NCBI Bookshelf. A service of the National Library of Medicine, National Institutes of Health.
Varki A, Cummings RD, Esko JD, et al., editors. Essentials of Glycobiology. 2nd edition. Cold Spring Harbor (NY): Cold Spring Harbor Laboratory Press; 2009.
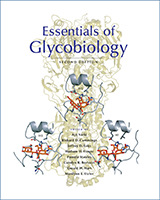
Essentials of Glycobiology. 2nd edition.
Show detailsC-type lectins are CA++-dependent glycan-binding proteins that share primary and secondary structural homology in their carbohydrate-recognition domains (CRDs). These proteins have a C-type lectin fold, which is a fold with highly variable protein sequence that is also present in many proteins that do not bind carbohydrates (C-type lectin domain [CTLD]-containing proteins). C-type lectins and proteins with CTLDs are found in all organisms. The large family of C-type lectins includes collectins, selectins, endocytic receptors, and proteoglycans. Some of these proteins are secreted and others are transmembrane proteins. They often oligomerize into homodimers, homotrimers, and higher-ordered oligomers, which increases their avidity for multivalent ligands. Although they share structural homology, C-type lectins usually differ significantly in the types of glycans that they recognize with high affinity. These proteins function as adhesion and signaling receptors in many immune functions such as inflammation and immunity to tumor and virally infected cells.
HISTORICAL BACKGROUND AND DISCOVERY OF C-TYPE LECTINS
The first C-type lectin identified in animals was the hepatic asialoglycoprotein receptor (ASGPR), also termed the hepatic galactose/N-acetylglucosamine receptor, as introduced in Chapter 26. It was discovered during studies on the structure and function of the sialylated serum glycoprotein, ceruloplasmin. Most serum glycoproteins contain terminal sialic acid residues, and it was observed that desialylated glycoproteins were rapidly removed from the blood. A key experimental result was that the clearance of enzymatically desialylated and radiolabeled ceruloplasmin depended on exposure of the penultimate galactose residue in ceroluplasmin, and removal or modification of the galactose caused retention in the circulation. The desialylated glycoproteins removed from the circulation were sequestered in the liver, principally in lysosomes.
A Ca++-dependent receptor specific for asialoglycoproteins was identified in hepatocyte plasma membrane fractions, and the ASGPR was purified by affinity chromatography on asialo-orosomucoid–Sepharose. The ASGPR was found to consist of a major subunit with a molecular mass of approximately 48 kD and a minor subunit with a mass of about 40 kD. The purified rabbit hepatocyte receptor agglutinated desialylated human and rabbit erythrocytes and also induced mitogenesis in desialylated peripheral lymphocytes. This was the first demonstration that an animal lectin could have such profound effects on cellular metabolism. Binding of rabbit hepatic lectin to cells was inhibited by both N-acetylgalactosamine and galactose, with the former being more potent than the latter, and the lectin bound best to glycoproteins containing either nonreducing terminal N-acetylgalactosamine or galactose. At the time of the discovery, it was not known that circulating glycoproteins had terminal N-acetylgalactosamine residues, but now we know that many pituitary glycoprotein hormones and parasite-derived glycoproteins contain terminal β-linked N-acetylgalactosamine residues and may be recognized by this hepatic receptor. A similar lectin in rats, which contained an unusual heterotrimeric structure composed of two subunits, was termed rat hepatic lectin R2/3. A related lectin identified in chicken hepatocytes recognized glycoproteins containing terminal N-acetylglucosamine residues. Thus, the avian liver rapidly cleared only glycoproteins that lacked both terminal sialic acid and galactose residues. Interestingly, circulating glycoproteins in birds appear to be constitutively desialylated compared to their mammalian counterparts.
DEFINITION OF C-TYPE LECTINS AND STRUCTURAL MOTIFS
The C-type lectin fold has been found in more than 1000 proteins, and it represents a ligand-binding motif that is not necessarily restricted to binding sugars. The CTLD broadly denotes proteins with a C-type lectin domain, regardless of their ability to bind sugars. In animals, C-type lectins are the major representatives of CTLD-containing proteins. In metazoans, most proteins with a CTLD are not lectins. Proteins use the C-type lectin fold to bind other proteins, lipids, inorganic molecules (e.g., Ca2CO3), or even ice (e.g., the antifreeze glycoproteins). Glycan binding by the C-type lectins is always Ca++-dependent because of specific amino acid residues that coordinate Ca++ and bind the hydroxyl groups of sugars. The C-type lectin fold is a rigid scaffold that can accommodate a remarkable number of sequence variations. An extreme example is seen in the major tropism determinant (Mtd), which is a receptor-binding protein of Bordetella bacteriophage. This C-type lectin fold may occur in at least 1013 different sequences, a diversity that rivals the immunoglobulin fold in its conservation of structure using millions of different primary amino acid sequences. Thus, the C-type lectin fold is an evolutionarily ancient structure that is adaptable for many uses.
The C-type lectin fold is unique. It is a compact domain of 110–130 amino acid residues with a double-looped, two-stranded antiparallel β-sheet formed by the amino-and carboxy-terminal residues connected by two α-helices and a three-stranded antiparallel β-sheet (Figure 31.1). The CRD has two highly conserved disulfide bonds and up to four sites for binding Ca++, with site occupancy depending on the lectin. Amino acid residues with carbonyl side chains are often coordinated to Ca++ in the CRD, and these residues directly bind to sugars when Ca++ is bound in site 2. A ternary complex may be formed between a sugar in a glycan, the Ca++ ion in site 2, and amino acids within the CRD. Changes in amino acids within the CRD may alter sugar specificity. Key conserved residues that bind sugars include the “EPN” and “WND” motifs within the CRD of C-type lectins (see mouse L-selectin and rat mannose-binding protein C in Figure 31.1). The presence of such motifs in the CRD, along with Ca++ binding in site 2 and other secondary structures (including hydrogen bond donors and acceptors flanking a conserved Pro residue in the double-loop region), allows predictions as to whether a CRD binds sugar. However, because the binding site is relatively shallow with few contacts to sugars, it is difficult to predict the glycan that binds to a particular C-type lectin. Nevertheless, sequence determinants in the CRD provide clues to the “monosaccharide” specificity of C-type lectins (e.g., mannose vs. galactose). In several C-type lectins, such as P-selectin and the ASGPR, Ca++ binding induces structural changes in the CRD that stabilize the double loop region. Loss of Ca++ can lead to destabilization of these loops and loss of ligand binding, even when Ca++ is not directly involved in complexing the ligand, as seen in the macrophage mannose receptor. This destabilization is also important in pH-induced changes that lead to loss of ligand-binding affinity, because of the pH-induced loss of Ca++. In CTLD-containing proteins such as human tetranectin, which is not known to bind sugars, Ca++ is also important for interactions with several kringle-domain-containing proteins.

FIGURE 31.1
Structure of C-type lectins. (a) Ribbon diagram of the carbohydrate-recognition domain (CRD) of rat mannose-binding protein A (MBP-A). (Light green spheres) Ca++-binding sites, where 1 is the auxiliary binding site and 2 is the principal binding site. (more...)
C-type lectins exist as both oligomers and monomers. Many C-type lectins occur as trimers, including the trimeric rat mannose-binding protein-A (MBP-A) in complex with α-methyl mannoside (Figure 31.2). The CRD of trimeric lectins is angled to the side of the stalk domain through which the protein associates to form the trimer. The CRDs are at the top of the trimer and can function to enhance multivalent interactions with carbohydrate ligands.

FIGURE 31.2
Crystal structure of trimeric rat mannose-binding protein-A complexed with α-methylmannoside. (Created from PDB deposited structure 1kwu and with permission of the American Society for Biochemistry and Biology from Ng K.K., Kolatkar A.R., Park-Snyder (more...)
DIFFERENT SUBFAMILIES OF C-TYPE LECTINS
There are at least 17 groups of proteins with CTLDs, which are distinguished by their domain architecture. Well over 100 different proteins encoded in the human genome contain the CTLD (Figure 31.3). Most of these groups have a single CTLD, but the macrophage mannose receptor (group VI) has eight of these domains. Groups VII (REG), IX (tetranectin), XI (attractin), XIII (DGCR2; DiGeorge syndrome critical region gene 2), XV (BIMLEC), and XVII (CBCP) have no known glycan ligands. From a functional perspective, we know most about collectins, endocytic receptors, myeloid lectins, and selectins, and these groups are discussed in detail below.

FIGURE 31.3
Different groups of C-type lectins and their domain structures. Seventeen groups are shown, defined by their phylogenetic relationships and domain structures. Some of the groups are soluble proteins and others are transmembrane proteins. The group III (more...)
CTLD-containing proteins are found in all metazoans and many nonmetazoans. This latter group includes bacterial toxins (e.g., pertussis toxin), outer-membrane adhesion proteins (e.g., invasin from Yersinia pseudotuberculosis), and viral proteins (e.g., envelope protein in Epstein–Barr virus). Interestingly, the viral proteins have more similarity to mammalian CTLD-containing proteins than the bacterial proteins.
There are at least 135 proteins with CTLDs within the genome of Caenorhabditis elegans, which has approximately 19,000 protein-coding sequences. Within this group, 183 C-type CTLDs have been found, because some proteins have multiple CTLDs. Interestingly, in response to pathogens, C. elegans expresses at least 10 genes that encode proteins with a CTLD out of the 68 pathogen up-regulated genes described to date.
THE COLLECTINS
The collectins are C-type lectins that contain a collagen-like domain and usually assemble in large oligomeric complexes containing 9–27 subunits (Figure 31.3). To date, nine different collectins have been identified: mannose-binding protein (MBP), conglutinin, surfactant proteins SP-A and SP-D, and collectins CL-43, CL-46, CL-P1, CL-L1, and CL-K1. The collectins MBP, conglutinin, CL-43, CL-46, CL-K1, SP-A, and SP-D are soluble, whereas CL-L1 and CL-P1 are membrane proteins. Some collectins, such as MBP and SP-A, organize into a “bouquet,” and others, such as bovine conglutinin and SP-D, organize into a “cruciform” shape. One of the best-studied serum collectins is MBP. Bovine CL-43 is structurally one of the simplest collectins, consisting of only three polypeptides, each of which contains a terminal CTLD. Rats have two serum MBPs designated A and C, sometimes called mannan-binding proteins. Humans appear to have only a single MBP corresponding to the rat MBP-A.
The collectins contribute to “innate” immunity and act before the induction of an antibody-mediated response. Collectins stimulate in vitro phagocytosis by recognizing surface glycans on pathogens, they promote chemotaxis, and they stimulate the production of cytokines and reactive oxygen species by immune cells. Lung surfactant lipids have the ability to suppress a number of immune cell functions such as proliferation, and this suppression of the immune response is further augmented by SP-A. Although SP-A and SP-D were originally found in the lung, they are also expressed in the intestine.
MBP forms a trimeric helical structure via interactions of the collagenous tails that are stabilized by disulfide bonds in the cysteine-rich aminoterminal region. These trimers aggregate to generate three or six trimers in a “bouquet” organization. Each CRD in the trimer is separated by approximately 53 Å, which is critical to the function of the lectin. This is because each individual CRD has a relatively low affinity and low specificity for glycan ligands and can bind to glycans rich in N-acetylglucosamine, N-acetylmannosamine, fucose, and glucose. The spacing between CRDs provides regulation and enhances the potential interactions with extended mannan-containing glycoconjugates, especially those on bacteria, yeast, and parasites.
Binding of MBP and other collectins to a target cell directly activates complement via the classical pathway and generates opsonic C3b fragments that coat pathogens and lead to their phagocytosis. For human MBP, this activation results from a novel type of C1s-like serine protease that complexes with MBP and initiates the complement cascade in vivo. Some individuals with MBP deficiency syndrome have mutations in the Gly-X-Y repeat encoded within exon-1 of the MBP gene (MBL2). Mutations within exon-1, which are highly variable among human populations, inhibit assembly of the MBP subunit, leading to increased risk of microbial infections. Furthermore, several polymorphisms within the promoter region of MBL2 are associated with MBP deficiency and enhanced susceptibility to infections.
THE ENDOCYTIC RECEPTORS
Many C-type lectins function in receptor-mediated endocytosis to deliver bound, soluble ligands to lysosomes (Figure 31.4). The ASGPR and most endocytic receptors are type II transmembrane proteins, whereas the macrophage mannose receptor is type 1. The cytoplasmic domains of endocytic C-type lectins, such as the ASGPR in hepatocytes, DC-SIGN (dendritic cell-specific intercellular adhesion molecule 3-grabbing nonintegrin) in myeloid cells, and P-selectin in platelets and endothelial cells, have internalization motifs that include triads of acidic amino acid and dileucine- or tyrosine-based motifs. Myeloid cells, including dendritic cells and macrophages, express a large number of C-type lectins. Endocytosis of ligands by C-type lectins in dendritic cells and macrophages can lead to receptor accumulation and degradation in phagolysosomes or to recycling of the receptor to the cell surface. The pathway taken is dependent on the bound ligand. For example, dectin-1 is degraded when it internalizes zymosan, but it is recycled when it endocytoses soluble ligands. Stimulation of C-type lectins such as dectin-1 in myeloid cells activates mitogen-activated protein kinase (MAPK) and NF-κB and enhances transcription of genes important in innate immune responses. Internalization of antigens via the C-type lectins in dendritic cells induces production of reactive oxygen species and other responses.

FIGURE 31.4
C-type lectins that function as endocytic receptors. Ligands are internalized by clathrin-dependent pathways and delivered to early and then late endosomes. Receptors may be recycled or degraded, depending on the receptor and the type of ligand it endocytoses. (more...)
The mammalian ASGPR is composed of major and minor subunits in different ratios that are encoded by two genes. The human ASGPR is a heterotetramer (H1 and H2 in a 3:1 ratio). The H2 transcript, however, exhibits splice variants H2a, H2b, and H2c, and the encoded proteins may contribute to different receptor structures and activities. The rat ASGPR is composed of three subunits, designated rat hepatic lectins (RHL) 1, 2, and 3. RHL1 is derived from a single gene, whereas RHL2 and RHL3 are derived from the same gene and have the same primary sequence, but they differ in glycosylation. In contrast, the chicken hepatic lectin is probably a homotrimer and contains a single subunit. These hepatic lectins occur in oligomeric forms that promote high affinity for specific glycoconjugate ligands. Clustering of the CRDs may determine both the specificity and affinity of the lectins for ligands because each individual CRD can act independently to bind sugar. Studies have shown that the precise orientation of the trimers within the ASGPR can dictate its affinity to specific glycans that have multivalent presentations. Tri- and tetra-antennary N-glycans with appropriate branching and presentation of nonreducing terminal galactose or N-acetylgalactosamine residues bind to the rat ASGPR with greater than 100,000 times higher affinity (~nM range) than ligands with a single terminal Gal/GalNAc residue. Interestingly, the rat hepatic ASGPR can also bind sialylated ligands (Siaα2-6Galβ1-4GlcNAc-R). The hepatic lectins have a single C-type CRD, whereas the macrophage mannose receptor has eight CRDs in a single polypeptide. The adjacent CRDs may help it to bind specific multivalent, mannose-containing glycans. The macrophage mannose receptor internalizes lysosomal enzymes containing high-mannose-type N-glycans and facilitates phagocytosis of several pathogens such as yeast, Pneumocystis carinii, and Leishmania.
Although the ASGPR was predicted to contribute to homeostasis and maintenance of serum glycoprotein levels, mice lacking subunit 2 have no obvious phenotypic abnormalities and do not accumulate endogenous glycoproteins in serum. However, the mutant mice have defective hepatic uptake and clearance of injected asialoglycoproteins. Thus, ASGPR may be required to regulate serum glycoprotein levels in periods of induced stress, which is known to elevate serum glycoprotein levels, and/or to be involved in specific interactions with glycoconjugates from pathogenic organisms. In the latter case, these hepatic receptors may function as part of the innate immune system, perhaps in a manner like the C-type lectins in myeloid cell function. For example, the human ASGPR promotes endocytosis-dependent entry of hepatitis B virus particles into liver cells. It is interesting that autoimmune hepatitis is associated with autoantibodies to the human hepatic ASGPR, and there is evidence that hepatitis virus invasion can augment this response.
Domains of C-type lectins other than the canonical CRD may also have receptor activity. For example, the cysteine-rich domain of the “mannose” receptor binds to glycans containing R-GalNAc-4-SO4 on pituitary glycoprotein hormones and thus acts to clear these hormones from the circulation.
THE MYELOID C-TYPE LECTINS
Myeloid cells have many C-type lectins, which belong mainly to groups II, V, and VI (see Figure 31.3). In addition, myeloid cells express many members of the galectin and Siglec families of lectins. C-type lectins in myeloid cells include in group II, DC-SIGN (in humans, but there is no murine homolog), SIGN-R1 (in mice, but there is no human homolog), macrophage C-type lectin (MCL), dectin-2, langerin, and the macrophage galactose-binding lectin (MGL); in group V, dectin-1, myeloid-DAP12-associating lectin (MDL-1), and dendritic-cell-associated lectin-1 (DCAL-1); and in group VI, macrophage mannose receptor and DEC-205.
Dendritic cells (DCs) are important antigen-presenting cells. They internalize antigens by fluid-phase pinocytosis and via specific endocytic receptors, and they process antigens for presentation to CD8+ cytotoxic T cells. DCs also contribute to the balance between tolerance and the induction of immunity and help to distinguish harmless “self-antigens” from pathogens (Figure 31.5). When DCs are activated, they migrate to lymph nodes where they interact with T cells. The types of antigens that DCs encounter lead to their differentiation into different subtypes, which function to instruct the differentiation of T cells. Toll-like receptors (TLRs) and C-type lectins (for myeloid cells, these have been termed C-type lectin receptors or CLRs) act to help DCs discriminate between pathogens and self-antigens. TLRs are pattern-recognition receptors (PRRs) that interact with “pathogen-associated molecular patterns” (PAMPs). TLRs, unlike CLRs, cannot directly promote phagocytosis of bound ligands. Ligation of TLRs can lead to DC maturation in a pathogen-specific manner. In contrast, DC interaction with pathogens through CLRs, in the absence of TLR activation, leads to internalization and processing of antigens and can result in DCs remaining immature. Interactions of T cells with antigens presented by immature DCs may lead to tolerogenic, rather than pathogenic, responses. However, if both TLRs and CLRs are activated and “cross-talk” ensues, DCs mature in different ways. For example, mycobacteria interact with DCs via TLR-2 and TLR-4, resulting in strong T-helper 1 (Th1) responses by the activated DCs. However, some virulent strains of mycobacteria secrete glycosylated factors (e.g., ManLAM) that are bound by CLRs, but not by TLRs, which lead to down-regulation of TLR activation and limitation of DC maturation.

FIGURE 31.5
C-type lectins function in innate immune responses and have a dual function in pathogen recognition and cell adhesion. Lectins expressed on dendritic cells (DCs) and macrophages interact with free pathogen-derived glycans or directly with pathogens, which (more...)
Dectin-1 is a type of natural killer (NK) cell C-type lectin (group V; see Figure 31.3) that binds ligands independently of Ca++. Dectin-1, which is also expressed on human neutrophils and macrophages, is a major PRR that recognizes glycan antigens such as β-glucans. When dectin-1 and TLR-2 are coligated by PAMPs, they coordinate the secretion of proinflammatory cytokines (interleukin-12 and tumor necrosis factor-α [TNF-α]) and the production of reactive oxygen species. Dectin-1 is degraded intracellularly upon internalization of large-sized β-glucans, but it can recycle upon internalizing small-sized β-glucans. Dectin-1 carries an immunoreceptor tyrosine-based activation motif (ITAM) in the cytoplasmic domain, which is involved in cell signaling through Syk family tyrosine kinases. DC-SIGN in human cells has been of special interest because it binds to the oligomannose-type N-glycans in the envelope glycoprotein of HIV-1. This protein also recognizes fucosylated ligands expressed by parasites such as the helminth Schistosoma mansoni.
Langerhans cells are a special type of DC and represent immature DCs found in the epidermis and mucosal tissues. These cells probably do not commonly coexpress the macrophage mannose receptor, but they do express many other C-type lectins, including langerin, which is a C-type lectin that largely recognizes glycans rich in mannose. Internalization of ligands by langerin leads to accumulation in Birbeck granules, which are subdomains of endosomes specifically found in Langerhans cells. Interestingly, polymorphisms in the gene encoding langerin are associated with changes in ligand specificity and loss of Birbeck granules.
THE SELECTINS
The selectins are perhaps the best-characterized family of C-type lectins because of their extensively documented roles as cell-adhesion molecules that mediate the earliest stages of leukocyte trafficking. The selectins are type-1 membrane proteins that are expressed on endothelial cells, leukocytes, and platelets. Interactions between the selectins and cell-surface glycoconjugate ligands play key roles in adhesive interactions among these cells (Figure 31.6). These interactions promote tethering and rolling of leukocytes and platelets on vascular surfaces, and are important for lymphocyte homing to secondary lymphoid organs and for leukocyte recruitment to sites of inflammation and injury. Rolling is a form of adhesion that requires rapid formation and dissociation of bonds between selectins and their ligands. Rolling adhesion enables leukocytes to encounter endothelium-bound chemokines. Signaling through chemokine receptors cooperates with signaling through selectin ligands to activate leukocyte integrins, which bind to immunoglobulin superfamily ligands on endothelial cells to slow rolling velocities and arrest leukocytes on vascular surfaces. The arrested leukocytes then emigrate across the vascular wall into the underlying tissues.

FIGURE 31.6
Tethering of circulating leukocytes to activated endothelium via interactions between selectins and their ligands. (a) In normal venules, leukocytes flow without adhesive interactions with the endothelium, but in inflamed vessels, selectins and integrin (more...)
The three selectins are L-selectin, which is expressed on all leukocytes; E-selectin, which is expressed by cytokine-activated endothelial cells; and P-selectin, which is expressed constitutively in α-granules of platelets, in Weibel–Palade bodies of endothelial cells, and on the surfaces of activated platelets and endothelial cells (Figure 31.7). Each selectin has a C-type CRD at the amino terminus followed by a consensus epidermal growth factor (EGF)-like domain and a number of short consensus repeats composed of sushi domains (also called complement control protein [CCP] modules). The proteins have a single transmembrane domain (TM) and a cytoplasmic domain and are relatively rigid, extended molecules. The C-type CRD of each selectin has modest affinity for the sialylated, fucosylated structure known as the sialyl Lewisx antigen NeuAcα2-3Galβ1-4(Fucα1-3)GlcNAcβ1-R (SLex). In addition, P- and L-selectin, but not E-selectin, bind to some forms of heparin/heparan sulfate. However, each of the selectins binds with higher affinity to specific macromolecular ligands. Many of the known ligands are mucins containing sialylated, fucosylated O-glycans. The major ligand for P-selectin, termed P-selectin glycoprotein ligand-1 (PSGL-1), has sulfated tyrosine residues adjacent to a core-2-based O-glycan expressing SLex. Ligands for L-selectin that occur within specialized endothelia termed high endothelial venules (HEV) contain 6-sulfo-SLex antigens on mucin-type O-glycans and on N-glycans.

FIGURE 31.7
Overall domain structures of P-selectin, E-selectin, L-selectin, and PSGL-1. (a) The domain organization of the selectins and their expression patterns are indicated. P-Selectin also forms homodimers in the membrane. The predicted disulfide-bonded dimeric (more...)
P-Selectin
P-Selectin (CD62P) was discovered as an antigen expressed on the surface of activated platelets. It is constitutively expressed in megakaryocytes, where it is packaged into the membranes of α-granules of circulating platelets. It is also expressed in the Weibel–Palade bodies of vascular endothelial cells. Within minutes following activation of either platelets or endothelial cells by proinflammatory secretagogues such as histamine, thrombin, or complement components, P-selectin is expressed on the cell surface because of fusion of the intracellular storage membranes with the plasma membrane. Sequences within the cytoplasmic domain of P-selectin mediate its sorting to secretory granules as well as its rapid endocytosis from the plasma membrane and movement from endosomes to lysosomes, where it is degraded. Splice variants of human P-selectin transcripts yield forms of P-selectin that lack a transmembrane domain, thus contributing to low-level soluble forms of P-selectin in the circulation. Leukocyte adhesion also stimulates proteolytic cleavage of the ectodomain of P-selectin from the plasma membrane, releasing it into the circulation. The inflammatory mediators TNF-α, interleukin 1-β (IL1-β), and lipopolysaccharide (LPS) augment transcription of mRNA for P-selectin in endothelial cells in mice but not in humans.
P-Selectin contributes to leukocyte recruitment in both acute and chronic inflammation. Mice that lack P-selectin exhibit defective rolling on endothelial cells of postcapillary venules, diminished recruitment of neutrophils or monocytes into tissues following injection of inflammatory mediators, and impaired recruitment of T cells into skin or other tissues following challenge with specific antigens. Mobilization of P-selectin to the surfaces of activated endothelial cells is important for all these responses. In addition, P-selectin expressed on the surfaces of activated platelets contributes to inflammation as well as to hemostasis and thrombosis. Activated platelets adhere through P-selectin to neutrophils, monocytes, NK cells, and some subsets of T lymphocytes. This adhesion augments the recruitment of leukocytes and platelets to sites of vascular injury. Expression of P-selectin on both platelets and endothelial cells contributes to experimental atherosclerosis in mice. Platelet-expressed P-selectin also stimulates monocytes to synthesize tissue factor, a key cofactor of blood coagulation that facilitates fibrin deposition during clot formation.
As discussed below, the major leukocyte counterreceptor for P-selectin is PSGL-1. P-Selectin binds weakly to some forms of heparin/heparan sulfate and to some glycoproteins that bear the SLex determinant. The physiological significance of this binding is unclear, but the levels of heparin that are clinically prescribed might be able to block P-selectin functions. In addition, P-selectin can interact with mucins containing highly clustered glycans bearing SLex antigens, which might be important in metastasis of tumors bearing such ligands (see Chapters 43 and 44). Heparin might be therapeutically useful in blocking cancer metastases through blocking adhesion of tumor cells to P-selectin.
PSGL-1
PSGL-1 (CD162) is a homodimeric, disulfide-bonded mucin with subunits with a molecular mass of approximately 120 kD (351 amino acids). It is the major physiological ligand on leukocytes for P- and L-selectin and is also an important ligand for E-selectin (Figure 31.7). The precursor to the PSGL-1 monomer has 402 amino acids, including an 18-amino-acid signal sequence. Maturation of the protein occurs following cleavage at residues 38–41 by a paired basic amino-acid-converting enzyme in leukocytes. There are 16 decapeptide repeating units with the consensus sequence spanning residues 118–277 in the ectodomain of the long form of the protein, which is the major form expressed in humans. Murine PSGL-1 has 397 amino acids with recognizable sequence similarity to the human sequence, but the mouse protein has only 10 decameric repeats with the consensus sequence -E-T-S-Q/K-P-A-P-T/M-E-A-, which is different from human PSGL-1. The highest homology between human and murine PSGL-1 occurs in the transmembrane and cytoplasmic domains.
PSGL-1 is primarily expressed in hematopoietic cells (including some hematopoietic stem cells), all neutrophils, monocytes, eosinophils, and basophils and certain subsets of T cells. PSGL-1 is also expressed in some activated endothelial cells, most notably the inflamed microvessels of the ileum in a spontaneous model of chronic ileitis in mice. When it undergoes the appropriate posttranslational modifications (see below), PSGL-1 interacts with each of the three selectins to support leukocyte rolling under flow. Engagement of PSGL-1 during rolling also transduces signals into leukocytes that activate leukocyte integrins to slow rolling velocities. Signaling through PSGL-1 also cooperates with signaling through chemokine receptors to elicit other effector responses in leukocytes.
PSGL-1 is heavily glycosylated; each subunit of human PSGL-1 has three potential sites for N-glycosylation and 70 serine and threonine residues in the extracellular domain that are potential sites for O-glycosylation. In addition, human PSGL-1 has three amino-terminal Tyr residues and murine PSGL-1 has two tyrosine residues that are potentially sulfated. Like most mucins, PSGL-1 has an extended structure (Figure 31.7).
Most of the O-glycans of native PSGL-1 purified from human HL60 cells are core-2 structures, of which only a subset are fucosylated. Optimal binding of P-selectin to PSGL-1 requires that the latter presents SLex on a specific, amino-terminal core-2 O-glycan and sulfate esters on specific amino-terminal tyrosine residues. Tyrosine sulfate residues are generated by two tyrosylprotein sulfotransferases (TPST-1 and -2), which transfer sulfate from the sulfate donor (phosphoadenosine phosphosulfate) to exposed tyrosine residues. Thus, the key binding domain of PSGL-1 resides in its amino-terminal region. There are many lines of evidence that support this conclusion. Antibodies to this region block binding of PSGL-1 to P- and L-selectin (but not to E-selectin, which interacts with more than one site on PSGL-1 and does not interact with sulfated tyrosines). Treatment of neutrophils with a cobra venom metalloproteinase that removes the first ten amino acids from the amino terminus of PSGL-1 abrogates its binding to P- and L-selectin. Site-directed mutagenesis of a specific O-glycosylated amino-terminal threonine and/or the three tyrosine residues in the amino terminus of PSGL-1 prevents binding to P- and L-selectin. Finally, synthetic glycosulfopeptides with the structure shown in Figure 31.8 bind to P-selectin with the same high affinity (~300 nM) as that of native PSGL-1.

FIGURE 31.8
The molecular interactions between P-selectin and the amino terminus of PSGL-1. The C-type carbohydrate-recognition domain (CRD) at the amino terminus of P-selectin is a relatively large binding site that interacts with tyrosine sulfate residues, peptide (more...)
The data point to a model in which the combination of tyrosine sulfate residues and oligosaccharides on the protein are required for high-affinity binding to P-selectin (Figure 31.7). The cocrystal structure of a PSGL-1-derived glycosulfopeptide with P-selectin confirms the complex and tight association between these binding partners. The interactions between the glycosulfopeptide and P-selectin result from a combination of hydrophobic and electrostatic contacts. These include contacts of at least two of the three tyrosine sulfate residues as well as other PSGL-1 amino acids with multiple residues within the P-selectin CRD. In addition, two hydroxyl groups in the fucose residue of SLex ligate the lectin domain-bound Ca++, and there are additional binding interactions with the hydroxyl groups of galactose and the –COOH group of Neu5Ac.
E-Selectin
E-Selectin (CD62E) was discovered as a leukocyte adhesion molecule expressed by activated vascular endothelial cells. In most tissues (the bone marrow and skin may be exceptions), endothelial cells do not constitutively express E-selectin. Cytokine-dependent transcriptional processes lead to an inducible expression of E-selectin on the surface of the endothelium. Inducible transcription of the E-selectin locus by TNF-α, IL1-β, and LPS is mediated at least in part through NF-κB-dependent events. In vitro, cytokine-dependent regulation of the E-selectin locus yields E-selectin expression beginning about 2 hours after cytokine treatment, with maximal expression at about 4 hours. E-Selectin expression then declines to basal levels within 12–24 hours in vitro, but E-selectin may be expressed chronically at sites of inflammation in vivo. Decline of E-selectin expression is associated with decreased transcription of the E-selectin locus, degradation of E-selectin transcripts, and internalization and turnover of E-selectin protein. Acute and chronic inflammatory conditions associated with E-selectin expression include sepsis, rheumatoid arthritis, and organ transplantation. E-Selectin cooperates with P- and L-selectin to recruit leukocytes to sites of inflammation.
Physiological ligands for E-selectin contain the SLex antigen and occur on neutrophils, monocytes, eosinophils, memory/effector T cells, and NK cells. Each of these cell types is found in acute and chronic inflammatory sites in association with the expression of E-selectin, which implicates E-selectin in the recruitment of these cells to such inflammatory sites. PSGL-1 is one of the physiological ligands for E-selectin, but E-selectin can also interact with several other glycoproteins that express the SLex antigen on either N- or O-glycans, including the E-selectin ligand-1, CD44, L-selectin (in humans), and possibly long-chain glycosphingolipids expressing the SLex antigen. These interactions may have a potential role in cancer metastasis (see Chapter 44).
L-Selectin
L-Selectin (CD62L) is expressed on the microvilli of most leukocytes, including all myeloid cells, naïve T and B cells, and some memory/effector T cells. L-Selectin was discovered through efforts to define molecules that facilitate recirculation of lymphoid cells from the intravascular compartment to the secondary lymphoid organs, including lymph nodes and Peyer’s patches, from which the lymphoid cells then return to the circulation through the lymphatic system. This recirculation process provides lymphocytes with the opportunity to encounter foreign antigens displayed by antigen-presenting cells within secondary lymphoid organs. Early studies indicated that blood lymphocytes enter lymph nodes in postcapillary venules within these organs. These postcapillary venules are lined with a specialized endothelium (HEV). Cells in the HEV are cuboid in shape and their surfaces are decorated with certain glycoproteins required for adhesion of lymphocytes to the HEV, leading to subsequent transmigration. L-Selectin also cooperates with P- and E-selectins to promote leukocyte recruitment of myeloid cells and memory/effector T cells to sites of infection or injury during acute or chronic inflammation. L-Selectin is proteolytically shed from the surface of activated leukocytes by a metalloproteinase TNF-α-converting enzyme (TACE).
Rolling mediated by L-selectin exhibits a counterintuitive “shear threshold” requirement (e.g., a minimum flow rate is required for leukocytes to roll). As the flow rate drops below this threshold, leukocytes roll faster and more unstably and then detach. Flow-enhanced rolling operates through a force-dependent mechanism. As the flow rate increases, the force applied to adhesive bonds between L-selectin and its ligands increases. At threshold levels, the force actually strengthens the bonds, which prolongs their lifetimes. These are called “catch bonds.” As the flow rate increases further, the applied force begins to weaken the bonds, which shortens their lifetimes. These are called“slip bonds.”Catch bonds are regulated by force-dependent straightening of the angle between the lectin and EGF domains of L-selectin, which affects how ligand dissociates from the binding interface on the lectin domain. Transitions between catch and slip bonds are also seen for interactions between P-selectin and PSGL-1 and probably also occur between E-selectin and its ligands. However, the E-selectin transitions occur at lower forces with less dramatic effects at physiologically relevant shear forces in the circulation.
Lymphocytes home to secondary lymphoid organs where they may encounter antigens. Lymphocytes can return to the blood through the thoracic duct. This process is termed lymphocyte recirculation or homing. Entry to the lymph occurs in these organs through the HEV, where cuboid cells express ligands for lymphocyte L-selectin. These ligands are called peripheral node addressins, a group of mucins expressed on the HEV of lymph nodes. They include the mucins CD34, Sgp200, GlyCAM-1, MAdCAM-1, endoglycan, endomucin, and the podocalyxin-like protein (PCLP).
A unique feature of the L-selectin ligands on HEV is the requirement for sulfated glycans, such as 6-sulfo-SLex on both core-2 O-glycans and on extended core-1 O-glycans. The 6-sulfo-SLex determinant is associated with the MECA-79 epitope on O-glycans (Figure 31.9), an antibody that binds to 6-sulfo-N-acetyllactosamine on extended core-1 O-glycans. The biosynthesis of the 6-sulfo-SLex determinant depends on two key α1-3 fucosyltransferases, FucT-VII and FucT-IV, along with at least four different sulfotransferases that may form the 6-sulfo-SLex determinant. Two of these sulfotransferases, GlcNAc6ST-1 and GlcNAc6ST-2, are both expressed in HEV and appear to be most important. Mice lacking FucT-VII or both FucT-VII and FucT-IV have dramatically reduced homing of lymphocytes to lymph nodes. Mice lacking both GlcNAc6ST-1 and GlcNAc6ST-2 do not express 6-sulfo-SLex or the MECA-79 epitope and exhibit markedly diminished lymphocyte homing to lymph nodes. A unique β1-3GlcNAcT generates the extended core-1 O-glycan; mice lacking both this β1-3GlcNAcT and the β1-6GlcNAcT branching enzyme for core-2 O-glycan biosynthesis do not express the MECA-79 antigen, but they have residual lymphocyte rolling on HEV and only a minimal decrease in lymphocyte numbers in peripheral and mesenteric lymph nodes. The residual L-selectin-dependent lymphocyte homing appears to result from 6-sulfo-SLex on N-glycans, suggesting that both N- and O-glycans on HEV glycoproteins contribute to L-selectin-dependent lymphocyte recirculation through lymph nodes.

FIGURE 31.9
Structures of fucosylated and sialylated O-glycans that have been shown to mediate selectin-dependent cell adhesion. In addition, the structures at the top may be found on N-glycans and may mediate interactions with L-selectin. The biosynthesis of these (more...)
L-Selectin also plays a role in adhesion of neutrophils, eosinophils, and monocytes to nonlymphoid vascular endothelium (see Figure 31.6). The major ligand for L-selectin in these inflammatory settings is PSGL-1 (see Figure 31.7), which is expressed on adherent leukocytes and may also be deposited on inflamed endothelial cells as fragments left behind by previously rolling leukocytes. Unlike the HEV ligands, PSGL-1 interacts with L-selectin through cooperative binding of its amino-terminal core-2 O-glycan capped with SLex and its amino-terminal sulfated tyrosines, analogous to but not identical to its interactions with P-selectin. In addition, an alternate ligand on endothelial cells is heparan sulfate. Mice lacking L-selectin have defects in neutrophil recruitment in the context of inflammation as well as defects in homing of naïve lymphocytes to secondary lymphoid organs.
THE NATURAL KILLER LYMPHOCYTE PROTEINS WITH C-TYPE LECTIN DOMAINS
The immunoglobulin-like receptors and NK cell receptors containing a CTLD are two families of major histocompatibility complex (MHC) class-I-specific receptors found on NK cells. NK receptors with the CTLD are largely in group V and are represented by the Ly49 family of receptors in mice and the NK complex (NKC) in humans (see Figure 31.3). The human NKC proteins include CD69, CD94, activation-induced C-type lectin (AICL), and mast-cell-function-associated antigen (MAFA). The murine Ly49 family includes the Ly49 group of about a dozen proteins, including CD94, NKG2-A, -C, -D, and -E.
NK receptors with a CTLD are either activating or inhibitory to immune responses. For the most part, these proteins function in targeting lymphocytes to cells that lack MHC class I antigens. The Ly49 and NKC proteins are disulfide-bonded, type II, homodimeric trans-membrane proteins. Most of these proteins express immunoreceptor tyrosine-based inhibitory motif (ITIM) motifs in their cytoplasmic domain. Dectin-1, which is not considered to be part of the Ly49 or NKC complex, is exceptional in that it contains an activating ITAM-like motif. In human NK cells, CD94 forms MHC class-I-specific, disulfide-linked, heterodimers with NKG2 family members, which also contain a CTLD. Ligand binding to the CTLDs of these NK receptors can trigger or inhibit target cell lysis by NK cells or can activate various hematopoietic cells.
Most of the NK receptors with CTLDs are not known to bind carbohydrate ligands and lack the conserved Ca++-binding residues and amino residues in most C-type lectins that bind glycans. In humans, it is known that Ca++ is not required for CD94/NKG2 interactions with HLA-E. The CTLD in the NK receptor has a somewhat different structure from typical C-type lectins and may have evolved to promote interactions with MHC class I glycoproteins. However, some recent studies suggest that murine CD69 may bind some sulfated glycans; in addition, another protein in the NK family, the osteoclast inhibitory lectin (OCIL) from humans and mice, also binds some sulfated polysaccharides in a Ca++-independent fashion. Thus, the possibility that the NK receptors with CTLDs bind to some types of glycan ligands should be explored. As discussed above, dectin-1 lacks the canonical residues for Ca++ binding and monosaccharide binding but nevertheless binds β-glucans. Thus, the CTLDs in the NK receptors may have evolved to recognize glycans by novel mechanisms.
The B-cell differentiation antigen (CD72) interacts with the B-cell receptor (BCR) and modulates BCR-mediated signals. CD72 is a type II membrane proteins with a CTLD that lacks the residues associated with Ca++ and monosaccharide binding. It has been speculated that CD72 might recognize BCR or other glycoprotein ligands by corecognizing glycan and protein determinants. The low-affinity IgE receptor, CD23, also contains a CTLD that interacts with CD21 and other ligands, but it may bind these ligands in a manner that depends on their correct glycosylation, perhaps a combination of glycan and peptide determinants.
PROTEOGLYCANS WITH C-TYPE LECTIN DOMAINS
The CTLD has also been identified in several proteoglycans (or lecticans) that lack trans-membrane domains and occur in the extracellular matrix (group I; see Figure 31.3). These include aggregan, brevican, versican, and neurocan. Like the selectins, each of these core proteins contains a CTLD, an EGF-like domain, and a CCP domain, but their order is different and they are located in the carboxyl terminus of the protein. A large region containing attachment sites for chondroitin sulfate and keratan sulfate is proximal to the lectin domain. A more complete discussion of the proteoglycans is provided in Chapter 16. The exact functions of the CTLD in these proteins are still unknown. The CTLD of rat aggrecan is important for Ca++-dependent binding to the fibronectin type II repeats 3–5 of rat tenascin-R. The CTLDs in other lecticans are probably also responsible for protein–protein interactions with other receptors, including tenascin-R, tenascin-C, and other tenascins, which are extracellular matrix glycoproteins highly expressed in the nervous system. Interestingly, the protein–protein interactions between rat fibronectin and the CTLD of rat aggregan bear resemblance to the protein–protein interactions seen in P-selectin binding to PSGL-1. Tenascin-R is one of the main carriers of the unusual glycan antigen HNK-1, which was named after its identification on human NK cells. Recent studies show that brevican, a lectican found in the nervous system, binds to the HNK-1-containing glycosphingolipids. Thus, the CTLD in lecticans may represent a versatile structural feature that can be used for both protein–protein and protein–glycan interactions.
OTHER TYPES OF C-TYPE LECTINS
A number of proteins with C-type CRDs have been identified in the pancreas and kidney, but the importance of the CRD and glycan binding to the function of these proteins is unclear. Autosomal dominant polycystic kidney disease (ADPKD) is a commonly occurring hereditary disease that accounts for about 10% of end-stage renal disease. PKD1, one of two recently isolated ADPKD gene products, has been implicated in cell–cell and cell–matrix interactions. The PKD1 gene encodes a novel protein named polycystin that has multiple cell-recognition domains, including a single CTLD at its amino terminus. The function of PKD1 and the effect of mutations on its possible activity are unclear. Interestingly, some C-type lectins can be extremely small; for example, HIP (hepatic intestinal pancreatic protein) and PSP (pancreatic stone protein), which are essentially isolated CTLDs that are preceded by a signal sequence.
Lower vertebrates, invertebrates, and some viruses have now been found to contain the CTLD, and some of these proteins have been shown to bind sugars. For example, the galactose-specific lectin from Crotalus atrox binds a variety of galactose-containing glycolipids in a Ca++-dependent fashion. A number of related venom proteins inhibit platelet function and/or the coagulation cascade. Alboaggregin A from Trimeresurus albolabris (the white-lipped pit viper) contains four subunits, and subunit 1 contains a single CTLD at its amino terminus. The protein binds to the platelet GPIb/IX receptor and stimulates platelet agglutination, but the potential role of glycan recognition in this process is presently unknown.
FURTHER READING
- Drickamer K. C-type lectin-like domains. Curr Opin Struct Biol. 1999;9:585–590. [PubMed: 10508765]
- Ravetch JV, Lanier LL. Immune inhibitory receptors. Science. 2000;290:84–89. [PubMed: 11021804]
- McEver RP. Selectins: Lectins that initiate cell adhesion under flow. Curr Opin Cell Biol. 2002;14:581–586. [PubMed: 12231353]
- Cambi A, Figdor CG. Dual function of C-type lectin-like receptors in the immune system. Curr Opin Cell Biol. 2003;15:539–546. [PubMed: 14519388]
- McGreal EP, Martinez-Pomares L, Gordon S. Divergent roles for C-type lectins expressed by cells of the innate immune system. Mol Immunol. 2004;41:1109–1121. [PubMed: 15476922]
- Cambi A, Koopman M, Figdor CG. How C-type lectins detect pathogens. Cell Microbiol. 2005;7:481–488. [PubMed: 15760448]
- Iovanna JL, Dagorn JC. The multifunctional family of secreted proteins containing a C-type lectin-like domain linked to a short N-terminal peptide. Biochim. Biophys. Acta. 2005;1723:8–18. [PubMed: 15715980]
- McMahon SA, Miller JL, Lawton JA, Kerkow DE, Hodes A, Marti-Renom MA, Doulatov S, Narayanan E, Sali A, Miller JF, Ghosh P. The C-type lectin fold as an evolutionary solution for massive sequence variation. Nat Struct Mol Biol. 2005;12:886–892. [PubMed: 16170324]
- Zelensky AN, Gready JE. The C-type lectin-like domain superfamily. FEBS J. 2005;272:6179–6217. [PubMed: 16336259]
- Zhu C, McEver RP. Catch bonds: Physical models and biological functions. Mol Cell Biomech. 2005;2:91–104. [PubMed: 16708472]
- Brown GD. Dectin-1: A signalling non-TLR pattern-recognition receptor. Nat Rev Immunol. 2006;6:33–43. [PubMed: 16341139]
- Chen M, Geng JG. P-Selectin mediates adhesion of leukocytes, platelets, and cancer cells in inflammation, thrombosis, and cancer growth and metastasis. Arch Immunol Ther Exp. 2006;54:75–84. [PubMed: 16648968]
- Kishore U, Greenhough TJ, Waters P, Shrive AK, Ghai R, Kamran MF, Bernal AL, Reid KB, Madan T, Chakraborty T. Surfactant proteins SP-A and SP-D: Structure, function and receptors. Mol Immunol. 2006;43:1293–1315. [PubMed: 16213021]
- Ludwig IS, Geijtenbeek TBH, van Kooyk Y. Two way communication between neutrophils and dendritic cells. Curr Opin Pharmacol. 2006;6:408–413. [PubMed: 16750420]
- Pyz E, Marshall ASJ, Gordon S, Brown GD. C-type lectin-like receptors on myeloid cells. Ann Med. 2006;38:242–251. [PubMed: 16754255]
- Robinson MJ, Sancho D, Slack EC, Leibund Gut-Landmann S, Reis e Sousa C. Myeloid C-type lectins in innate immunity. Nat Immunol. 2006;7:1258–1265. [PubMed: 17110942]
- Sperandio M. Selectins and glycosyltransferases in leukocyte rolling in vivo. FEBS J. 2006;273:4377–4389. [PubMed: 16956372]
- Uchimura K, Rosen SD. Sulfated L-selectin ligands as a therapeutic target in chronic inflammation. Trends Immunol. 2006;27:559–565. [PubMed: 17049924]
- Zhou T, Chen Y, Hao L, Zhang Y. DC-SIGN and immunoregulation. Cell Mol Immunol. 2006;3:279–283. [PubMed: 16978536]
- Gupta G, Surolia A. Collectins: Sentinels of innate immunity. BioEssays. 2007;29:452–464. [PubMed: 17450595]
- Trinchieri G, Sher A. Cooperation of Toll-like receptor signals in innate immune defence. Nat Rev Immunol. 2007;7:179–190. [PubMed: 17318230]
- HISTORICAL BACKGROUND AND DISCOVERY OF C-TYPE LECTINS
- DEFINITION OF C-TYPE LECTINS AND STRUCTURAL MOTIFS
- DIFFERENT SUBFAMILIES OF C-TYPE LECTINS
- THE COLLECTINS
- THE ENDOCYTIC RECEPTORS
- THE MYELOID C-TYPE LECTINS
- THE SELECTINS
- THE NATURAL KILLER LYMPHOCYTE PROTEINS WITH C-TYPE LECTIN DOMAINS
- PROTEOGLYCANS WITH C-TYPE LECTIN DOMAINS
- OTHER TYPES OF C-TYPE LECTINS
- FURTHER READING
- Review C-Type Lectins.[Essentials of Glycobiology. 2015]Review C-Type Lectins.Cummings RD, McEver RP. Essentials of Glycobiology. 2015
- Review C-Type Lectins.[Essentials of Glycobiology. 2022]Review C-Type Lectins.Cummings RD, Chiffoleau E, van Kooyk Y, McEver RP. Essentials of Glycobiology. 2022
- Review Targeting C-type lectin receptors with multivalent carbohydrate ligands.[Adv Drug Deliv Rev. 2013]Review Targeting C-type lectin receptors with multivalent carbohydrate ligands.Lepenies B, Lee J, Sonkaria S. Adv Drug Deliv Rev. 2013 Aug; 65(9):1271-81. Epub 2013 May 30.
- Review The C-type lectin superfamily in the immune system.[Immunol Rev. 1998]Review The C-type lectin superfamily in the immune system.Weis WI, Taylor ME, Drickamer K. Immunol Rev. 1998 Jun; 163:19-34.
- C-Type lectin-like domains in Caenorhabditis elegans: predictions from the complete genome sequence.[Glycobiology. 1999]C-Type lectin-like domains in Caenorhabditis elegans: predictions from the complete genome sequence.Drickamer K, Dodd RB. Glycobiology. 1999 Dec; 9(12):1357-69.
- C-type Lectins - Essentials of GlycobiologyC-type Lectins - Essentials of Glycobiology
- Glycans in Biotechnology and the Pharmaceutical Industry - Essentials of Glycobi...Glycans in Biotechnology and the Pharmaceutical Industry - Essentials of Glycobiology
- docking protein 4 isoform 1 [Homo sapiens]docking protein 4 isoform 1 [Homo sapiens]gi|2030424401|ref|NP_001381588.1|Protein
- Homo sapiens chromosome 16 clone RP11-250E14, complete sequenceHomo sapiens chromosome 16 clone RP11-250E14, complete sequencegi|29124041|gnl|lanlchgs|250E14|gb| 052.8|Nucleotide
Your browsing activity is empty.
Activity recording is turned off.
See more...