The content of this book is licensed under a Creative Commons Attribution-NonCommercial-NoDerivs 4.0 Unported license. To view the terms and conditions of this license, visit https://creativecommons.org/licenses/by-nc-nd/4.0/
NCBI Bookshelf. A service of the National Library of Medicine, National Institutes of Health.
Varki A, Cummings RD, Esko JD, et al., editors. Essentials of Glycobiology [Internet]. 4th edition. Cold Spring Harbor (NY): Cold Spring Harbor Laboratory Press; 2022. doi: 10.1101/glycobiology.4e.25
This chapter focuses on the nematode (roundworm) Caenorhabditis elegans as an example of the phylum Nematoda. C. elegans provides a powerful genetic system for studying glycans during embryological development and in primitive organ systems.
DEVELOPMENTAL BIOLOGY OF C. ELEGANS
C. elegans is transparent, and individual cells can be easily visualized in the living organism through all stages of development. Basically, the worm is a tube within a tube (Figure 25.1). A cuticle composed of a collagenous, multilayered, protective exoskeleton surrounds the worm. The “mouth” at the anterior end connects to a tubular intestinal system, which is composed of a muscular pharynx and an intestine. The gonad occupies most of the body cavity. In the hermaphrodite, the gonad is bilobed, with each lobe connecting via an oviduct and spermatheca to a shared midventral vulva and uterus. The worm exists as two sexes, hermaphrodite or male. Eggs pass through the spermatheca where fertilization takes place by stored sperm, and the eggs begin to develop inside the uterus. During sexual reproduction, males fertilize hermaphrodites. The male sperm is also stored in the spermatheca and is preferentially used during fertilization.
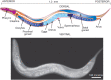
FIGURE 25.1.
Caenorhabditis elegans. A composite diagram (upper panel) and photograph (lower panel) of the adult hermaphrodite with labeled body parts. (Photograph kindly provided by Dr. Ian D. Chin-Sang at Queen's University, Kingston, Ontario.) For additional details (more...)
Gastrulation starts before egg laying; at this stage, the embryo contains about 30 cells (Figure 25.2). Proliferation results in an embryo of 558 relatively undifferentiated cells. Then organogenesis/morphogenesis begins, terminal differentiation occurs, and the embryo hatches. The animal normally passes through four larval stages, termed L1, L2, L3, and L4 (Figure 25.2). The end of each larval stage is marked by molting, when the cuticle is shed. In L1 larvae, the nervous system, the reproductive system, and the digestive tract begin to develop, and this is completed by the L4 stage. Mature adults hatch after ∼45–50 h, whereby hermaphrodites contain 959 somatic cells, including 302 neurons, and 95 body-wall muscle cells. At this time, the hermaphrodite can lay its first eggs, thus completing the 3.5-d life cycle; production of oocytes continues for ∼4 d, resulting in about 300 progeny. Afterwards, the animal lives for another 10–15 d. Overcrowding or starvation leads to formation of dauer larvae, a dormant stage, which is easily distinguished from other developmental stages by morphology and behavior.

FIGURE 25.2.
Life cycle of Caenorhabditis elegans. For additional details on the biology of C. elegans, see the WormAtlas.
GLYCANS IN C. ELEGANS
Considering the large number of glycomic studies on C. elegans since 2001, it is probably not an exaggeration to state that this anatomically simple worm has one of the most varied and unusual glycomes of any invertebrate organism studied to date. Although there are a number of conserved elements in its glycans, there are many notable differences between the types of glycans made by C. elegans and those in “higher” animals. There are, for example, no sialic acids or other anionic moieties on its N-glycans, but a wide range of fucose modifications on truncated “paucimannosidic” glycans have been found. Moreover, worm O-glycans and glycolipids, which also lack sialic acids, have different core structures. Although some predictions regarding the types of oligosaccharides in the worm can be made from the range of glycosylation-relevant genes in its genome, glycan analyses continue to surprise as to the glycomic potential of this organism.
As in most eukaryotes, the N-glycans of C. elegans are derived from a 14-sugar dolichol-linked precursor Glc3Man9GlcNAc2, which is transferred to asparagine residues within the -Asn-X-Ser/Thr- sequons of nascent polypeptides in the endoplasmic reticulum (ER) (Chapter 9). Subsequent trimming is akin to that in plants and other animals, but the GlcNAc1Man5GlcNAc2-Asn generated in the Golgi is then further processed in a unique way (Figure 25.3). On one hand, C. elegans can generate various complex structures with up to three antennae with either single N-acetylglucosamine residues or HexNAc2-3 motifs, some of which are modified with phosphorylcholine as in many other nematode glycoproteins. On the other hand, a high degree of modification of the core region is also a feature of this organism. The action of various Golgi β-hexosaminidases and α-mannosidase results in truncated so-called “paucimannosidic” structures; in addition to core α1,6-fucosylation as in “higher” animals, two types of α1,3-fucose substitution of the core chitobiose are known, all of which can be galactosylated. Particularly curious is the addition of a bisecting galactose, which can also be α1,2-fucosylated, to the core β-mannose in a significant population of the N-glycans (Figure 25.3); maximally, five fucose residues as well as multiple galactose and methyl substitutions of the core region are possible.
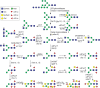
FIGURE 25.3.
Biosynthesis of paucimannosidic and core fucosylated N-glycans in Caenorhabditis elegans. Solid and dashed lines refer, respectively, to proven and proposed biosynthetic steps.
Many forms of O-glycans familiar from mammals and insects are either structurally defined or genetically predicted in C. elegans. These include “mucin-type” O-glycans, many of which are based on the core 1 O-glycan structure common to vertebrates (Chapter 10), but which can be extended by β-glucose, glucuronic acid, and α1-2 fucose residues (Figure 25.4). In terms of glycosaminoglycans (GAGs), chondroitin and heparan sulfate (CS and HS) have been detected in C. elegans, but neither keratan sulfate (KS), dermatan sulfate (DS), nor hyaluronan (Chapter 16); whereas the CS chains contain a low amount of sulfate, the overall structure of HS in C. elegans is similar to the chains elaborated by vertebrates (see Figure 25.5). Enzymatic and genomic data indicate that there are various domain-specific forms of glycosylation in C. elegans, including O-fucose (Fucα1-Ser/Thr) on epidermal growth factor (EGF)-like domains and thrombospondin type-1 repeats (TSRs), in the context of precise consensus sequences (Chapter 13), C-mannosylation of TSRs, and TMTC-dependent O-mannosylation of cadherins. However, POMT-mediated O-mannosylation of dystroglycan is absent. Cytoplasmic and nuclear proteins in C. elegans can be modified with O-GlcNAc as in other animals.

FIGURE 25.4.
Biosynthesis of core-1 O-glycans in Caenorhabditis elegans (A) and some O-glycans proposed to occur in adult worms (B).
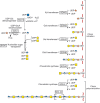
FIGURE 25.5.
Biosynthesis of chondroitin in Caenorhabditis elegans. Mutations in individual steps were identified as squashed vulva mutants, as described in the text.
Glycosphingolipids (GSLs) (Chapter 11) in C. elegans have a core consisting of GlcNAcβ1-3Manβ1-4Glcβ1-Cer, which is based, as in insects, on the arthro-series Manβ1-4Glcβ1-Cer core rather than the common Galβ1-4Glcβ1-Cer core found in vertebrates; some of the glycolipids carry fucose or phosphorylcholine residues (see Figure 25.7). In addition, C. elegans has genes encoding the enzymes for synthesis of glycosylphosphatidylinositol (GPI)-anchored glycoproteins, but the GPI anchor structures are not yet defined (Chapter 12).
GLYCOSYLTRANSFERASE GENES IN C. ELEGANS
The C. elegans genome encodes homologs of many of the enzymes for glycoconjugate biosynthesis that are found in “higher” animals and humans, including enzymes for the synthesis of O-GalNAc (mucin-type) glycans, GAGs, O-GlcNAc, N-glycans, GSLs, and GPI anchors. In contrast to vertebrates (Chapter 15), the worm lacks sialic acids and any enzymes associated with sialic acid biosynthesis or utilization. Additionally, on the basis of the glycoconjugate structures, C. elegans is predicted to express a wide assortment of enzymes involved in their metabolism. Indeed, the genome of C. elegans appears to encode about 300 carbohydrate-active enzymes (CAZy database; http://www.cazy.org/), including glycosyltransferases, glycosidases, epimerases, polysaccharide lyases, and carbohydrate esterases (Chapter 52). To date, most of these putative enzymes are not characterized, and very little is known overall about their expression or function in C. elegans. Some information has arisen from phenotypes generated by mutagenesis, as discussed below. Together, these studies have identified some interesting differences and similarities between C. elegans and vertebrates.
The C. elegans genome is predicted to encode some 240 glycosyltransferases and some GT families are highly represented, including 11 putative ppGalNAcTs, 32 α-fucosyltransferase homologs (five α1-3FucTs, 26 α1-2FucTs, and one α1-6FucT), and more than 20 β-N-acetylglucosaminyltransferase homologs. Relatively few glycan-modifying enzymes from C. elegans have been shown to be functional; in only a few cases has their acceptor specificity been characterized. Indeed, there are still many gaps in our knowledge as glycosyltransferases required for many glycosidic bonds are still to be identified or there are glycosyltransferase homologs for which no corresponding activity is known.
In terms of Golgi modifications of N-glycans, C. elegans contrasts with humans and most other vertebrates in that it has three genes (gly-12, gly-13, and gly-14), rather than just one, encoding the β1-2 N-acetylglucosaminyltransferase (GlcNAcT-1) that catalyzes formation of GlcNAcβ1-2Man5GlcNAc2-Asn (see Figure 25.3). Triple-knockout worms defective in gly-12, gly-13, and gly-14 generate very low amounts of paucimannosidic Man2-3GlcNAc2 N-glycans (with and without core α1-6-fucose), but Man5GlcNAc2-Asn is the major structure. On the other hand, core α1-3-fucosylation is not abolished in this mutant.
The GlcNAcβ1-2Man5GlcNAc2-Asn biosynthetic intermediate is then acted on by a single α-mannosidase II to generate GlcNAcβ1-2Man3GlcNAc2-Asn. C. elegans contains three α-mannosidase activities: the lysosomal enzyme AMAN-1, the α-mannosidase II/IIx-like AMAN-2 involved in N-glycan processing, and the Co++-dependent AMAN-3. A mutant harboring a large deletion in the aman-2 (F58H1.1) gene generates largely Man5GlcNAc2-Asn and GlcNAc1Man5GlcNAc2-Asn-R as well as fucosylated and phosphorylcholine-modified variants thereof, but lacks the paucimannosidic structures. In addition, the mutant has reduced levels of the core α1-3 fucose antigen associated with reactivity to antibodies to horseradish peroxidase.
As in many invertebrates, the antennal GlcNAc is normally absent from the final glycan products and indeed C. elegans has two relevant β-hexosaminidases (HEX-2 and HEX-3), which cleave GlcNAcβ1-2Man3GlcNAc2-Asn to generate the paucimannose structure Man3GlcNAc2-Asn, a reaction not found in vertebrates. Furthermore, there is no evidence for galactosylation of antennal GlcNAc and the closest C. elegans homolog to the human β1-4 galactosyltransferase is actually a β1-4 N-acetylgalactosaminyltransferase generating the LacdiNAc sequence (GalNAcβ1-4GlcNAc-R) on N-glycan and glycolipid antennae. Three core fucosyltransferases have been characterized and the glycomes of the corresponding mutants analyzed. Although the fut-8 gene encodes an enzyme with the same specificity as the mammalian FUT8 α1-6 fucosyltransferases, two α1-3 fucosyltransferases (FUT-1 and FUT-6) with unusual substrate preferences transfer fucose to the proximal and distal core GlcNAc residues; whereas FUT-1, unlike plant and insect core α1-3 fucosyltransferases, cannot transfer to glycans with a β1-2GlcNAc on the α1-3-mannose, the action of FUT-6 is blocked by the presence of α1-6-mannose. Activity of recombinant forms of two α1-2 fucosyltransferases and a fucose-modifying β1-4-galactosyltransferase (GALT-1) have also been reported.
In terms of peptide-modifying glycosyltransferases, 11 of the nematode UDP-GalNAc polypeptide α-N-acetylgalactosaminyltransferases (ppGalNAcTs) that modify Ser/Thr residues of mucin core polypeptides have been prepared as recombinant proteins, but only five of them are active toward mammalian peptide acceptors. Additionally, the C. elegans β1-3 galactosyltransferase (T-synthase), which generates the core 1 O-glycan structure common to vertebrates (Chapter 10), has been characterized. The single C. elegans cytosolic or nuclear O-GlcNAc transferase (OGT) and the GAG-initiating O-xylosyltransferase homologs as well as its POFUT1 and 2 O-fucosyltransferases have been characterized at the biochemical and genetic levels. C. elegans can also synthesize the canonical core tetrasaccharide of GAGs, GlcAβ1-3Galβ1-3Galβ1-4Xylβ1-Ser, and can extend this to generate CS and HS. In the case of HS biosynthesis, there are numerous sulfotransferases and an HS epimerase, but fewer chondroitin-modifying enzymes, in C. elegans. The genes required for initiation of the unusual arthroseries glycolipid core structure (Manβ1-4Glcβ1-Cer) are also known, but C. elegans lacks the β1-4 galactosyltransferase that would synthesize the more familiar Galβ1-4Glcβ1-Cer glycolipid core.
Expression of the known glycosyltransferases in C. elegans has not been systematically mapped at the cellular level during development or in the adult organism. Many of the relevant studies are based on promoter analyses using GFP as a reporter. Therefore, the promoter region for a gene of interest (usually 0.5–1.5 kb upstream of the gene and sometimes including the upstream elements and the first few exons of the gene) is ligated to the cDNA encoding GFP. Transgenic animals are then produced by direct injection of DNA into the hermaphrodite gonad. Newly developing animals take up this DNA and become transgenic. Thus, one can observe promoter utilization in the different stages of development (see Figure 25.2). Using this approach, it has been found that some glycosyltransferase genes are widely expressed, including the T-synthase, which generates core 1 O-glycans (Figure 25.4), the SQV-2 involved in GAG biosynthesis (Figure 25.5), and the two protein O-fucosyltransferases POFUT1 and POFUT2. In contrast, expression of the six individual glycosyltransferases related to core-2 N-acetylglucosaminyltransferases in vertebrates (see Chapter 10) is tissue-specific; one such gene, gly-15, is expressed only in two gland cells. Relevant to N-glycan biosynthesis, promoter analyses show that gly-12 and gly-13 are expressed in all cells beginning in embryogenesis, whereas gly-14 is expressed only in intestinal cells from L1 to adults; the aman-2 gene is expressed in most cells of the organism. Among the 26 α1-2FucTs that are predicted in C. elegans, one of them (CE2FT-1; FUT-2) is expressed in a single cell in embryos and exclusively in 20 intestinal cells of larval stages L1–L4 and adult worms. Thus, in large gene families, individual members may be expressed in a localized fashion and have unique activities toward certain substrates, whereas single gene families appear to be expressed in all cells.
FUNCTIONAL ANALYSIS OF GLYCOCONJUGATES
Different methods exist to genetically manipulate C. elegans and many of these approaches have yielded important information about the functions of glycosyltransferases, their glycan products, and lectin-binding proteins. Several dozen genes involved in glycosylation pathways have been shown to be developmentally important in C. elegans or important in resistance or susceptibility to pathogens in the innate immunity of the worm.
N-Glycans and O-Glycans on Glycoproteins
Glycoproteins in C. elegans have both N- and O-glycans, as discussed above. In vertebrates, interference of the early steps in N-glycosylation or O-glycosylation causes embryonic lethality or results in severe developmental phenotypes (Chapter 45). As in vertebrates, interference of the later steps in N- and O-glycan biosynthesis in C. elegans does not cause developmental problems, but the effects on the glycome can be very profound. For instance, ablation in C. elegans of the three genes (gly-12, gly-13, and gly-14) that encode the three GlcNAcT-I isoforms or of the single mannosidase II gene (aman-2) results in drastically altered N-glycomes without affecting development under laboratory conditions, which is in contrast to the effects in mammals; however, altered susceptibility to bacterial infection has been reported for GlcNAcT-I mutant worms. Also, deletion of the OGT transferase required for the modification of cytoplasmic and nuclear O-GlcNAc proteins, although fatal to vertebrate cells (Chapter 19), is not lethal to C. elegans, but is accompanied by a phenotype that resembles human insulin resistance. A related phenotype is also produced by deletion of the “antagonistic” OGA O-GlcNAcase.
In terms of O-glycans, the redundancy of ppGalNAcT and α1,2 and α1,3 fucosyltransferase homologues may be a reason for a lack of developmental phenotypes associated with the loss of any members of these families. However, RNAi studies on the worm's POFUT2 (encoded by the pad-2 gene) suggest that O-fucose–modifications of TSRs (Chapter 13) are required for normal morphogenesis. The biosynthesis of all fucosylated ligands requires the precursor GDP-fucose and its transport into the Golgi apparatus by nucleotide sugar transporters (Chapter 5). One human disease, leukocyte-deficiency type II (LAD II), is caused by a defect in the transport of GDP-fucose (Chapter 34). A screen of candidate C. elegans nucleotide sugar transporter genes led to the identification of one which indeed complemented the transport and fucosylation defect in LAD II fibroblasts and thereby to pinpointing of the genetic defect in these patients. A defect in GDP-fucose biosynthesis caused by a mutation in the bre-1 gene results in resistance to some fungal toxic lectins; furthermore, mutations in genes encoding the FUT-1, -6, and -8 core fucosyltransferases, the GALT-1 galactosyltransferase, the HEX-2 and HEX-3 hexosaminidases, or the SAMT-1 transporter (the latter required for glycan methylation) are associated with altered susceptibility to proteins expressed by nematoxic fungi.
C. elegans is also an interesting model system to study infection and innate immunity. The organism may be colonized by different bacterial pathogens, including Pseudomonas aeruginosa, Yersinia pestis, and Yersinia pseudotuberculosis. The two Yersinia species generate a sticky biofilm (an exopolysaccharide matrix encasing a community of bacteria) on the exterior of the worm's head that impairs viability. P. aeruginosa, in contrast, colonizes the intestinal tissues. Another bacterium, Microbacterium nematophilum, sticks to the anus of the animals and induces an irritation in the underlying hypodermal tissue. Bacillus thuringiensis (Bt) infection leads to destruction of the intestine, which is discussed in more detail below in regard to glycolipids. Mutations in the worm, some of them affecting glycosylation processes, have been found that affect colonization by these bacteria. Examples of such mutants include those with defects in bus genes encoding putative glycosyltransferases.
An especially interesting set of mutations are the srf mutants (altered surface antigenicity mutants). Some of the srf mutants were identified by altered antibody or lectin binding to the cuticle, indicating that loss of cuticle components exposed new antigens. srf-3 mutants are resistant to infection by M. nematophilum and lack some O-linked glycoconjugates containing glucuronic acid and galactose as well as reduced levels of some N-glycans. srf-3 encodes a nucleotide sugar transporter that can transport both UDP-galactose and UDP-N-acetylglucosamine, suggesting that altered sugar composition of the cuticle resulting from mutations in this transporter confer resistance to M. nematophilum. Interestingly, there are 18 putative nucleotide sugar transporters (Chapter 5) in the genome of C. elegans, which is a considerably larger number than the known nucleotide sugars (UDP-galactose, UDP-glucose, UDP-N-acetylglucosamine, UDP-N-acetylgalactosamine, UDP-xylose, GDP-mannose, and GDP-fucose), suggesting possible functional overlap in these transporters.
Proteoglycans and Glycosaminoglycans
During egg laying, fertilized eggs must pass through the vulva, which is a simple tubular structure that links the gonads with the external cuticle. During postembryonic development, vulva morphogenesis arises through the invagination of a single layer of epithelial cells. Using mutagenesis, several mutations that perturb invagination of the vulva were identified (designated sqv or squashed vulva). In the original screen, 25 mutations were identified in eight genes named sqv-1 through sqv-8. All of the mutations produced a similar phenotype: that is, partial collapse of vulval invagination, elongation of the central vulval cells, hermaphrodite sterility associated with maternal-effect lethality, and cytokinesis defects in the early embryo. All eight sqv genes show homology with vertebrate enzymes that are involved in the biosynthesis of GAGs (Figure 25.5). sqv-1, sqv-4, and sqv-7 encode proteins that have roles in nucleotide sugar metabolism and transport. The SQV-7 nucleotide transporter was the first example of a carrier that could import more than one nucleotide sugar into the Golgi (Chapter 5). SQV-4 and SQV-1 proteins represent sequential enzymes involved in the formation of UDP-glucuronic acid and UDP-xylose, respectively, showing that the sqv mutations most likely affect GAG synthesis. Biochemical analysis of sqv-6, sqv-3, sqv-2, and sqv-8 showed that they encode worm orthologs of the vertebrate transferases required for the assembly of the linkage region tetrasaccharide common to HS and chondroitin. Finally, characterization of sqv-5 showed that it encodes the chondroitin synthase, which acts in concert with the chondroitin polymerizing factor encoded by the mig-22 gene. Thus, the various phenotypes (failed invagination of the epithelial layer that forms the vulva, maternal-effect lethality, and cytokinesis defects) result from defective chondroitin formation.
The requirement for chondroitin assembly in seemingly disparate systems may result from biophysical changes in the lumen of the vulva or between the eggshell and the embryo. One idea is that the high negative charge imparted by the glucuronic acids in chondroitin attracts counterions that raise the local osmolarity, causing a swelling pressure. Another possibility is that the chondroitin acts as a physical scaffold bound to the cell membrane or eggshell. Interestingly, the sqv screen did not detect mutations affecting genes that encode proteoglycan core proteins on which the chondroitin chains assemble, but proteomic analysis has led to the identification of nine novel chondroitin sulfate proteoglycan (CPG) core proteins modified with chondroitin chains (Figure 25.6). Two of these (CPG-1 and CPG-2) contain chitin-binding domains that presumably allow the proteoglycans to interact with chitin in the eggshell, thus positioning the proteoglycans between the eggshell and the plasma membrane of the embryo, in which they could serve as spacers or osmotic regulators. Silencing cpg-1 and cpg-2 expression by RNAi recapitulates the cytokinesis defect observed in sqv mutants, suggesting that these are the relevant proteoglycans. The proteoglycans involved in epithelial invagination have not yet been determined.
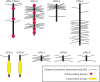
FIGURE 25.6.
Chondroitin proteoglycans (CPGs) of Caenorhabditis elegans.
HS biosynthesis in C. elegans follows the same pattern observed in vertebrate systems (Chapter 17). Mutations in the pathway for HS biosynthesis are lethal in C. elegans. Two of the key genes involved in this pathway are rib-1 and rib-2, homologs of the vertebrate genes Ext2 and Ext1, respectively, which catalyze the polymerization of the backbone of HS chains (GlcAβ1-4GlcNAcα1-4) (Chapter 17). Mutants in rib-2, the worm homolog of Ext1, have defects in development and egg laying. The worm genome also contains a single gene for glucuronic acid C-5 epimerase (hse-5) and five genes for sulfotransferase activities (GlcNAc N-deacetylase/N-sulfotransferase [Ndst], hst-1; uronyl 2-O-sulfotransferase, hst-2; 3-O-sulfotransferases, hst-3.1 and hst-3.2; and 6-O-sulfotransferase, hst-6), all of which are homologs of vertebrate genes involved in HS synthesis. In contrast, vertebrates contain four Ndsts, three 6-O-sulfotransferases, and seven 3-O-sulfotransferases. Although mutations in the epimerase (hse-5) and the sulfotransferases (hst-6, hst-2) do not affect viability, they cause defects in specific cell migration, axonal outgrowth, and/or neurite branching. Consistent with this finding, inactivation of the cell surface HS proteoglycan syndecan (sdn-1) affects neural migration and axonal guidance. C. elegans also produces two GPI-anchored HS proteoglycans. LON-2, a member of the glypican family, negatively regulates a bone morphogenetic protein-like signaling pathway that controls body length in C. elegans. Worms also contain a homolog of the vertebrate basement membrane proteoglycan perlecan (encoded by unc-52). At least three major classes of UNC-52 isoforms are produced through alternative splicing, and distinct spatial and temporal expression patterns occur throughout development. In keeping with the “uncoordinated” phenotype, unc-52 mutants affect myofilament assembly in body-wall muscle during embryonic development. Thus, as in vertebrates, HS proteoglycans mediate many fundamental processes during development and in the adult animal.
Glycolipids
The arthro-series glycolipids in C. elegans include structures modified with either phosphorylcholine or fucose residues (Figure 25.7A). Recent studies on the resistance of C. elegans to bacterial toxins (Chapter 37) have led to interesting insights into the structures and functions of glycolipids. Bt crystal toxins are used in both transgenic and organic farming because of their ability to kill insect pests. Bt is toxic to C. elegans, but following mutagenesis, strains resistant to one crystal toxin (Cry5B) were identified and classified as bre-1 through bre-5—which encode either an enzyme required for GDP-fucose biosynthesis or glycolipid-specific glycosyltransferases. None of the strains showed altered development, but they were highly resistant to Bt, whereas the Cry5B-resistant mutants had truncated glycolipids lacking terminal fucose. Therefore, the Cry5B ligands in the intestinal epithelium are concluded to be the largest C. elegans glycolipids (see also Figure 25.7 for the relevant enzymatic steps defective in bre mutants).
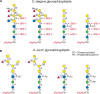
FIGURE 25.7.
Examples of nematode glycolipids. (A) Structures of glycolipids from Caenorhabditis elegans. Mutations that result in truncated glycolipids have been identified as bre mutants, as described in the text; the steps absent in these mutants are catalyzed (more...)
GLYCAN-BINDING PROTEINS IN C. ELEGANS
Although the C. elegans genome encodes a number of predicted glycan-binding proteins (GBPs), only a few of them have been characterized biochemically or explored by genetic manipulation. The first GBP found in C. elegans was a galectin (Chapter 36), which was isolated by affinity-chromatography and sequenced in 1992. This was surprising because until this observation, galectins were thought to be expressed only in vertebrates. Amazingly, the C. elegans genome encodes 28 putative galectins, nearly twice as many as in humans. Only two of these proteins have been studied in detail: a tandem-repeat 32-kDa galectin (LEC-6) and a prototypical 16-kDa galectin (LEC-1). Both galectins can bind to galactose-containing ligands.
Some 283 clec genes have been identified in the C. elegans genome that encode proteins with C-type lectin domains (CTLDs) (Chapter 34), whereby some proteins have multiple CTLDs. Only 19 of these CTLDs have the sequence hallmarks predicting carbohydrate recognition. In contrast to the CTLD-containing proteins in vertebrates, most of the proteins with CTLDs in C. elegans have signal sequences and no transmembrane domains, indicating that they are secreted proteins, but their functional roles have not yet been studied in detail in this organism. However, as expression of several of these CTLDs is up-regulated on challenge of the animal with nematocidal Bt strains and other pathogenic bacteria; these lectins may well have roles in the innate immune system of C. elegans.
GLYCOBIOLOGY OF OTHER NEMATODES
Studies in the nonparasitic nematode C. elegans have been incredibly rewarding because of the ease of genetic manipulation and culture. Much less is known about parasitic nematodes, which cause tremendous death and suffering in animals and people throughout the world. It might be expected that C. elegans and other nematodes share much in common in terms of glycoconjugate structures and biosynthesis, but each nematode has differences in glycans compared with C. elegans. These may pertain to their virulence and parasitic requirements.
Some of the major parasitic nematodes that have been studied in terms of glycoconjugates include Acanthocheilonema viteae, Ascaris suum, Dictyocaulus viviparous, Dirofilaria immitis, Haemonchus contortus, Oesphagostomum dentatum, Onchocerca volvulus, Trichuris suis, Trichinella spiralis, Toxocara canis, and Toxocara cati (Chapter 43). A. suum is a parasitic intestinal nematode of pigs. Like C. elegans, the A. suum N-glycans are paucimannose-rich and contain phosphorylcholine and core fucose residues. In contrast, the N-glycans of the parasitic nematode of deer Parelaphostrongylus tenuis are extensively terminally modified with galactose and carry the terminal structure Galα1-3Galβ1-4GlcNAc-R. The cattle parasite D. viviparous has N-glycans with Lewis antigens (see Chapter 14), including Lewis x. The sheep parasite H. contortus synthesizes N-glycans containing the fucosylated LacdiNAc antigen GalNAcβ1-4(Fucα1-3)GlcNAc-R (Chapter 43), whereas the dog heartworm D. immitis has N-glycans with extending chito-motifs and/or with terminal glucuronic acid. Most nematode N-glycomes reported to date contain phosphorylcholine-modified structures. There are few data on O-glycans of nematode parasites other than short O-glycans in Toxocara or phosphorylcholine-modified GAG-like structures in O. dentatum (Figure 25.8).

FIGURE 25.8.
Examples of nematode glycans. Caenorhabditis core modified N-glycan; Oesophagostomum and Haemonchus N-glycans; Trichuris N-glycan with fucose/PC-modified LacdiNAc; PC-modified glycosaminoglycans from Oesophagostomum; Toxocara O-glycan; Dictyocaulus Lewis-x-modified (more...)
Nematodes also make unusual glycolipids, and it is likely that each type of nematode synthesizes different glycolipid structures. One of the best-studied nematodes in terms of glycolipids is A. suum. Many of its glycolipids, which also contain the arthroseries core, have galactose and fucose modifications, in addition to phosphorylcholine and phosphorylethanolamine (Figure 25.7B). Virtually nothing is known about the genetics regulating glycosylation in these parasitic nematodes, as most studies have focused on the analysis of glycan structures; on the other hand, the application of glycan microarray technology is starting to reveal ligands for proteins of mammalian innate immune systems.
ACKNOWLEDGMENTS
The authors acknowledge contributions to the previous version of this chapter from Jeffrey D. Esko and helpful comments and suggestions from Todd Lowary and Hiroshi Nakato.
FURTHER READING
- Brenner S. 1974. The genetics of Caenorhabditis elegans. Genetics 77: 71–94. doi:10.1093/genetics/77.1.71 [PMC free article: PMC1213120] [PubMed: 4366476] [CrossRef]
- Drickamer K, Dodd RB. 1999. C-Type lectin-like domains in Caenorhabditis elegans: predictions from the complete genome sequence. Glycobiology 9: 1357–1369. doi:10.1093/glycob/9.12.1357 [PubMed: 10561461] [CrossRef]
- Oriol R, Mollicone R, Cailleau A, Balanzino L, Breton C. 1999. Divergent evolution of fucosyl-transferase genes from vertebrates, invertebrates, and bacteria. Glycobiology 9: 323–334. doi:10.1093/glycob/9.4.323 [PubMed: 10089206] [CrossRef]
- Dodd RB, Drickamer K. 2001. Lectin-like proteins in model organisms: implications for evolution of carbohydrate-binding activity. Glycobiology 11: 71R–79R. doi:10.1093/glycob/11.5.71r [PubMed: 11425795] [CrossRef]
- Hirabayashi J, Arata Y, Kasai K. 2001. Glycome project: concept, strategy and preliminary application to Caenorhabditis elegans. Proteomics 1: 295–303. doi:10.1002/1615-9861(200102)1:2<295::aid-prot295>3.0.co;2-c [PubMed: 11680876] [CrossRef]
- Schachter H. 2004. Protein glycosylation lessons from Caenorhabditis elegans. Curr Opin Struct Biol 14: 607–616. doi:10.1016/j.sbi.2004.09.005 [PubMed: 15465323] [CrossRef]
- Olson SK, Bishop JR, Yates JR, Oegema K, Esko JD. 2006. Identification of novel chondroitin proteoglycans in C. elegans: embryonic cell division depends on CPG-1 and CPG-2. J Cell Biol 173: 985–994. doi:10.1083/jcb.200603003 [PMC free article: PMC2063922] [PubMed: 16785326] [CrossRef]
- Shi H, Tan J, Schachter H. 2006. N-glycans are involved in the response of Caenorhabditis elegans to bacterial pathogens. Methods Enzymol 417: 359–389. doi:10.1016/s0076-6879(06)17022-6 [PubMed: 17132514] [CrossRef]
- Antoshechkin I, Sternberg PW. 2007. The versatile worm: genetic and genomic resources for Caenorhabditis elegans research. Nat Rev Genet 8: 518–532. doi:10.1038/nrg2105 [PubMed: 17549065] [CrossRef]
- Barrows BD, Haslam SM, Bischof LJ, Morris HR, Dell A, Aroian RV. 2007. Resistance to Bacillus thuringiensis toxin in Caenorhabditis elegans from loss of fucose. J Biol Chem 282: 3302–3311. doi:10.1074/jbc.m606621200 [PubMed: 17135259] [CrossRef]
- Laughlin ST, Bertozzi CR. 2009. In vivo imaging of Caenorhabditis elegans glycans. ACS Chem Biol 4: 1068–1072. doi:10.1021/cb900254y [PMC free article: PMC2807738] [PubMed: 19954190] [CrossRef]
- Gravato-Nobre MJ, Stroud D, O'Rourke D, Darby C, Hodgkin J. 2011. Glycosylation genes expressed in seam cells determine complex surface properties and bacterial adhesion to the cuticle of Caenorhabditis elegans. Genetics 187: 141–155. doi:10.1534/genetics.110.122002 [PMC free article: PMC3018313] [PubMed: 20980242] [CrossRef]
- Wohlschlager T, Butschi A, Grassi P, Sutov G, Gauss R, Hauck D, Schmieder SS, Knobel M, Titz A, Dell A, et al. 2014. Methylated glycans as conserved targets of animal and fungal innate defense. Proc Natl Acad Sci 111: E2787–E2796. doi:10.1073/pnas.1401176111 [PMC free article: PMC4103367] [PubMed: 24879441] [CrossRef]
- Yan S, Brecker L, Jin C, Titz A, Dragosits M, Karlsson NG, Jantsch V, Wilson IB, Paschinger K. 2015. Bisecting galactose as a feature of N-glycans of wild-type and mutant Caenorhabditis elegans. Mol Cell Proteomics 14: 2111–2125. doi:10.1074/mcp.m115.049817 [PMC free article: PMC4523199] [PubMed: 26002521] [CrossRef]
- Jiménez-Castells C, Vanbeselaere J, Kohlhuber S, Ruttkowski B, Joachim A, Paschinger K. 2017. Gender and developmental specific N-glycomes of the porcine parasite Oesophagostomum dentatum. Biochim Biophys Acta 1861: 418–430. doi:10.1016/j.bbagen.2016.10.011 [PMC free article: PMC5201199] [PubMed: 27751954] [CrossRef]
- Martini F, Eckmair B, Štefanić S, Jin C, Garg M, Yan S, Jiménez-Castells C, Hykollari A, Neupert C, Venco L, et al. 2019. Highly modified and immunoactive N-glycans of the canine heartworm. Nat Commun. 10: 75. doi:10.1038/s41467-018-07948-7 [PMC free article: PMC6325117] [PubMed: 30622255] [CrossRef]
- Review Nematoda.[Essentials of Glycobiology. 2015]Review Nematoda.Wilson IBH, Cummings RD, Aebi M. Essentials of Glycobiology. 2015
- Review Nematoda.[Essentials of Glycobiology. 2009]Review Nematoda.Cummings RD, Esko JD. Essentials of Glycobiology. 2009
- Review The use of Caenorhabditis elegans in parasitic nematode research.[Parasitology. 2004]Review The use of Caenorhabditis elegans in parasitic nematode research.Gilleard JS. Parasitology. 2004; 128 Suppl 1:S49-70.
- Review Caenorhabditis elegans in anthelmintic research - Old model, new perspectives.[Int J Parasitol Drugs Drug Res...]Review Caenorhabditis elegans in anthelmintic research - Old model, new perspectives.Hahnel SR, Dilks CM, Heisler I, Andersen EC, Kulke D. Int J Parasitol Drugs Drug Resist. 2020 Dec; 14:237-248. Epub 2020 Oct 2.
- On the extent and origins of genic novelty in the phylum Nematoda.[PLoS Negl Trop Dis. 2008]On the extent and origins of genic novelty in the phylum Nematoda.Wasmuth J, Schmid R, Hedley A, Blaxter M. PLoS Negl Trop Dis. 2008 Jul 2; 2(7):e258. Epub 2008 Jul 2.
- Nematoda - Essentials of GlycobiologyNematoda - Essentials of Glycobiology
- Taxonomy Links for SRA (Select 283736) (1)Taxonomy
- TSA: Chloropicon maureeniae, TRINITY-DN14291-c0-g1-i1, transcribed RNA sequenceTSA: Chloropicon maureeniae, TRINITY-DN14291-c0-g1-i1, transcribed RNA sequencegi|2001906067|emb|HBQS01001926.1|Nucleotide
Your browsing activity is empty.
Activity recording is turned off.
See more...