabbing the face with cotton soaked with 70% ethanol 3–4 hours after the face was washed with soap. Because all of the samples from inhabitants of an area known to be contaminated with chlordane contained chlordane residues, and because the profile of chlordane components in skin lipids closely resembled those in technical chlordane, the authors suggested that skin lipid analysis is a satisfactory indicator of dermal exposure to airborne chlordane, such as occurs in homes treated for termites. Oxychlordane in the skin lipids was positively correlated (correlation coefficient 0.68, p<0.01) with concentrations in internal adipose tissue. The authors concluded that the concentration of oxychlordane in skin lipids was a satisfactory indicator of body accumulation of chlordane.
A later study in monkeys confirmed that chlordane residues in skin lipids correlate closely with residues in blood (Sasaki et al. 1992). In this study, monkeys were given five consecutive, weekly, subcutaneous doses of 1 or 10 mg trans-chlordane/kg, and blood, adipose tissue and skin lipids were sampled up to 28 weeks after the last treatment for analysis for trans-chlordane and oxychlordane. trans-Chlordane concentrations in adipose tissue declined rapidly after the last dose; oxychlordane concentrations increased for about 5 weeks and leveled off. The correlation coefficients for trans-chlordane in the adipose tissue and blood, and in the adipose tissue and skin lipids were 0.93 and 0.72, respectively. The correlation coefficients for oxychlordane in the adipose tissue and blood, and in the adipose tissue and skin lipids, were 0.94 and 0.83, respectively. These data suggest that trans-chlordane concentrations in skin lipids are a satisfactory biomarker of recent exposure, and that oxychlordane concentrations in skin lipids are a satisfactory marker of previous exposure and of the body burden (in adipose tissue) of oxychlordane.
Data are available that correlate exposure to chlordane with levels of the pesticide and/or its metabolites in biological samples taken from humans. Total chlordane residues in the blood were 3–16 times higher in pesticide applicators, and 1.5–10 times higher in residents of a heavily contaminated area, where many of the houses were treated for termites with chlordane, than in residents of a relatively noncontaminated area (Wariishi and Nishiyama 1989). Recent information indicates a relatively strong correlation between the length of exposure to atmospheric chlordane in a termite-treated home and the concentration of chlordane in human milk fat (Taguchi and Yakushiji 1988); however, actual atmospheric chlordane concentrations were not reported. A relatively strong correlation between blood chlordane concentration and the number of days that a pest control operator has sprayed has been reported by Saito et al. (1986). Takamiya (1987) demonstrated a strong correlation between total chlordane residues (i.e., trans-nonachlor plus oxychlordane) in the blood of pest control operators and their duration of exposure. The atmospheric concentrations of chlordane to which these pest control operators had been exposed were not reported (Saito et al. 1986; Takamiya 1987). Kawano and Tatsukawa (1982) showed that the levels of heptachlor epoxide, oxychlordane, and trans-nonachlor in the blood of pest control operators in Japan increased with increased duration of exposure (years of employment).
Elevated serum levels of creatine phosphokinase (CPK) were measured in Japanese pest control operators exposed to chlordane (Ogata and Izushi 1991). Levels of ALT and AST were not elevated, and the investigators concluded that elevated CPK was somewhat specific for exposure to chlordane, compared with other organochlorine compounds.
3.3.2. Biomarkers of Effect
The most sensitive indicators of acute chlordane toxicity in humans are central nervous system effects including headache, confusion, behavioral aberrations, and tremors (EPA 1980a; Harrington et al. 1978). Effects on the liver appear to be the only manifestations in humans of chronic exposure to chlordane (EPA 1980a; Ogata and Izushi 1991). Changes in indicators of compromised liver function may serve as biomarkers of chlordane toxicity; however, these indicators are not specific to chlordane toxicity.
3.4. INTERACTIONS WITH OTHER CHEMICALS
Chlordane, like other organochlorine insecticides, functions as a potent inducer of hepatic microsomal enzymes. Induction of these enzymes following chlordane administration is associated with an increased rate of metabolism of many endogenous and xenobiotic compounds, including therapeutic drugs and hormones (Welch and Harrison 1966; Welch et al. 1971). Exposure to other pesticides or chemicals that induce hepatic microsomal enzymes may increase the toxicity of chlordane, probably by enhancing the transformation of chlordane to more highly toxic metabolites. For example, previous exposure to aldrin, dieldrin, or DDT increased the acute toxicity of chlordane to rats by 2.3–4.6 times (Deichmann and Keplinger 1970). When given simultaneously to rats or mice, the acute lethal effects of chlordane in combination with most pesticides appeared to be roughly additive, except that aldrin or endrin and chlordane, and methoxychlor and chlordane were more than additive in mice (Keplinger and Deichmann 1967).
The acute toxicity of chlordane in rats increased when rats were fed protein-deficient diets (Boyd and Taylor 1969). Chlordane treatment has also been demonstrated to enhance the hepatotoxic effects produced by carbon tetrachloride in rats, as indicated by its effect on ALT levels, presumably by inducing the metabolism of carbon tetrachloride to its toxic metabolite (Mahon et al. 1978; Stenger et al. 1975). On the other hand, chlordane provided some protection against carbon tetrachloride-induced liver necrosis in rats, possibly by inducing a type of cytochrome P-450 with diminished ability to metabolize carbon tetrachloride to its toxic metabolite (Mahon et al. 1978). Pretreatment of rats with chlordane accelerated the metabolism of lindane, presumably by inducing the metabolism of lindane to its toxic metabolite (Chadwick et al. 1977).
Chlordane-induced testicular effects in mice were markedly increased when lead oxide was coadministered (Al-Omar et al. 2000).
- TOXICOKINETICS, SUSCEPTIBLE POPULATIONS, BIOMARKERS, CHEMICAL INTERACTIONS - Tox...TOXICOKINETICS, SUSCEPTIBLE POPULATIONS, BIOMARKERS, CHEMICAL INTERACTIONS - Toxicological Profile for Chlordane
Your browsing activity is empty.
Activity recording is turned off.
See more...