This work is licensed under a Creative Commons Attribution-NonCommercial-NoDerivs 3.0 Unported License. To view a copy of this license, visit http://creativecommons.org/licenses/by-nc-nd/3.0/
NCBI Bookshelf. A service of the National Library of Medicine, National Institutes of Health.
Kozak JA, Putney JW Jr., editors. Calcium Entry Channels in Non-Excitable Cells. Boca Raton (FL): CRC Press/Taylor & Francis; 2018. doi: 10.1201/9781315152592-9
9.1. Introduction
Ca2+ signaling is crucial in a variety of physiological/pathological processes associated with acidosis and alkalosis. In particular, capacitive Ca2+ entry [1] through the Ca2+ release-activated Ca2+ (CRAC) channels [2] plays an essential role in mediating intracellular and extracellular acidification and alkalinization-induced functional changes. Physiologically, intracellular alkalinization is associated with various physiological functions such as activity-dependent membrane depolarization [3], oocyte maturation [4], oocyte fertilization, sperm activation [5–7], mast cell degranulation [8], smooth muscle contraction [9], and growth factor-induced cell proliferation, differentiation, migration, and chemotaxis [10] (Figure 9.1). Pathologically, intracellular alkalinization is a hallmark of malignant cells associated with tumor progression [11,12], whereas acidic intracellular pH (pHi) has been shown to promote apoptosis [13]. Additionally, extracellular acidosis is another hallmark of tumor progression [11,12], and also a major cause of immunodeficiency in clinical acidosis due to impaired lymphocyte proliferation and cytotoxicity [14]. Furthermore, extracellular low pH, which occurs under injury and ischemic conditions, inhibits a number of cellular responses, including cytosolic- and membrane-associated enzyme activities as well as ion transport and ion channel activities [14].

Figure 9.1
Diagram illustrating CRAC channel-mediated Ca2+ signaling and pathophysiological processes associated with intracellular and extracellular acidification and alkalinization. Top: Regulation of ICRAC by acidic and basic extracellular pH and physiological/pathological (more...)
Like many other ion channels, native ICRAC is inhibited by acidic but potentiated by basic extracellular or intracellular solutions in various cell types including macrophages [15], Jurkat T-lymphocytes [16], SH-SY5Y neuroblastoma cells [17], and smooth muscle cells [18]. Moreover, it has been shown that intracellular alkalinization-induced increase in intracellular Ca2+ is essential for platelet aggregation in response to thrombin [19]. Similarly, extracellular acidosis-induced inhibition, as well as alkalosis-induced stimulation of platelet aggregation, is mediated by changes in store-operated Ca2+ entry [20]. Furthermore, store-operated Ca2+ entry was suggested to mediate intracellular alkalinization in neutrophils [21], and a variety of growth factors have been demonstrated to induce cytosolic alkalinization together with Ca2+ entry [8]. Given the essential role of ICRAC in acidosis- and alkalosis-associated physiological and pathological processes, there is a great interest in understanding the molecular basis of CRAC channel regulation by intracellular and extracellular pH.
The discovery of the molecular basis of ICRAC and its gating mechanisms [22–29] provides a great opportunity to investigate the molecular basis of pH regulation of ICRAC. CRAC channel activity can be influenced by alterations of either the coupling of Orai and STIM subunits or biophysical properties of the pore-forming Orai subunit. Thus, pH regulation of ICRAC can be mediated by influencing this coupling process and/or by changing the biophysical characteristics of the pore-forming Orai subunits. Using Ca2+ imaging techniques, a previous study suggested that cytosolic alkalinization may lead to store depletion and therefore activate Orai1/STIM1 channels [30], whereas several other studies demonstrated that cytosolic alkalinization-induced Ca2+ release is not always related to Ca2+ entry [31–33]. Moreover, intracellular low pH caused by oxidative stress may result in uncoupling of Orai1 and STIM1, thereby inhibiting CRAC currents [34]. Recent studies have focused on using heterologously expressed Orai/STIM channels to fully understand how these channels are regulated by high and low internal and external pH, as well as the molecular basis of pH regulation of Orai/STIM [35–37].
This chapter summarizes recent advances in our understanding of pH regulation of ICRAC by focusing on how Orai/STIM channels are regulated by pH and the potential molecular basis of pH regulation. We summarize the essential methods required to investigate the influence of pH on Orai/STIM channel function as well as molecular mechanisms of pH regulation and highlight future directions in this exciting research field.
9.2. Basic Methods
9.2.1. Cell Culture and Transfection
HEK-293 cells are cultured in DMEM/F12 medium supplemented with 10% FBS, penicillin, and streptomycin at 37°C in a humidity-controlled incubator with 5% CO2. Cells are transiently transfected with wild-type (WT) Orai channels or its mutants and WT STIM1 using Lipofectamine 2000 (Invitrogen). 5 μL Lipofectamine 2000 is used for transfection of cells in a 35 mm culture dish. The GFP-containing pEGFP vector is transfected as a mock control. Successfully transfected cells can be identified by their green fluorescence when illuminated at 488 nm (eGFP excitation 488 nm, emission 510 nm). We cotransfected Orai1 (Addgene #12199), Orai2 (Addgene #16369), or Orai3 (Addgene #16370) together with STIM1 (Addgene #19754). After 24–36 h of transfection, Orai/STIM channel currents can be recorded using patch-clamp technique at room temperature (20°C–25°C).
Native ICRAC can be readily recorded from various cell types including rat basophilic leukemia cells (RBL-2H3) [23]. RBL-2H3 cells are cultured using conditions similar to those of culturing HEK-293 cells.
9.2.2. Site-Directed Mutagenesis of Orai
Mutations at selected residues can be generated using site-directed mutagenesis. QuikChange™ Site-Directed Mutagenesis Kit (Agilent) is used for single, double, or triple mutations if the residues are close to each other [37–40]. If the amino acid residues to be mutagenized are distant from each other, one mutation can be generated first, and the mutant construct can serve as a template for the second and third mutations. All mutant constructs are sequenced to confirm that the mutagenized sequence has been generated and that there are no unexpected mutations introduced. We generated 6 mutations of Orai1 for investigating extracellular pH sensitivity, and 11 mutations for evaluating intracellular pH sensitivity [37].
9.2.3. Orai/STIM Current Recording by Patch-Clamp Electrophysiology
Orai/STIM currents can be recorded using patch-clamp methods (see Chapter 1). We use conventional whole-cell patch-clamp, an Axopatch 200B amplifier, Digidata 1320A, and pClamp9 software (Molecular Devices) for data acquisition and analysis. Data are usually digitized at 10 or 20 kHz and digitally filtered off-line at 1 kHz. Patch electrodes are pulled using P-97 (Sutter Instrument) puller from borosilicate glass capillaries and fire-polished to a resistance of 2–4 MΩ when filled with internal solutions. Series resistance (RS) is compensated up to 90% to reduce RS errors to <5 mV. Cells with RS < 10 MΩ are used for analysis [41].
9.2.4. Ca2+ Imaging
Since Orai/STIM channels are highly Ca2+ selective, regulation of channel activity can also be evaluated by directly measuring Ca2+ influx. We use ratiometric calcium imaging to detect changes of intracellular Ca2+ concentration (see Chapters 1 and 16). Cells plated on 25 mm glass coverslips are loaded with 5 μM Fura-2/AM (Molecular Probes) for 45 min. Unincorporated dye is washed away using a HEPES-buffered saline solution (HBSS) containing (in mM) 1.2 MgCl2, 1.3 CaCl2, 1.2 KH2PO4, 4.7 KCl, 140 NaCl, 20 HEPES, and 10 glucose (pH 7.4). Changes in intracellular Ca2+ concentration can be monitored as changes of the ratio of fluorescence intensity at emission wavelength 510 nm and excitation wavelengths at 340 and 380 nm. Alternating the excitation wavelengths between 340 and 380 nm can be achieved using DG4 (Sutter), and fluorescence signals are acquired using CoolSNAP HQ2 (Photometrics). Data are analyzed off-line using NIS-Elements (Nikon). Changes in cytosolic Ca2+ concentration can be calculated using NIS-Elements. We also use 1 μM ionomycin as an internal control to monitor dye loading variability among different experimental groups. Changes of F340/380 induced by various experimental manipulations are normalized to F340/F380 elicited by 1 μM ionomycin as previously reported [42].
9.2.5. Measurement of Changes in Intracellular pH by Ratiometric pHi Imaging
Several ion channels are not only modulated by protons but also permeable to these ions at acidic external pH, which may cause changes in intracellular pH [43,44]. Intracellular pH changes can be monitored by ratiometric pH imaging [45,46] using membrane-permeable pH indicators, such as 2′,7′-bis-(2-carboxyethyl)-5-(and-6)-carboxyfluorescein (BCECF/AM), or the genetically encoded ratiometric pH indicator SypHer, as demonstrated in recent studies [47,48]. For BCECF/AM, the ratio of fluorescence intensity detected at 535 nm when BCECF is excited at 490 versus 440 nm is used for calculation of intracellular pH. For calibration, cells are loaded with 0.5–0.8 μM BCECF/AM (Molecular Probes) at 37°C for 30 min. Excitation spectra of free and protonated BCECF can be determined in HEK-293 cells equilibrated in a high K+ solution containing 128 mM KCl, 10 mM NaCl, 1 mM MgCl2, 1 mM CaCl2, 10 mM glucose, 0.2% (w/v) bovine serum albumin, and 10 mm HEPES, supplemented with nigericin and monensin (10 μM each) and pH adjusted to 9.0 or 4.0, respectively [45]. The relative concentrations of free and protonated BCECF in the probe are calculated by multivariate linear regression analysis [49] to analyze the spectral properties of experimental data with stored spectra of the free and protonated indicator. Experimental data can then be converted to pHi from the equation pHi = 6.97 - log([BCECF-H]/[BCECF-]) [45].
9.3. Regulation of Orai/STIM Channel by Internal and External pH
Many ion channels and transporters are modulated by changes of internal and external pH. Patch-clamp electrophysiology is a commonly used method to evaluate the pH regulation of ion channel activities, whereas mutation of the presumptive amino acid residues responsible for pH sensitivity is an effective approach to understand the molecular mechanisms of pH regulation.
9.3.1. Influence of Changes of Internal and External pH on Orai/STIM Channel Activation
A unique feature of Orai/STIM channels is that the activation of these channels involves coupling of Orai and STIM proteins. To determine if activation of Orai/STIM channels is influenced by pH changes, perforated patch recording can be performed in order to maintain the integrity of intracellular contents. We used pipette solutions containing 145 mM Cs-methanesulfonate (CsSO3CH3), 8 NaCl, 3 MgCl2, 10 EGTA, and 10 HEPES (pH adjusted to 7.4 with CsOH) and the antibiotic nystatin (180 μg/mL) for this recording configuration. Increases of cytoplasmic pH can be achieved by superfusing the cells with 30 mM NH4Cl added to Tyrode’s buffer [37]. We found that changes of either intracellular or extracellular pH can regulate but not activate Orai/STIM channels [37].
9.3.2. Orai/STIM Channel Regulation by External Protons
The native ICRAC is inhibited by extracellular low pH in macrophages [15]. To test pH effects on Orai/STIM channels heterologously expressed in HEK-293 cells, we passively depleted the calcium stores to activate Orai/STIM using pipette solutions with free Ca2+ concentration chelated to subnanomolar levels (calculated with MaxChelator software http://web.stanford.edu/∼cpatton/webmaxcS.htm). We used divalent-free (DVF) solution at various pH values as the extracellular solution. The internal pipette solution for whole-cell current recordings contained 145 mM Cs-methanesulfonate (CsSO3CH3), 8 mM NaCl, 10 EGTA, and 20 HEPES, with pH 7.2 adjusted with CsOH. MgCl2 (3 mM) was included in the pipette solution to block endogenous TRPM7 currents present in the HEK-293 cells or other cell types [50]. The standard extracellular Tyrode’s buffer for whole-cell recordings contained (mM) 145 NaCl, 5 KCl, 2 CaCl2, 1 MgCl2, 10 HEPES, and 10 glucose; pH was adjusted to 7.4 with NaOH. For altering the pH, HEPES, MES (2-(N-morpholino)ethanesulfonic acid) and citric acid, and Tris can be used as the pH buffer for solutions at pH 6–8, pH < 6.0, and pH > 8.0, respectively. We used HCl and 5 mM MES for acidic extracellular solutions and N-methyl-d-glucamine (NMDG) for basic extracellular solutions. Divalent-free external solution contained (mM) 145 Na-SO3CH3, 20 HEPES, 5 EGTA, 2 EDTA, and 10 glucose, with free [Ca2+] ≤ 1 nM and free [Mg2+] ≈ 10 nM estimated at pH 7.4. When higher Ca2+ concentrations are required in the external solution, Na+ concentration is reduced accordingly to keep the same osmolality. For extracellular acidic solutions, pH was adjusted with citric acid and MES, and for alkaline solutions, NMDG was used in order to maintain the same monovalent cation concentrations [51]. NMDG used for adjusting pH in solutions did not significantly influence Orai1/STIM1 current amplitudes by itself [37].
Orai/STIM channels can be activated by active or passive store depletion (Chapter 1). We activated the channels by passive store deletion after the pipette solution diffused into the cells. Moreover, we used DVF external solution since Na+-carried CRAC current is larger than the Ca2+ current in normal Tyrode’s [23]. ICRAC is elicited by a command voltage ramp ranging from -120 to +100 mV of 200 ms duration. Inward current amplitude was used to evaluate the effects of various external pH solutions on Orai/STIM channel activity. NMDG can be used to monitor leak current when switching between various external solutions. Since the effect of pH on these channels is reversible, ICRAC in one cell can be used to test the effects of various external pH solutions to avoid current amplitude variations among cells [37]. A complete recovery after each pH stimulation can be observed by superfusing the cells with normal Tyrode’s solution at pHo 7.4 (Figure 9.3).
For both native ICRAC and overexpressed Orai/STIM, acidic pH inhibited whereas basic pH potentiated the current amplitude [35–37]. The effects of external protons on Orai1/STIM1, Orai2/STIM1, and Orai3/STIM1 are similar [37] and also similar to their effects on ICRAC in RBL-2H3 cells. Moreover, ICRAC arising from overexpression of different Orai isoforms with STIM2 also showed a similar response to external protons [36].
9.3.3. Regulation of Orai/STIM Channel Activity by Internal Protons
The effects of intracellular pH (pHi) can be tested by fixing pipette solutions at various pH. Acidic pHi can be adjusted with citrate and MES, and basic pHi can be adjusted with CsOH [37]. Alternatively, internal pH changes can be achieved by superfusing the cells with ammonium [50,52]. For current recording under various pHi conditions, normal Tyrode’s or DVF solution can be used as the external solution. We used passive store depletion by including high concentrations of Ca2+ chelator in the pipette solution to activate ICRAC and used DVF as the external solution to obtain larger ICRAC currents [1]. At acidic pHi, ICRAC amplitude recorded from overexpression of Orai1/STIM1 is significantly smaller than that at neutral pHi 7.2; whereas at basic pHi, ICRAC current amplitude of Orai1/STIM1 is much larger than at neutral pHi 7.2. The increased channel activity at basic pHi supports the notion that CRAC channel-mediated Ca2+ signaling may play a crucial role in various physiological functions (Figure 9.1) including alkalinization associated with oocyte maturation and fertilization [6,53–55] as well as mast cell degranulation [8].
The effects of internal pH on Orai/STIM channel activity can also be assessed by the Ca2+ imaging approach, as conventional patch-clamp can only test one pHi value in a cell. Store depletion can be induced by thapsigargin, a SERCA pump inhibitor [1,23]. After store depletion and activation of Orai/STIM channels, increases of intracellular pH can be achieved by adding different concentrations of NH4Cl [50,52], whereas acidic pHi can be produced by adding NaHCO3 [56]. The concentrations of NH4Cl or NaHCO3 required for a specific pHi can be defined from ratiometric pH imaging [45,46].
9.3.4. Evaluation of Proton Permeation
For Ca2+-permeable channels, external protons not only modulate ion channel activity or channel gating but also become permeant cations for some ion channels, such as TRPM7 [44,57,58] and TRPV1 [45]. Proton permeation can be determined by electrophysiology or measurement of intracellular pH. To determine if protons are permeant cations by patch-clamp, Na+ and K+ cations in the external solution can be replaced with NMDG and the concentration of protons systematically altered. For TRPM7, the channel activity is potentiated by external protons [57–59], and the channel becomes permeable to protons at acidic pHo. A noticeable proton current (∼200 pA) through TRPM7 channels can be readily recorded at pHo 4.0 [57,59]. Proton permeation can also be determined by measuring intracellular pH changes. Proton permeation through TRPV1 leads to cellular acidification at pHo 5.5 [45]. TRPM2 was also suggested to be permeable to protons based on the altered I-V shape [60]. Orai/STIM channel permeability to protons is yet to be determined.
9.4. Molecular Mechanisms of pH Sensitivity
Protons can regulate ion channel activities by changing channel permeation properties or gating properties. For Orai/STIM channels, channel gating is governed by coupling of Orai and STIM proteins [23]. Since changes of pH cannot activate Orai/STIM without store depletion, we reasoned that it is unlikely that protons regulate coupling of Orai and STIM proteins [35–37]. Therefore, we focused on how intracellular and extracellular protons regulate Orai/STIM channel activity through altering the function of the pore-forming Orai subunits.
9.4.1. Key Amino Acid Residues Responsible for Extracellular pH Sensitivity
To understand the mechanism by which external protons regulate Orai/STIM channel activities, mutations of candidate amino acid residues were generated by site-directed mutagenesis. We chose the negatively charged residues in the outer mouth of the pore (D110, D112, and D114) as well as the residues along the channel pore (E106 and E190) (Figure 9.2) and neutralized those residues to evaluate their sensitivity to extracellular protons. Among the residues proposed for external pH sensitivity, E106, D110, and E190 are conserved in Orai1, Orai2, and Orai3, albeit D110 in Orai1 is replaced by E110 in Orai2 and Orai3 (Figure 9.2). The residues D112 and D114 of Orai1, however, are replaced with neutral residues Q112 and Q114 in Orai2 but are conserved as negatively charged residues in Orai3 with D112 and E114 (Figure 9.2). In addition to neutralizing the negatively charged residues, we also generated mutants E106D and E190D that preserved charge, given that the length of the side chain of the residues may also contribute to altered channel activity. Mutants of Orai1 were then coexpressed with STIM1 for evaluation of channel activity by patch-clamp. The mutants and the oligo primers used for mutagenesis are listed in Table 9.1.
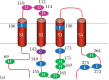
Figure 9.2
Mutations of intracellular and extracellular residues and pH sensitivity.
Table 9.1
Primers Used for Site-Directed Mutagenesis of Putative Residues Responsible for Internal and External pH Sensitivity.
To compare the pH sensitivity of WT Orai1/STIM1 to mutant channels, dose-response curves can be constructed by measuring the Orai/STIM current amplitude for every pHo. The dose-response curve can be fitted with the Boltzmann equation to obtain the proton concentration required for inducing 50% of maximal response, EC50 (or IC50) or pKa (Figure 9.3). A shift of dose-response curves and changes of pKa indicate altered pH sensitivity [37]. Using dose-response curves to evaluate changes of pH sensitivity allows evaluation of both the potency and efficacy of protons in Orai/STIM channels.
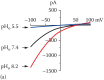
Figure 9.3
Regulation of Orai/STIM channel activity by internal and external pH.
Using monovalent cations as charge carriers is a common approach to study pH effects on different ion channels, including Ca2+-selective ion channels [44,61–67]. Interestingly, for Orai/STIM channels, the pHo sensitivity is determined by E190 when Na+ is the charge carrier but by E106 when Ca2+ is the charge carrier [37]. Mutants D110N and D112N/D114N produced minor changes in potentiation of current amplitude at very high pHo but did not change pKa or shift proton dose-response curves.
9.4.2. Molecular Basis of Intracellular pH Sensitivity of Orai/STIM Channels
To identify the amino acid residues responsible for intracellular pH sensitivity of Orai1/STIM1 channels, we made a series of mutations at the N- and C-termini and at the loop between TM2 and TM3 of Orai1 (Figure 9.2). We chose titratable residues such as histidine and glutamic acid, as well as cysteine residues, which have been shown to be involved in internal pH sensing in other channels [68–70], and generated 10 single or double mutants by neutralizing positive or negative charges.
Since the effects of intracellular pH can only be tested one value at a time, an initial screening of the mutants responsible for altered pHi sensitivity can be performed by using a high and a low pH stimulation. For example, pHi 9.0 and 5.5 can induce maximal potentiation and inhibition of Orai/STIM channel activities, respectively. The effects of pHi 9.0 and pHi 5.5 on different mutants were compared to those of WT Orai/STIM channels, and the mutants that showed altered pH response were further analyzed by testing concentration-dependent effects using additional pH values. pKa can be obtained by analyzing concentration-dependent effects of protons on the mutant channels. Among all the mutants tested, H115 appears to be the residue governing internal pH regulation of Orai1/STIM1 channel activities.
9.5. Functional Assessment of pH Regulation of Orai/STIM Channels
ICRAC is involved in various physiological/pathological functions. An increase in ICRAC is associated with various cellular functions and can easily be detected. For example, the increase of ICRAC by alkalinization can lead to mast cell release of histamine, which is readily measureable [8]. Mast cells can be isolated from peritoneal cavities of rats [71] or mice and purified using isotonic Percoll centrifugation at 400 x g for ∼10 min. Purified mast cells are then maintained in a cell culture incubator. NH4Cl is used for inducing cellular alkalinization. After 10 min incubation with NH4Cl, supernatants of cell culture are collected for the histamine release assay. Since histamine may not be completely released from cells into the supernatant, mast cells can also be collected for residual histamine measurement. Histamine concentrations can be determined using commercially available ELISA-based histamine kits or the conventional histamine assay method [72].
9.6. Summary and Future Research Directions
As a major Ca2+ channel in many non-excitable cell types, the CRAC channel plays a pivotal role in various physiological functions [23]. In acidosis and alkalosis, ICRAC is likely a major mediator of Ca2+ signaling in several pathophysiological processes [11–13]. For example, the increased ICRAC by cytosolic alkalinization may contribute to tumor progression [11,12], whereas reduced ICRAC in acidosis may cause impaired lymphocyte functions thereby leading to immunodeficiency associated with clinical acidosis [14]. Therefore, understanding the molecular basis of pH regulation on Orai/STIM channels may provide important information for identifying pharmacological tools to modulate Orai/STIM channel activity under acidic and basic pH conditions. Although we find that internal and external protons modulate Orai/STIM channel activities after the channels are activated, a thorough investigation of pH influence on the coupling of Orai and STIM subunits and channel gating is necessary to fully understand how the activation process of Orai1/STIM is influenced by pH changes. Furthermore, the results of pH regulation of Orai/STIM channels point toward the importance of exploring the therapeutic potential of manipulating ICRAC in reverting or preventing pathological consequences of acidosis and alkalosis, such as immunodeficiency and tumor progression.
Acknowledgments
We would like to thank the current and former lab members for helpful discussions. This work was supported by the National Institutes of Health, National Heart, Lung and Blood Institute (NHLBI, 2R01HL078960), and American Heart Association (AHA, 16GRNT26430113) to L.Y.
References
- 1.
- Parekh, A.B. and Putney, J.W., Jr. 2005. Store-operated calcium channels. Physiol Rev 85:757-810. [PubMed: 15788710]
- 2.
- Hoth, M. and Penner, R. 1992. Depletion of intracellular calcium stores activates a calcium current in mast cells. Nature 355:353-356. [PubMed: 1309940]
- 3.
- Lyall, V. and Biber, T.U. 1994. Potential-induced changes in intracellular pH. Am J Physiol 266:F685-F696. [PubMed: 7515581]
- 4.
- Mahnensmith, R.L. and Aronson, P.S. 1985. The plasma membrane sodium-hydrogen exchanger and its role in physiological and pathophysiological processes. Circ Res 56:773-788. [PubMed: 2988813]
- 5.
- Lishko, P.V. and Kirichok, Y. 2010. The role of Hv1 and CatSper channels in sperm activation. J Physiol 588:4667-4672. [PMC free article: PMC3010136] [PubMed: 20679352]
- 6.
- Webb, D.J. and Nuccitelli, R. 1981. Direct measurement of intracellular pH changes in Xenopus eggs at fertilization and cleavage. J Cell Biol 91:562-567. [PMC free article: PMC2111970] [PubMed: 6796594]
- 7.
- Nuccitelli, R., Webb, D.J., Lagier, S.T., and Matson, G.B. 1981. 31P NMR reveals increased intracellular pH after fertilization in Xenopus eggs. Proc Natl Acad Sci USA 78:4421-4425. [PMC free article: PMC319802] [PubMed: 6945594]
- 8.
- Alfonso, A., Cabado, A.G., Vieytes, M.R., and Botana, L.M. 2000. Calcium-pH crosstalks in rat mast cells: Cytosolic alkalinization, but not intracellular calcium release, is a sufficient signal for degranulation. Br J Pharmacol 130:1809-1816. [PMC free article: PMC1572257] [PubMed: 10952669]
- 9.
- Heaton, R.C., Taggart, M.J., and Wray, S. 1992. The effects of intracellular and extracellular alkalinization on contractions of the isolated rat uterus. Pfügers Arch 422:24-30. [PubMed: 1437523]
- 10.
- Srivastava, J., Barber, D.L., and Jacobson, M.P. 2007. Intracellular pH sensors: Design principles and functional significance. Physiology (Bethesda) 22:30-39. [PubMed: 17289928]
- 11.
- Reshkin, S.J., Bellizzi, A., Caldeira, S., Albarani, V., Malanchi, I., Poignee, M., Alunni-Fabbroni, M., Casavola, V., and Tommasino, M. 2000. Na+/H+ exchanger-dependent intracellular alkalinization is an early event in malignant transformation and plays an essential role in the development of subsequent transformation-associated phenotypes. FASEB J 14:2185-2197. [PubMed: 11053239]
- 12.
- Lindner, D. and Raghavan, D. 2009. Intra-tumoural extra-cellular pH: A useful parameter of response to chemotherapy in syngeneic tumour lines. Br J Cancer 100:1287-1291. [PMC free article: PMC2676543] [PubMed: 19367285]
- 13.
- Lagadic-Gossmann, D., Huc, L., and Lecureur, V. 2004. Alterations of intracellular pH homeostasis in apoptosis: Origins and roles. Cell Death Differ 11:953-961. [PubMed: 15195071]
- 14.
- Lardner, A. 2001. The effects of extracellular pH on immune function. J Leukoc Biol 69:522-530. [PubMed: 11310837]
- 15.
- Malayev, A. and Nelson, D.J. 1995. Extracellular pH modulates the Ca2+ current activated by depletion of intracellular Ca2+ stores in human macrophages. J Membr Biol 146:101-111. [PubMed: 7563033]
- 16.
- Guse, A.H., Roth, E., and Emmrich, F. 1994. Ca2+ release and Ca2+ entry induced by rapid cytosolic alkalinization in Jurkat T-lymphocytes. Biochem J 301(Pt 1):83-88. [PMC free article: PMC1137146] [PubMed: 8037695]
- 17.
- Laskay, G., Kalman, K., Van Kerkhove, E., Steels, P., and Ameloot, M. 2005. Store-operated Ca2+-channels are sensitive to changes in extracellular pH. Biochem Biophys Res Commun 337:571-579. [PubMed: 16198307]
- 18.
- Iwasawa, K., Nakajima, T., Hazama, H., Goto, A., Shin, W.S., Toyo-oka, T., and Omata, M. 1997. Effects of extracellular pH on receptor-mediated Ca2+ influx in A7r5 rat smooth muscle cells: Involvement of two different types of channel. J Physiol 503(Pt 2):237-251. [PMC free article: PMC1159859] [PubMed: 9306269]
- 19.
- Siffert, W. and Akkerman, J.W. 1987. Activation of sodium-proton exchange is a prerequisite for Ca2+ mobilization in human platelets. Nature 325:456-458. [PubMed: 3027576]
- 20.
- Marumo, M., Suehiro, A., Kakishita, E., Groschner, K., and Wakabayashi, I. 2001. Extracellular pH affects platelet aggregation associated with modulation of store-operated Ca2+ entry. Thromb Res 104:353-360. [PubMed: 11738078]
- 21.
- Sandoval, A.J., Riquelme, J.P., Carretta, M.D., Hancke, J.L., Hidalgo, M.A., and Burgos, R.A. 2007. Store-operated calcium entry mediates intracellular alkalinization, ERK1/2, and Akt/PKB phosphorylation in bovine neutrophils. J Leukoc Biol 82:1266-1277. [PubMed: 17684040]
- 22.
- Roos, J., DiGregorio, P.J., Yeromin, A.V., Ohlsen, K., Lioudyno, M., Zhang, S., Safrina, O.et al. 2005. STIM1, an essential and conserved component of store-operated Ca2+ channel function. J Cell Biol 169:435-445. [PMC free article: PMC2171946] [PubMed: 15866891]
- 23.
- Prakriya, M. and Lewis, R.S. 2015. Store-operated calcium channels. Physiol Rev 95:1383-1436. [PMC free article: PMC4600950] [PubMed: 26400989]
- 24.
- Yeromin, A.V., Zhang, S.L., Jiang, W., Yu, Y., Safrina, O., and Cahalan, M.D. 2006. Molecular identification of the CRAC channel by altered ion selectivity in a mutant of Orai. Nature 443:226-229. [PMC free article: PMC2756048] [PubMed: 16921385]
- 25.
- Prakriya, M., Feske, S., Gwack, Y., Srikanth, S., Rao, A., and Hogan, P.G. 2006. Orai1 is an essential pore subunit of the CRAC channel. Nature 443:230-233. [PubMed: 16921383]
- 26.
- Vig, M., Peinelt, C., Beck, A., Koomoa, D.L., Rabah, D., Koblan-Huberson, M., Kraft, S.et al. 2006. CRACM1 is a plasma membrane protein essential for store-operated Ca2+ entry. Science 312:1220-1223. [PMC free article: PMC5685805] [PubMed: 16645049]
- 27.
- Park, C.Y., Hoover, P.J., Mullins, F.M., Bachhawat, P., Covington, E.D., Raunser, S., Walz, T., Garcia, K.C., Dolmetsch, R.E., and Lewis, R.S. 2009. STIM1 clusters and activates CRAC channels via direct binding of a cytosolic domain to Orai1. Cell 136:876-890. [PMC free article: PMC2670439] [PubMed: 19249086]
- 28.
- Yuan, J.P., Zeng, W., Dorwart, M.R., Choi, Y.J., Worley, P.F., and Muallem, S. 2009. SOAR and the polybasic STIM1 domains gate and regulate Orai channels. Nat Cell Biol 11:337-343. [PMC free article: PMC2663385] [PubMed: 19182790]
- 29.
- Wang, X., Wang, Y., Zhou, Y., Hendron, E., Mancarella, S., Andrake, M.D., Rothberg, B.S., Soboloff, J., and Gill, D.L. 2014. Distinct Orai-coupling domains in STIM1 and STIM2 define the Orai-activating site. Nat Commun 5:3183. [PMC free article: PMC3995141] [PubMed: 24492416]
- 30.
- Li, S., Hao, B., Lu, Y., Yu, P., Lee, H.C., and Yue, J. 2012. Intracellular alkalinization induces cytosolic Ca2+ increases by inhibiting sarco/endoplasmic reticulum Ca2+-ATPase (SERCA). PLoS One 7:e31905. [PMC free article: PMC3288054] [PubMed: 22384096]
- 31.
- Yodozawa, S., Speake, T., and Elliott, A. 1997. Intracellular alkalinization mobilizes calcium from agonist-sensitive pools in rat lacrimal acinar cells. J Physiol 499(Pt 3):601-611. [PMC free article: PMC1159279] [PubMed: 9130157]
- 32.
- Nitschke, R., Riedel, A., Ricken, S., Leipziger, J., Benning, N., Fischer, K.G., and Greger, R. 1996. The effect of intracellular pH on cytosolic Ca2+ in HT29 cells. Pflügers Arch 433:98-108. [PubMed: 9019738]
- 33.
- Dettbarn, C. and Palade, P. 1991. Effects of alkaline pH on sarcoplasmic reticulum Ca2+ release and Ca2+ uptake. J Biol Chem 266:8993-9001. [PubMed: 1709160]
- 34.
- Mancarella, S., Wang, Y., Deng, X., Landesberg, G., Scalia, R., Panettieri, R.A., Mallilankaraman, K., Tang, X.D., Madesh, M., and Gill, D.L. 2011. Hypoxia-induced acidosis uncouples the STIM-Orai calcium signaling complex. J Biol Chem 286:44788-44798. [PMC free article: PMC3247972] [PubMed: 22084246]
- 35.
- Scrimgeour, N.R., Wilson, D.P., and Rychkov, G.Y. 2012. Glu106 in the Orai1 pore contributes to fast Ca2+-dependent inactivation and pH dependence of Ca2+ release-activated Ca2+ (CRAC) current. Biochem J 441:743-753. [PubMed: 21967483]
- 36.
- Beck, A., Fleig, A., Penner, R., and Peinelt, C. 2014. Regulation of endogenous and heterologous Ca2+ release-activated Ca2+ currents by pH. Cell Calcium 56:235-243. [PMC free article: PMC4162834] [PubMed: 25168908]
- 37.
- Tsujikawa, H., Yu, A.S., Xie, J., Yue, Z., Yang, W., He, Y., and Yue, L. 2015. Identification of key amino acid residues responsible for internal and external pH sensitivity of Orai1/STIM1 channels. Sci Rep 5:16747. [PMC free article: PMC4649748] [PubMed: 26576490]
- 38.
- Li, M., Du, J., Jiang, J., Ratzan, W., Su, L.T., Runnels, L.W., and Yue, L. 2007. Molecular determinants of Mg2+ and Ca2+ permeability and pH sensitivity in TRPM6 and TRPM7. J Biol Chem 282:25817-25830. [PMC free article: PMC3239414] [PubMed: 17599911]
- 39.
- Xie, J., Sun, B., Du, J., Yang, W., Chen, H.C., Overton, J.D., Runnels, L.W., and Yue, L. 2011. Phosphatidylinositol 4,5-bisphosphate (PIP(2)) controls magnesium gatekeeper TRPM6 activity. Sci Rep 1:146. [PMC free article: PMC3238349] [PubMed: 22180838]
- 40.
- Du, J., Xie, J., and Yue, L. 2009. Modulation of TRPM2 by acidic pH and the underlying mechanisms for pH sensitivity. J Gen Physiol 134:471-488. [PMC free article: PMC2806426] [PubMed: 19917732]
- 41.
- Yue, L., Navarro, B., Ren, D., Ramos, A., and Clapham, D. 2002. The cation selectivity filter of the bacterial sodium channel, NaChBac. J Gen Physiol 160:845-853. [PMC free article: PMC2229573] [PubMed: 12451053]
- 42.
- Du, J., Xie, J., Zhang, Z., Tsujikawa, H., Fusco, D., Silverman, D., Liang, B., and Yue, L. 2010. TRPM7-mediated Ca2+ signals confer fibrogenesis in human atrial fibrillation. Circ Res 106:992-1003. [PMC free article: PMC2907241] [PubMed: 20075334]
- 43.
- DeCoursey, T.E. 2013. Voltage-gated proton channels: Molecular biology, physiology, and pathophysiology of the H(V) family. Physiol Rev 93:599-652. [PMC free article: PMC3677779] [PubMed: 23589829]
- 44.
- Sabovcik, R., Li, J., Kucera, P., and Prod'hom, B. 1995. Extracellular protons modulate the Ca2+ block of a Ca2+-blockable monovalent cation channel in chick embryo. Pflügers Arch 430:599-601. [PubMed: 7491290]
- 45.
- Hellwig, N., Plant, T.D., Janson, W., Schäfer, M., Schultz, G., and Schaefer, M. 2004. TRPV1 acts as proton channel to induce acidification in nociceptive neurons. J Biol Chem 279:34553-34561. [PubMed: 15173182]
- 46.
- Han, J. and Burgess, K. 2010. Fluorescent indicators for intracellular pH. Chem Rev 110:2709-2728. [PubMed: 19831417]
- 47.
- Matlashov, M.E., Bogdanova, Y.A., Ermakova, G.V., Mishina, N.M., Ermakova, Y.G., Nikitin, E.S., Balaban, P.M.et al. 2015. Fluorescent ratiometric pH indicator SypHer2: Applications in neuroscience and regenerative biology. Biochim Biophys Acta 1850:2318-2328. [PMC free article: PMC4587288] [PubMed: 26259819]
- 48.
- Shirmanova, M.V., Druzhkova, I.N., Lukina, M.M., Matlashov, M.E., Belousov, V.V., Snopova, L.B., Prodanetz, N.N., Dudenkova, V.V., Lukyanov, S.A., and Zagaynova, E.V. 2015. Intracellular pH imaging in cancer cells in vitro and tumors in vivo using the new genetically encoded sensor SypHer2. Biochim Biophys Acta 1850:1905-1911. [PubMed: 25964069]
- 49.
- Lenz, J.C., Reusch, H.P., Albrecht, N., Schultz, G., and Schaefer, M. 2002. Ca2+-controlled competitive diacylglycerol binding of protein kinase C isoenzymes in living cells. J Cell Biol 159:291-302. [PMC free article: PMC2173038] [PubMed: 12391024]
- 50.
- Kozak, J.A., Matsushita, M., Nairn, A.C., and Cahalan, M.D. 2005. Charge screening by internal pH and polyvalent cations as a mechanism for activation, inhibition, and rundown of TRPM7/MIC Channels. J Gen Physiol 126:499-514. [PMC free article: PMC2266608] [PubMed: 16260839]
- 51.
- Yamashita, M. and Prakriya, M. 2014. Divergence of Ca2+ selectivity and equilibrium Ca2+ blockade in a Ca2+ release-activated Ca2+ channel. J Gen Physiol 143:325-343. [PMC free article: PMC3933933] [PubMed: 24567508]
- 52.
- Galler, S. and Moser, H. 1986. The ionic mechanism of intracellular pH regulation in crayfish muscle fibres. J Physiol 374:137-151. [PMC free article: PMC1182712] [PubMed: 3091812]
- 53.
- Gomez-Fernandez, C., Pozo-Guisado, E., Ganan-Parra, M., Perianes, M.J., Alvarez, I.S., and Martin-Romero, F.J. 2009. Relocalization of STIM1 in mouse oocytes at fertilization: Early involvement of store-operated calcium entry. Reproduction 138:211-221. [PubMed: 19470709]
- 54.
- Gomez-Fernandez, C., Lopez-Guerrero, A.M., Pozo-Guisado, E., Alvarez, I.S., and Martin-Romero, F.J. 2012. Calcium signaling in mouse oocyte maturation: The roles of STIM1, ORAI1 and SOCE. Mol Hum Reprod 18:194-203. [PubMed: 22053056]
- 55.
- Martin-Romero, F.J., Lopez-Guerrero, A.M., Alvarez, I.S., and Pozo-Guisado, E. 2012. Role of store-operated calcium entry during meiotic progression and fertilization of mammalian oocytes. Int Rev Cell Mol Biol 295:291-328. [PubMed: 22449493]
- 56.
- Lagadic-Gossmann, D., Buckler, K.J., and Vaughan-Jones, R.D. 1992. Role of bicarbonate in pH recovery from intracellular acidosis in the guinea-pig ventricular myocyte. J Physiol 458:361-384. [PMC free article: PMC1175160] [PubMed: 1302269]
- 57.
- Jiang, J., Li, M., and Yue, L. 2005. Potentiation of TRPM7 inward currents by protons. J Gen Physiol 126:137-150. [PMC free article: PMC2266571] [PubMed: 16009728]
- 58.
- Numata, T. and Okada, Y. 2008. Proton conductivity through the human TRPM7 channel and its molecular determinants. J Biol Chem 283:15097-15103. [PMC free article: PMC3258882] [PubMed: 18390554]
- 59.
- Numata, T. and Okada, Y. 2008. Molecular determinants of sensitivity and conductivity of human TRPM7 to Mg2+ and Ca2+. Channels (Austin) 2:283-286. [PubMed: 18719395]
- 60.
- Starkus, J.G., Fleig, A., and Penner, R. 2010. The calcium-permeable non-selective cation channel TRPM2 is modulated by cellular acidification. J Physiol 588:1227-1240. [PMC free article: PMC2872729] [PubMed: 20194125]
- 61.
- Yeh, B.I., Kim, Y.K., Jabbar, W., and Huang, C.L. 2005. Conformational changes of pore helix coupled to gating of TRPV5 by protons. EMBO J 24:3224-3234. [PMC free article: PMC1224685] [PubMed: 16121193]
- 62.
- Yeh, B.-I., Sun, T.-J., Lee, J.Z., Chen, H.-H., and Huang, C.-L. 2003. Mechanism and molecular determinant for regulation of rabbit Transient Receptor Potential Type 5 (TRPV5) channel by extracellular pH. J Biol Chem 278:51044-51052. [PubMed: 14525991]
- 63.
- Chen, X.H., Bezprozvanny, I., and Tsien, R.W. 1996. Molecular basis of proton block of L-type Ca2+ channels. J Gen Physiol 108:363-374. [PMC free article: PMC2229351] [PubMed: 8923262]
- 64.
- Chen, X.-H. and Tsien, R.W. 1997. Aspartate substitutions establish the concerted action of P-region glutamates in repeats I and III in forming the protonation site of L-type Ca2+ Channels. J Biol Chem 272:30002-30008. [PubMed: 9374474]
- 65.
- Jordt, S.E., Tominaga, M., and Julius, D. 2000. Acid potentiation of the capsaicin receptor determined by a key extracellular site. Proc Natl Acad Sci USA 97:8134-8139. [PMC free article: PMC16682] [PubMed: 10859346]
- 66.
- Ryu, S., Liu, B., and Qin, F. 2003. Low pH potentiates both capsaicin binding and channel gating of VR1 receptors. J Gen Physiol 122:45-61. [PMC free article: PMC2234467] [PubMed: 12835470]
- 67.
- Ryu, S., Liu, B., Yao, J., Fu, Q., and Qin, F. 2007. Uncoupling proton activation of vanilloid receptor TRPV1. J Neurosci 27:12797-12807. [PMC free article: PMC6673297] [PubMed: 18032651]
- 68.
- Dhaka, A., Uzzell, V., Dubin, A.E., Mathur, J., Petrus, M., Bandell, M., and Patapoutian, A. 2009. TRPV1 is activated by both acidic and basic pH. J Neurosci 29:153-158. [PMC free article: PMC2729567] [PubMed: 19129393]
- 69.
- Zong, X., Stieber, J., Ludwig, A., Hofmann, F., and Biel, M. 2001. A single histidine residue determines the pH sensitivity of the pacemaker channel HCN2. J Biol Chem 276:6313-6319. [PubMed: 11096117]
- 70.
- Fujita, F., Uchida, K., Moriyama, T., Shima, A., Shibasaki, K., Inada, H., Sokabe, T., and Tominaga, M. 2008. Intracellular alkalization causes pain sensation through activation of TRPA1 in mice. J Clin Invest 118:4049-4057. [PMC free article: PMC2582441] [PubMed: 19033673]
- 71.
- Botana, L.M., Espinosa, J., and Eleno, N. 1987. Adrenergic activity on rat pleural and peritoneal mast cells. Loss of beta-receptors during the purification procedure. Gen Pharmacol 18:141-148. [PubMed: 2436972]
- 72.
- Shore, P.A. 1971. The chemical determination of histamine. Methods Biochem Anal 1971(Suppl):89-97. [PubMed: 4934154]
Albert S. Yu
Department of Cell Biology
University of Connecticut School of Medicine
Farmington, Connecticut
Zhichao Yue
Department of Cell Biology
University of Connecticut School of Medicine
Farmington, Connecticut
Jianlin Feng
Department of Cell Biology
University of Connecticut School of Medicine
Farmington, Connecticut
Lixia Yue
Department of Cell Biology
University of Connecticut School of Medicine
Farmington, Connecticut
- Review Store-Independent Orai Channels Regulated by STIM.[Calcium Entry Channels in Non-...]Review Store-Independent Orai Channels Regulated by STIM.Zhang X, Gueguinou M, Trebak M. Calcium Entry Channels in Non-Excitable Cells. 2018
- Review Studies of Structure-Function and Subunit Composition of Orai/STIM Channel.[Calcium Entry Channels in Non-...]Review Studies of Structure-Function and Subunit Composition of Orai/STIM Channel.Fahrner M, Schindl R, Romanin C. Calcium Entry Channels in Non-Excitable Cells. 2018
- Orai1- and Orai2-, but not Orai3-mediated I(CRAC) is regulated by intracellular pH.[J Physiol. 2022]Orai1- and Orai2-, but not Orai3-mediated I(CRAC) is regulated by intracellular pH.Rychkov GY, Zhou FH, Adams MK, Brierley SM, Ma L, Barritt GJ. J Physiol. 2022 Feb; 600(3):623-643. Epub 2021 Dec 25.
- Review Pharmacology of Store-Operated Calcium Entry Channels.[Calcium Entry Channels in Non-...]Review Pharmacology of Store-Operated Calcium Entry Channels.Bird GS, Putney JW Jr. Calcium Entry Channels in Non-Excitable Cells. 2018
- Review Regulation and Role of Store-Operated Ca(2+) Entry in Cellular Proliferation.[Calcium Entry Channels in Non-...]Review Regulation and Role of Store-Operated Ca(2+) Entry in Cellular Proliferation.Hodeify R, Yu F, Courjaret R, Nader N, Dib M, Sun L, Adap E, Hubrack S, Machaca K. Calcium Entry Channels in Non-Excitable Cells. 2018
- Regulation of Orai/STIM Channels by pH - Calcium Entry Channels in Non-Excitable...Regulation of Orai/STIM Channels by pH - Calcium Entry Channels in Non-Excitable Cells
Your browsing activity is empty.
Activity recording is turned off.
See more...