NCBI Bookshelf. A service of the National Library of Medicine, National Institutes of Health.
Varki A, Cummings RD, Esko JD, et al., editors. Essentials of Glycobiology. 2nd edition. Cold Spring Harbor (NY): Cold Spring Harbor Laboratory Press; 2009.
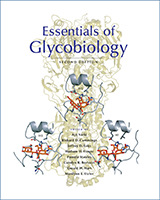
Essentials of Glycobiology. 2nd edition.
Show detailsThis chapter provides an overview of glycosylation from the perspective of a single cell, taking into account the patterns of expression, topology, and other features of the biosynthetic and degradative enzymes that are common to most cell types. The focus is mainly on eukaryotic cells, for which more information is available.
GLYCOSYLATION IS UNIVERSAL IN LIVING ORGANISMS
It is a remarkable fact that every free-living cell and every cell type within multicellular organisms is covered with a dense and complex layer of glycans. Even enveloped viruses that bud from surfaces of infected cells carry with them the glycosylation patterns of the host cell. Additionally, most secreted molecules are glycosylated and the extracellular matrices of multicellular organisms are rich in glycans and glycoconjugates. The matrices secreted by unicellular organisms when they congregate (e.g., bacterial biofilms; see Chapter 20) also contain glycans. The reason for the apparent universality of cell-surface and secreted glycosylation is not clear, but it suggests that evolution has repeatedly selected for glycans as being the most diverse and flexible molecules to position at the interface between cells and the extracellular milieu. For example, the enormous diversity, complexity, and flexibility of glycans may allow host cells to make changes to avoid pathogens, without causing major deleterious effects on cellular functions.
Like membrane proteins, secretory proteins in eukaryotic cells typically pass through an endoplasmic reticulum (ER)-Golgi pathway, the cellular system in which many major glycosylation reactions occur (see below). Perhaps for this reason, most proteins in the blood plasma of animals (with the exception of albumin) are also heavily glycosylated. Glycosylation of secreted proteins may provide solubility, hydrophilicity, and negative charge, thus reducing unwanted nonspecific intermolecular interactions in extracellular spaces and also protecting against proteolysis. Another, not mutually exclusive, hypothesis is that the glycans on secreted molecules act as decoys, binding pathogens that seek to recognize cell-surface glycans to initiate invasion.
In bacteria, archaea, and fungi, glycans have critical structural roles in forming the cell wall and in resisting large differences in osmolarity between the cytoplasm and the environment. Glycans surrounding bacteria could also have a role in defense against bacteriophages or antibiotics generated by other microorganisms in the environment.
TOPOLOGICAL ISSUES RELEVANT TO GLYCAN BIOSYNTHESIS
The ER-Golgi Pathway of Eukaryotes
Studies stemming from the classic work of George Palade and colleagues have indicated that most cell-surface and secreted molecules in eukaryotic cells originate in the ER. They then make their way via an intermediate compartment through multiple stacks of the Golgi apparatus, finally being distributed to various destinations from the trans-Golgi network. Along the way, lipids and proteins are modified by a variety of glycosylation reactions mediated by glycosyltransferases (see Chapter 5). Figure 3.1 superficially depicts some steps in the synthesis of the major glycan classes in the ER-Gogli pathway of animal cells. These pathways are discussed in the following sections and in other chapters of this book. As mentioned earlier, the ER-Golgi pathway is a universal feature of eukaryotic cells and also harbors other glycan-modifying enzymes (see below).

FIGURE 3.1
Initiation and maturation of the major types of eukaryotic glycoconjugates in relation to sub-cellular trafficking in the ER-Golgi–plasma membrane pathway. This illustration outlines the different mechanisms and topology for initiation, trimming, (more...)
Not all glycans and glycoconjugates assemble within the ER-Golgi pathway. For example, many cytoplasmic and nuclear proteins contain O-GlcNAc, O-Glc, or O-Fuc, and these modifications occur in the cytoplasm (see Chapters 17 and 18). Hyaluronan and chitin assembly occurs at the plasma membrane, with direct extrusion into the extracellular matrix (see Chapter 15). In plant cells, cellulose synthesis also occurs at the plasma membrane (see Chapter 22).
Regardless of their location, most glycosylation reactions use activated forms of monosaccharides (most often nucleotide sugars) as donors for reactions that are catalyzed by enzymes called glycosyltransferases (see Chapter 4 for a listing of these enzymes and details about their biochemistry). A variety of glycan modifications are also found in nature (see Chapter 5). Of these, the most common are generated by sulfotransferases, acetyltransferases, and methyltransferases, which use activated forms of sulfate (3′phosphoadenyl-5′-phosphosulfate; PAPS), acetate (acetyl-CoA), and methyl groups (S-adenosylmethionine; AdoMet), respectively. Almost all the donors for glycosylation reactions and glycan modifications are synthesized within the cytoplasmic compartment from precursors of endogenous origin. In eukaryotes, most of these donors must be actively transported across a membrane bilayer in order to become available for reactions within the lumen of the ER-Golgi pathway.
Much effort has gone into understanding the mechanisms of glycosylation and glycan modification within the ER and the Golgi apparatus, and it is clear that a variety of interacting and competing factors determine the final outcome of the reactions. The glycosyltransferases, processing glycosidases, and sulfotransferases are well studied (see Chapter 5), and their location has helped to define various functional compartments of the ER-Golgi pathway. A popular model envisioned these enzymes as being physically lined up along this pathway in the precise sequence in which they actually work. In fact, there is considerable overlap of the enzymes across Golgi stacks and the actual distribution of a given enzyme depends on the cell type.
Some glycan chains are made on the cytoplasmic face of intracellular membranes and flipped across to the other side, but most are added to the growing chain on the inside of the ER or the Golgi (see Figure 3.1). Regardless, the portion of a molecule that faces the inside of the lumen of the ER or Golgi will ultimately face the outside of the cell or the inside of a secretory granule or lysosome. To date there are no well-documented exceptions to this topological rule. A consequence of this topological asymmetry is that many classes of glycans are optimized to be involved in cell–cell and cell–matrix interactions. Of course, these topological considerations are reversed for nuclear and cytoplasmic glycosylation (see below), because the active sites of the relevant glycosyltransferases for these reactions face the cytoplasm. Perhaps not surprisingly, the types of glycans found on the two sides of the cell membrane are generally quite distinct from each other.
Glycosylation Pathways in Eubacteriae and Archaea
Much less is known about the topology of glycoconjugate assembly in eubacteriae and Archaea (formerly grouped as prokaryotes). Bacterial cells perform most glycosylation reactions on the inner aspect of the cytoplasmic membrane, using precursors assembled in the cytoplasm (see Chapter 20). These glycan intermediates are then flipped across the cytoplasmic membrane and used to form polymeric (often large) structures in the periplasm (see Chapter 21). In gram-negative organisms, which have an outer membrane, some of the glycans or glycoconjugates must be transferred between membranes or flipped across the outer membrane. The mechanisms underlying these processes are active areas of research. Even less is known about the topology of glycosylation pathways in Archaea.
GOLGI ENZYMES SHARE SECONDARY STRUCTURE
Despite the lack of sequence homology among different families of glycosyltransferases and sulfotransferases, almost all Golgi enzymes share some features. Early studies of the cell biology and biochemistry of vertebrate glycosyltransferases indicated that some of these activities could be found in soluble form in secretions and body fluids; others were identified as membrane-bound activities within cells, and some exhibited both properties. Cell fractionation studies generally found cell-associated transferase activities in membrane-rich microsomal fractions, which could be liberated in soluble form with the aid of detergents. These observations implied that some transferases probably represent membrane-spanning proteins, whereas others correspond to secreted proteins. However, following the initial molecular cloning efforts that defined the sequences of a β1-4 galactosyltransferase, an α2-6 sialyltransferase, and the blood group A α1-3 N-acetylgalacto-saminyltransferase, it became clear that Golgi glycosyltransferases share a common secondary structure that could account for all previous findings.
Almost all Golgi glycosyltransferases and sulfotransferases described to date have a single transmembrane domain flanked by a short amino-terminal domain and a longer carboxy-terminal domain. This structure is characteristic of so-called type II transmembrane proteins, whose single amino-terminal membrane-spanning domain functions as a signal-anchor sequence, placing the short amino-terminal segment within the cytoplasm while directing the larger carboxy-terminal domain to the other side of the biological membrane into which the signal anchor has been inserted (Figure 3.2). For plasma-membrane-associated type II proteins, the “other side” is the extracellular surface. For glycosyltransferases, the “other side” is the lumen of the membrane-delimited compartments that constitute the ER-Golgi pathway. These include vesicles that transit from the ER to the cis cisterna of the Golgi, the cisternae of the Golgi apparatus itself, the vesiculotubular network of the trans-Golgi network, and membrane-delimited structures distal to the trans-Golgi network. This arrangement predicts that the larger carboxy-terminal domain contains the catalytic activity of the transferase, and this supposition has extensive experimental support. The intralumenal location of this domain allows it to participate in the synthesis of the growing glycans displayed by glycoproteins and glycolipids during their transit through the secretory pathway.

FIGURE 3.2
Typical transmembrane topology and proteolytic processing of Golgi glycosyltransferases. Golgi glycosyltransferases and sulfotransferases generally have a single hydrophobic segment (TM) that functions as a signal-anchor sequence. This segment spans the (more...)
The type II transmembrane topology predicted by initial sequences of vertebrate glycosyltransferases has been widely confirmed experimentally. The topology may also explain reports of the expression of glycosyltransferases at the surface of mammalian cells. Interestingly, several other types of Golgi enzymes (e.g., processing glycosidases) that have been cloned share a similar topological arrangement. There are a few clear exceptions, such the UDP-GlcNAc:lysosomal enzyme N-acetylglucosamine-1-phosphotransferase (GlcNAc-phosphotransferase) and the GlcNAc-1-phosphodiester α-N-acetylglucosaminidase that are both involved in the synthesis of the Man-6-P targeting signal of newly synthesized lysosomal hydrolases (see Chapter 30). The former is a multisubunit complex, and the latter is a type I membrane-spanning glycoprotein with its amino terminus in the lumen of the Golgi apparatus. One of the sulfotransferases involved in heparan sulfate synthesis (GlcNAc 3-O-sulfotransferase 1) is a resident soluble enzyme in the Golgi. Likewise, the protein O-fucosyltransferase (Notch FucT) appears to be a soluble enzyme in the ER.
All of the above considerations do not apply to the glycosyltransferases involved in nuclear and cytoplasmic glycan synthesis. For example, the soluble GlcNAc transferase responsible for synthesizing the O-linked GlcNAc of nuclear and cytoplasmic proteins (see Chapter 18) has no detectable homology with the Golgi GlcNAc transferases. Another variation is presented by the hyaluronan and cellulose synthases, which are multipass membrane proteins present in the plasma membrane, extruding their products directly into the extracellular space. Similarly, all of the enzymes that use dolichol-linked precursors (and, in bacteria, bactoprenol-linked precursors) have more complex multi-membrane-spanning structures and contain a motif thought to bind to the isoprenoid chain.
GLYCOSYLTRANSFERASES CAN ALSO BE GLYCOSYLATED
Many Golgi glycosyltransferases have consensus N-glycosylation sequences as well as serine and threonine residues that could be modified by glycosylation processes. Biochemical analyses indicate that many mammalian glycosyltransferases are indeed posttranslationally modified by glycosylation, especially N-glycosylation. Glycosylation is, in some instances, required for proper folding and/or activity, and a few studies indicate that glycosyltransferases are subject to “autoglycosylation.” There is also limited evidence that glycosyltransferases may be modified by phosphorylation. The functional relevance of such posttranslational modifications remains unknown.
LOCALIZATION OF GLYCOSYLTRANSFERASES IN GOLGI COMPARTMENTS
Biochemical and ultrastructural studies indicate that glycosyltransferases partially segregate into distinct compartments within the secretory pathway. Generally speaking, enzymes acting early in glycan biosynthetic pathways have been localized to cis and medial compartments of the Golgi, whereas enzymes acting later in the biosynthetic pathway tend to colocalize in the trans-Golgi cisternae and the trans-Golgi network. These observations have prompted extensive exploration of the mechanisms whereby glycosyltransferases achieve this compartmental segregation. An effort was made to find Golgi-retention sequences, by analogy with the KDEL tetrapeptide implicated in retention/retrieval of ER-associated proteins. Although some general conclusions arise from these studies, the reader should consider the following caveats:
- Observations made with one enzyme are not necessarily applicable to others.
- The Golgi-retention properties of any given glycosyltransferase may vary depending on the cell type in which localization is examined.
- Variations in the expression level of a glycosyltransferase in an experimental system can have a major influence on retention/localization properties.
- Many studies used chimeric proteins composed of segments of a glycosyltransferase fused to a reporter protein, but conclusions from such experiments have not always been verified using intact glycosyltransferases or chimeras with a different reporter protein.
- In vitro studies using intact Golgi compartments indicate some spatial and functional overlap among enzymes that were previously thought to be segregated on the basis of data from other less sensitive techniques.
Most information relevant to the retention of glycosyltransferases within specific Golgi compartments derives from experiments done with an α2-6 sialyltransferase (ST6Gal-I), a β 1-4 galactosyltransferase (GalT-I), and an N-acetylglucosaminyltransferase I (GlcNAcT-I). The former pair of enzymes tends to concentrate in the trans-Golgi compartments and the trans-Golgi network, whereas GlcNAcT-I localizes mostly to the medial-Golgi compartment. With respect to ST6Gal-I, multiple signals and mechanisms may be involved in its Golgi localization. The transmembrane domain and flanking sequences are sufficient to direct heterologous proteins to the Golgi, and it appears that it is the length of the transmembrane segment (provided it is hydrophobic in nature) and not the precise sequence of this domain that is critical. Replacement of this region with a longer unrelated hydrophobic sequence does not compromise the Golgi localization of the intact enzyme, suggesting that other sequences are involved in localization. Recent evidence suggests that the cytoplasmic tail of this protein may mediate an additional localization mechanism and that the enzyme’s lumenal sequences are involved in a secondary oligomerization event that stabilizes Golgi retention.
In contrast, an examination of the Golgi-retention determinants of GalT-I points mainly to an important role for the transmembrane domain. The sequences that flank the membrane-spanning domain seem less important in Golgi retention for this enzyme. Retention of GlcNAcT-I is also dictated largely by its transmembrane domain, although its lumenal sequences are also involved in an oligomerization mechanism that has a role in its localization. Considered together, the available experimental observations suggest that the localization of glycosyltransferases within specific regions of the Golgi apparatus is probably not determined by simple primary sequence motifs. Rather, this process is likely determined by several different regions of each enzyme that mediate multiple redundant localization mechanisms.
Golgi localization may also be mediated by retention (in the context of the vesicular transport model) and continuous retrieval to earlier Golgi cisternae (in the context of the cisternal maturation model). Three models have been proposed to account for the localization of glycosyltransferases to specific Golgi subcompartments. In the oligomerization/kin-recognition model, glycosylation enzymes form homooligomers or heterooligomers through interactions between their transmembrane and lumenal sequences after arriving at the proper Golgi compartment. Heterooligomerization or kin recognition among enzymes in the same pathway would also presumably enhance the efficiency of the sequential glycosylation reactions. Some glycosylation enzymes have been found to form homooligomers (e.g., ST6Gal-I, GlcNAcT-1 and GlcNAcT-2), and oligomerization appears to have a role in their stable localization in the correct Golgi compartment. Experimental support for kin recognition comes from the observation that some pairs of glycosyltransferases known to catalyze sequential reactions in the same pathway colocalize to a specific Golgi compartment and are coimmunoprecipitated from cell extracts. However, this type of association has not been demonstrated for most of the enzymes.
A second model depends on partitioning the glycosyltransferases into lipid bilayers of different thicknesses. This bilayer thickness or lipid-partitioning model was proposed on the basis of observations that a cholesterol concentration gradient in the secretory pathway yields lipid bilayers of increasing thickness in the direction of cis to trans across the Golgi stack and that the transmembrane regions of glycosyltransferases are generally shorter than those of plasma membrane proteins. The model predicts that each glycosyltransferase sorts itself into the proper Golgi location by virtue of the length of its transmembrane segment, which will retain the enzyme once it reaches the proper compartment during the enzyme’s transit through the secretory pathway. This model was formulated largely on the basis of experiments involving ST6Gal-I, where the length of the membrane-spanning domain appears to play an important part in Golgi retention. However, the general applicability of this model is not apparent because there is no consistent relationship between the length of the transmembrane segment and retention in a specific Golgi compartment for a variety of glycosyltransferases. This model may therefore help to explain the overall phenomenon of retention of glycosyltransferases in the Golgi apparatus versus the delivery of other membrane proteins to the plasma membrane.
The third mechanism is based largely on the cisternal maturation model of transport through the secretory pathway. In this model, a new Golgi cisterna containing cargo molecules forms at the cis face of the stack and it progressively “matures” as Golgi glycosylation enzymes that define each subcompartment are transported into the new cisterna from the old cisterna to modify the cisternal cargo proteins. In this model, the steady-state localization of the Golgi enzymes is maintained by continuous retrograde transport. The cytoplasmic tails of enzymes may mediate interactions with coat proteins that select the enzymes for transport in vesicles or tubules. In support of this mechanism, the cytoplasmic tails of some glycosylation enzymes, such as ST6Gal-I and FucT-I, have been shown to have a role in their Golgi localization.
Taken together, evidence suggests that glycosylation enzymes use multiple mechanisms to maintain their localization in the Golgi. The number of signals and mechanisms used by an enzyme could determine how stable its Golgi localization actually is, whether it is able to move to a later compartment, and whether it can be cleaved and secreted into the extra-cellular space (see below).
PROTEOLYTIC CLEAVAGE AND SECRETION OF GOLGI GLYCOSYLTRANSFERASES
Many Golgi enzymes are secreted by cells, sometimes in large quantities, and can be found in cell culture supernatants and various body fluids. The nature of the secreted forms of glycosyltransferases first became clear from analysis of amino-terminal peptide sequences of purified mammalian glycosyltransferases that had been isolated as soluble forms without the aid of detergents. These studies showed that the soluble forms were actually derived from their membrane-associated forms by virtue of one or more proteolytic cleavage events that occurred a short distance away from the transmembrane segment within the stem region (Figure 3.2). These proteolytic cleavage events release a catalytically active fragment of the glycosyltransferase from its transmembrane tether and allow the cell to export this fragment to the extracellular space. The existence of catalytically active fragments of glycosyltransferases that are also deficient in various portions of the stem region together with mutational analyses imply that the stem region contributes little to the catalytic function of a glycosyltransferase. Nevertheless, some experimental analyses suggest that peptide sequences within the stem region can contribute to acceptor substrate preference.
The signals within the glycosyltransferase sequence that direct proteolysis are not defined, but it appears that the proteolytic cleavages are relatively specific and are generated by proteases functioning in the trans regions of the Golgi apparatus and beyond. The production of these soluble enzymes from cell types such as hepatocytes and endothelium can also be dramatically up-regulated under certain inflammatory conditions. Because these circulating enzymes do not have access to adequate concentrations of donor nucleotide sugars (primarily located inside cells), they should be functionally incapable of performing a transfer reaction in the extracellular spaces. The biological significance of these soluble transferases therefore remains a mystery. Possibilities to consider include a lectin-like activity recognizing their acceptor substrates and/or a role in scavenging small amounts of circulating sugar nucleotides that might otherwise be available to certain microbes, such as gonococci.
TURNOVER AND RECYCLING OF GLYCANS
Like all components of living cells, glycans turn over constantly. Some glycoconjugates, such as transmembrane heparan sulfate proteoglycans, turn over by shedding from the cell surface through limited proteolysis. Most glycoconjugate turnover occurs by endocytosis and subsequent degradation in lysosomes (see Chapter 41). Endoglycosidases can initially cleave glycans internally, producing substrates for exoglycosidases in the lysosome. Once broken down, individual monosaccharides are then typically exported from the lysosome into the cytoplasm, so that they can be reused (see Figure 1.8, Chapter 1). In contrast to the relatively slow turnover of glycans derived from the ER-Golgi pathway, glycans of the nucleus and cytoplasm may be more dynamic and rapidly turned over (see Chapters 17 and 18). Glycans in bacterial cells (especially those in the cell wall) also turn over during cell division when the cell wall undergoes cleavage and remodeling.
NUCLEAR AND CYTOPLASMIC GLYCOSYLATION IS VERY COMMON
Until the mid-1980s, a commonly stated dogma was that glycoconjugates such as glycoprotein and glycolipids occur exclusively on the outer surface of cells, on the internal (lumenal) surface of intracellular organelles, and on secreted molecules. As discussed above, this was in accord with information about the topology of the biosynthesis of the classes of glycans known at the time, which took place within the lumen of the Golgi-ER pathway. Thus, despite some clues to the contrary, the cytoplasm and nucleus (which are topologically semicontinuous because of the existence of nuclear pores) were assumed to be devoid of glycosylation capacity. However, it has now become clear that certain types of glycoconjugates are synthesized and reside within the cytoplasm and nucleus. Indeed, one of them (O-linked GlcNAc; see Chapter 18) may well be numerically the most common type of glycoconjugate in many cells.
GLYCOSYLATION REACTIONS AT THE PLASMA MEMBRANE
Because prokaryotic cells do not have an ER-Golgi pathway, they typically generate their cell-surface glycans at the interface of the cytoplasmic membrane and the cytoplasm or in the periplasm (see Chapter 20). Other glycans assembled at the plasma membrane include hyaluronan in vertebrate cells, chitin in invertebrate cells, and cellulose in plant cells. The topological difficulties of using cytoplasmic hydrophilic sugar nucleotides to manufacture glycans that are found on the opposite side of the cell-surface membrane are obvious. In eukaryotes, the process appears to involve enzyme molecules with multiple passes through the membrane that also act as a pore. In bacteria, membrane flippases may exist to aid in the transfer of intermediates across the various membranes. On the other hand, typical Golgi enzymes have also been claimed to be present at the cell surface in some animal cell types, with their active sites facing the extracellular space. It is not known how they could function at this location or how the nucleotide sugar donors would be delivered to this location. On the other hand, there are examples of remodeling of cell-surface glycans in animal cells, e.g., the sulf enzymes that modify heparan sulfate glycosaminoglycan (see Chapter 16) and sialidases that may remove cell-surface sialic acids (see Chapter 14).
GLYCOSYLATION IN UNEXPECTED SUBCELLULAR LOCATIONS
There are scattered reports of glycosylation in unexpected subcellular locations, for example, gangliosides in mitochondria and N-glycans in the nucleus. Many of these claims are based on incomplete evidence (see Chapter 17 for some discussion of these issues). One possibility is that there are indeed glycans in these unexpected cellular locations, but that their true structures are actually novel. Conversely, there are instances where the structural evidence is strong, but there is inadequate evidence to be certain about the topology of the claimed structures. Regardless, past experience tells us that the cell biology of glycosylation can hold many surprises, and dogmatic positions about such controversial issues are not warranted.
FURTHER READING
- Paulson JC, Colley KJ. Glycosyltransferases. Structure, localization, and control of cell type-specific glycosylation. J. Biol. Chem. 1989;264:17615–17618. [PubMed: 2681181]
- Bretscher MS, Munro S. Cholesterol and the Golgi apparatus. Science. 1993;261:1280–1281. [PubMed: 8362242]
- Baenziger JU. Protein-specific glycosyltransferases: How and why they do it. FASEB J. 1994;8:1019–1025. [PubMed: 7926366]
- Colley KJ. Golgi localization of glycosyltransferases: More questions than answers. Glycobiology. 1997;7:1–13. [PMC free article: PMC7108620] [PubMed: 9061359]
- Glick BS, Elston T, Oster G. A cisternal maturation mechanism can explain the asymmetry of the Golgi stack. FEBS Lett. 1997;414:177–181. [PubMed: 9315681]
- Varki A. Factors controlling the glycosylation potential of the Golgi apparatus. Trends Cell Biol. 1998;8:34–40. [PubMed: 9695806]
- Munro S. Localization of proteins to the Golgi apparatus. Trends Cell Biol. 1998;8:11–15. [PMC free article: PMC7172754] [PubMed: 9695801]
- Opat AS, van Vliet C, Gleeson PA. Trafficking and localization of resident Golgi glycosylation enzymes. Biochimie. 2001;83:763–773. [PubMed: 11530209]
- Mironov AA, Beznoussenko GV, Polishchuk RS, Trucco A. Intra-Golgi transport: A way to a new paradigm? Biochim Biophys Acta. 2005;1744:340–350. [PubMed: 15979506]
- Czlapinski JL, Bertozzi CR. Synthetic glycobiology: Exploits in the Golgi compartment. Curr. Opin. Chem. Biol. 2006;10:645–651. [PubMed: 17056297]
- GLYCOSYLATION IS UNIVERSAL IN LIVING ORGANISMS
- TOPOLOGICAL ISSUES RELEVANT TO GLYCAN BIOSYNTHESIS
- GOLGI ENZYMES SHARE SECONDARY STRUCTURE
- GLYCOSYLTRANSFERASES CAN ALSO BE GLYCOSYLATED
- LOCALIZATION OF GLYCOSYLTRANSFERASES IN GOLGI COMPARTMENTS
- PROTEOLYTIC CLEAVAGE AND SECRETION OF GOLGI GLYCOSYLTRANSFERASES
- TURNOVER AND RECYCLING OF GLYCANS
- NUCLEAR AND CYTOPLASMIC GLYCOSYLATION IS VERY COMMON
- GLYCOSYLATION REACTIONS AT THE PLASMA MEMBRANE
- GLYCOSYLATION IN UNEXPECTED SUBCELLULAR LOCATIONS
- FURTHER READING
- Review Cellular Organization of Glycosylation.[Essentials of Glycobiology. 2015]Review Cellular Organization of Glycosylation.Colley KJ, Varki A, Kinoshita T. Essentials of Glycobiology. 2015
- Review Cellular Organization of Glycosylation.[Essentials of Glycobiology. 2022]Review Cellular Organization of Glycosylation.Colley KJ, Varki A, Haltiwanger RS, Kinoshita T. Essentials of Glycobiology. 2022
- Eukaryotic glycosylation: online methods for site prediction on protein sequences.[Methods Mol Biol. 2015]Eukaryotic glycosylation: online methods for site prediction on protein sequences.Joshi HJ, Gupta R. Methods Mol Biol. 2015; 1273:127-37.
- [A theoretical analysis of the secondary structure and topology of dolichol-coupled enzymes].[Zh Evol Biokhim Fiziol. 1995][A theoretical analysis of the secondary structure and topology of dolichol-coupled enzymes].Shpakov AO. Zh Evol Biokhim Fiziol. 1995 Mar-Apr; 31(2):129-40.
- HMMpTM: improving transmembrane protein topology prediction using phosphorylation and glycosylation site prediction.[Biochim Biophys Acta. 2014]HMMpTM: improving transmembrane protein topology prediction using phosphorylation and glycosylation site prediction.Tsaousis GN, Bagos PG, Hamodrakas SJ. Biochim Biophys Acta. 2014 Feb; 1844(2):316-22. Epub 2013 Nov 10.
- Cellular Organization of Glycosylation - Essentials of GlycobiologyCellular Organization of Glycosylation - Essentials of Glycobiology
Your browsing activity is empty.
Activity recording is turned off.
See more...