NCBI Bookshelf. A service of the National Library of Medicine, National Institutes of Health.
Varki A, Cummings R, Esko J, et al., editors. Essentials of Glycobiology. Cold Spring Harbor (NY): Cold Spring Harbor Laboratory Press; 1999.
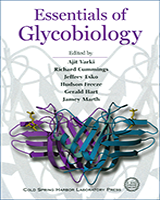
Essentials of Glycobiology.
Show detailsPrimary contributions to this chapter were made by J.D. Marth (HHMI, University of California at San Diego).
THE ONTOGENY OF MULTICELLULAR ORGANISMS and the activation of various cell types are associated with distinct changes in glycan structures. A vast amount of literature exists on this topic. This chapter provides examples of specific glycosylation changes occurring during development and physiologic responses with an emphasis on vertebrate systems, approaches used to detect these changes, and their potential functions.
Background
Glycosylation changes occurring on cell surfaces during mammalian embryogenesis and cell activation have been recognized for decades. Alterations in glycosylation have been proposed to participate in cell adhesion, receptor activation, cell differentiation, and tissue morphogenesis. Because the early embryo is composed of cells that must adhere to other embryonic cells, it seemed likely that factors modulating this adherence would have important roles in early development. Recognition that lectins promote various cell-cell adhesion events led to the speculation that cell surface glycans may be involved in vertebrate embryonic development. Indeed, studies with glycosylation inhibitors and exogenous glycans have resulted in substantial alterations in the adherence of embryonic cells with detrimental effects on embryonic development. Efforts to classify and determine the identities of these embryonic glycans began with the derivation of monoclonal antibodies against what were originally defined as “stage-specific” antigens. These antigenic structures were found to fluctuate at different times in embryogenesis. A surprising finding was that most of these monoclonal antibodies were carbohydrate-specific and recognized distinct glycan structures that wax and wane during vertebrate ontogeny. Since then, both lectins and anticarbohydrate antibodies have been used to derive information on vertebrate cell surface glycosylation in embryogenesis and cell activation. The biological relevance of these glycan modifications has remained mostly unresolved. However, in some cases, a direct role for certain glycans in vertebrate embryogenesis and cell activation has been obtained.
Fertilization (1–9)
The process of oocyte fertilization appears to require glycan recognition by lectins and has been explored in studies especially of the sea urchin, frog, and mouse. The sperm receptor on the sea urchin egg surface is composed of sulfated O-glycans which when exogenously added can inhibit fertilization. Additionally, sulfated glycans from sea urchin eggs can induce the sperm acrosome reaction needed for sperm fusion with the egg membrane. Species-specific glycosylation patterns are commonly found on vertebrate eggs. Sperm-egg binding in the frog Xenopus laevis may require glycan structures on the gp69/64 glycoproteins of the egg vitelline envelope. In the mouse, chemical removal of O-glycans has been shown to ablate sperm-egg binding, whereas removal of N-glycans had no effect. Addition of UDP-Gal and UDP-Fuc polymers also blocked sperm-egg binding. Although studies including the use of glycosidases indicated that the α1–3Gal glycan terminus was probably involved, α1–3Gal-deficient mice do not suffer from a deficiency of egg-sperm interaction or reduced fertility. Studies with β1–4 galactosyltransferase-deficient mice have suggested a function for this galactose linkage in the oocyte acrosome reaction that is induced by sperm binding during fertilization. More recent studies have suggested that α1–3-fucosylated O-glycans may be obligatory for high-affinity binding sperm. Additional studies will be needed to define which endogenous glycan structures are involved in mammalian fertilization.
Preimplantation Development (10–22)
Following sperm fertilization of an egg, the resulting one-cell embryo (or zygote) divides several times with no increase in size until the early embryo has completed travel through the oviduct (Fallopian tube) and entered the uterus, where it implants on the uterine epithelial wall. Vertebrate embryogenesis is often denoted by various stages of development. From fertilization until implantation is referred to as the preimplantation stage of development (Figure 34.1). This is when the first differentiative events occur, as cell division and embryo size constraints together produce daughter cells that have lost totipotency concurrent with cell-cell contacts that are now unique from parental cells. This is the morula stage of embryogenesis. Such embryos have approximately 16 cells, at which time they undergo the process of compaction, which is also the first morphologic event in embryonic development. Subsequently, the last stage of preimplantation development yields the blastocyst with approximately 64 cells. Embryos of this stage include an inner cell mass that will become the embryo itself, whereas the outer layer of trophoblast cells will ultimately form the placenta after implantation.

Figure 34.1
Preimplantation development in the mouse. (Reprinted, with permission, from [95] Hogan et al. 1994.)
It is during the preimplantation phase that the embryonic genome becomes active and produces the first embryonically derived proteins. This process actually begins early for some genes with transcriptional activation at the two-cell embryo stage. In studies referring to the role of genes and proteins during preimplantation development, it is necessary to consider the possibility that maternally (oocyte)-derived mRNAs and proteins may aid in development prior to induction of the embryonic genome. Such maternal sources may persist in preimplantation, but they are eventually lost by degradation and dilution.
Production of monoclonal antibodies generated against preimplantation embryonic cells yielded the first defined stage-specific embryonic antigens (SSEAs). SSEAs are glycan structures that may exist on glycoproteins or glycolipids. Their expression changes significantly in early embryogenesis (Figure 34.2). SSEA-1 is another term for what is also called the Lewis X oligosaccharide (Chapter 16) and is transiently found on 8-cell embryos but is lost by the 32–64-cell developmental stage. Absence of SSEA-1 correlates with the onset of compaction. These glycans may be functionally important, since synthetic multivalent Lewis-X-bearing haptens (attached to primary amino groups of lysyl-lysine) can decompact embryos (Figure 34.3). This was not observed with haptens bearing the Lewis A oligosaccharide or free Lewis X oligosaccharide added with free lysyl-lysine. In another study, glycans bearing fucose-linked α1–3 and α1–4 to GalNAc also caused decompaction. When mouse preimplantation embryos are further examined using antibodies that react with glycolipids, it is apparent that most glycolipids are of the globo series (containing Galα1–4Galβ1–4Glcβ1-ceramide) which includes SSEA-3, SSEA-4, and the Forssman antigen. SSEA-3 and SSEA-4 are found on membranes of early oocytes and zygotes, indicating that they are synthesized by maternal sources. Their expression is lost by the blastocyst stage. The Forssman antigen is found by the 8-cell stage and is maintained on cells of the inner cell mass of blastocysts. The function of these carbohydrate structures in embryogenesis is not known.

Figure 34.2
Carbohydrate variation in mouse preimplantation embryogenesis. (Modified, with permission, from [14] Fenderson et al. 1990.)
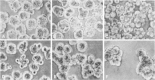
Figure 34.3
Adhesion of preimplantation fibroblasts regulated by multivalent oligosaccharides. (A) Compacted embryos treated with multivalent Lewis A (B) or multivalent SSEA-1/Lewis X (C) oligosaccharide. (D) Untreated embryos compared with those treated with fibroblast (more...)
Compaction (10–22)
The process of compaction can also be regulated by embryonic glycoconjugates bearing polylactosamines, sometimes termed embryoglycans. Natural human antibodies and distinct structural features define two types of polylactosamines: Those of the i type are unbranched and found to be widespread in expression from the zygote stage onward. In contrast, the branched I-type polylactosamines are absent from or expressed at low levels in preimplantation embryos (and see Chapter 16). Enzymatic removal of polylactosamines from embryos results in a greatly reduced rate of compaction, whereas addition of embryoglycans derived from F9 cells, but not from adult fibroblasts, hyperagglutinates 8–16-cell embryos (Figure 34.3). The antibody ECMA-2 is known to recognize a terminal α-linked galactose residue on polylactosamines of embryoglycans. ECMA-2 expression is widespread in preimplantation but progressively disappears by embryonic day (E)9–10 to become restricted to only a few subsets of adult tissues. The function of the ECMA-2 epitope in embryogenesis is not known.
Various glycosylation inhibitors have dramatic effects on preimplantation development. Tunicamycin blocks compaction and blastocyst formation but does not affect early cleavages. Continuous embryo exposure to tunicamycin kills trophoblast cells, but apparently not cells of the inner cell mass (Figure 34.4). This effect is similar to results obtained in embryos lacking the gene encoding GlcNAc-1-phosphotransferase, which is essential for the production of the dolichol oligosaccharide precursor needed in N-glycan biosynthesis; its absence ablates all embryonic N-glycosylation (see Chapter 7). In another study of N-glycosylation during preimplantation, compactin (an inhibitor of polyisoprenoid synthesis) was found to cause developmental arrest; however, this is not reversed by dolichol supplementation. Microenzymatic assays of enzymes involved in N-glycan biosynthesis, including Dol-P-Man synthetase, Dol-P-Glc synthetase, and Dol-P-P-chitobiosyl synthetase, indicate that these steadily decrease in activity from fertilization onward until they are induced at the blastocyst stage.

Figure 34.4
Effect of tunicamycin on blastocyst embryos in culture. Embryo outgrowths at equivalent embryonic day (EED) 7 (a,c,e) and 8 (b,d,f) are shown untreated (a,b) or treated with tunicamycin at 0.05 μg/ml (c,d) or 0.5 μg/ml (e,f). (Reprinted, (more...)
Postimplantation Embryogenesis (10,12,14,16,18,20,23–26)
Following implantation of the blastocyst-stage embryo on receptive uterine epithelium, various morphogenic processes occur in further development. In implantation and subsequently, the trophoblast cells act to interface the embryo with the maternal uterine epithelium and will ultimately form the placenta. Increased levels of polylactosamines are associated with implantation among cells involved in adhesion at the maternal-fetal interface, as well as with metastatic activity in various cancers (see Chapter 35). Gastrulation results in the formation of the mesoderm, thereby yielding the presence of all three embryonic germ layers: the endoderm, mesoderm, and ectoderm. This occurs during E7 in the mouse. Various glycan expression changes have been observed in early and late postimplantation embryos using lectins and anticarbohydrate antibodies (Figure 34.5). As in preimplantation, i-antigen expression remains widespread in the endoderm, ectoderm, and mesoderm. Sialylation of i-antigen structures, yielding sialyl-i, is also widespread. However, I-type polylactosamines are induced from gastrulation on and are more restricted in expression, with high levels found in the endoderm and mesoderm but very little expression noted in the ectoderm. Sialyl-I structures are present in the extraembryonic ectoderm. SSEA-3 and SSEA-4 reappear on the endoderm in early postimplantation development from E5 to E7, especially on the extraembryonic endoderm, which is involved in absorption, secretion, and formation of the placenta. The Forssman antigen is found on the primitive ectoderm from E5 to E10. The primitive ectoderm bears pluripotent stem cells that are derived from the inner cell mass.

Figure 34.5
Various changes in glycan structure throughout mouse gestation. (Reprinted, with permission, from [16] Muramatsu 1988 [© Wiley-Liss, Inc.].)
Most cell surface N-glycans on adult cells are of the complex subtype and therefore require the glycosyltransferase GlcNAcT-I. Embryonic induction of Mgat1-gene-encoded GlcNAcT-I has been shown to occur between E7 and E9 (Figure 34.6). Mice engineered to be deficient in GlcNAcT-I by inactivation of the Mgat1 gene die during E9, suggesting that embryonic Mgat1 gene induction occurs approximately at the time the embryo requires GlcNAcT-I function in various cell types. In the absence of GlcNAcT-I, morphogenic processes are affected including aberrant heart development (situs inversus), abnormal neural tube formation, and impaired vascularization. Prior to E9, it appears that the Mgat1-null embryo can acquire complex N-glycans from multiple parental sources including maternal RNA and glycoproteins with complex N-glycans, the latter of which are transported through the extraembryonic endoderm (the primitive placenta). These maternal sources may moderate the Mgat1-null phenotype and permit development to E9. Use of ConA and E-PHA to visualize N-glycans in embryos indicates no obvious distinctions among cell types of the three germ layers at E7 in the mouse, as all appear to react with these lectins (Figure 34.6). However, a subtype of complex N-glycans defined by L-PHA reactivity, and which requires GlcNAcT-V, is absent from E7 mesoderm and ectoderm (Figure 34.7).

Figure 34.6
Induction of GlcNAc-TI-encoding Mgat1 RNA, necessary for hybrid and complex N-glycan biosynthesis, in early postimplantation development of the mouse. Expression is shown in embryos at day 7 (a,b,c) or 9.5 (d,e) of development. A control experiment ( (more...)
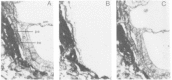
Figure 34.7
E-PHA (A), L-PHA (B), and ConA (C)-binding profiles on the three embryonic germ layers from E7 mouse embryos. Distinct absence of GlcNAc-TV branched N-glycans from the mesoderm (m), amnion (am), and neural ectoderm (ne) is noted. Embryonic endoderm (ee) (more...)
Cell movements during gastrulation and neural crest migration (see below) correlate with spatial changes in the composition of the ECM. Throughout the embryo, various pathways exist along which these molecules accumulate. The ECM is rich in hyaluronan and various proteoglycans, and these molecules may act to promote trafficking and differentiative events in this cytokine-rich milieu. Proteoglycans have been shown to accumulate at areas of major morphogenic developments, including organ and limb bud formation, and they can regulate the development of cell lineages and organs (see below). Relatively few reagents are presently available to detect changes in glycoaminoglycan structure in situ. However, proteoglycan sulfation detected with antibodies appears to be regulated in various spatial-temporal patterns including epithelial-mesenchymal cell boundaries and in regions of the developing brain.
Neurogenesis (27–51)
The finding that some monoclonal antibodies were specific for glycans restricted to neural cell subtypes or regions of the CNS suggested that carbohydrates might control some processes in neurogenesis. The SSEA-1 epitope expressed in early embryogenesis is reexpressed at later embryonic stages in a subpopulation of astrocytes within the cerebellum and spinal sensory neurons. Various lectins also detect changes in glycans in the developing nervous system. Early studies reported a ventral-to-dorsal gradient of GM2 ganglioside binding in the retinal-tectum system of vertebrates. Enzymatic activity that converts GM2 to GM1 by addition of a terminal galactose residue was found in a ventral-to-dorsal gradient, consistent with the above observation. Adhesion of neural cells to immobilized gangliosides was reported in vitro. Protease treatment of cells ablated this binding, suggesting the presence of cell surface lectins. In fact, an early study reported that chick retinal cell surfaces actually express an N-acetylgalactosaminyltransferase. This was a surprising finding since sugar donors needed for glycosylation are generally thought to be absent or at very low concentrations in the extracellular environment. Nevertheless, numerous observations of glycosyltransferases and glycosidases at the cell surface have led to the hypothesis that these enzymes may act as lectins in some situations. In the retino-tectum system, treatment of cells with N-acetylhexosaminidase or sialidase also decreased cell adhesion in the ventral region. Sialylation may thus also have a role. ST6Gal-I RNA has been reported in the rat retina with high expression in ganglion cells and various expression patterns among photoreceptor cells.
The JONES antigen is recognized by a monoclonal antibody specific for a 9-O-acetylated sialic acid on the ganglioside GD3. O-acetylated GD3 is observed on some neuroblasts and axons and is enriched on glial processes in regions through which axons migrate. In the retinal system, expression of O-acetylated GD3 is also found in a dorsal-to-ventral gradient and is thus opposite to the GM2 ganglioside binding described above. This is also distinct from the expression of GD3 itself, which does not show regional specificity in the retino-tectum system. Reactivity to the JONES epitope is highest in neural regions at times of maximal cell migration. Other studies of the developing retina revealed that removal of highly sulfated proteoglycans altered retinal histogenesis. These changes imply the possibility that glycan structures may modulate cell and axon guidance during neurogenesis (and see below).
A sulfated glycan structure termed HNK-1 (which refers to the monoclonal antibody) is found on neural crest migratory cells of the neural epithelium and other cells of neuroectodermal origin during postimplantation development. It is carried on both glycolipids and glycoproteins including N-CAM, myelin-associated glycoprotein, and tenascin. The biological role of this modification is unclear, although antibodies against HNK-1 have been reported to inhibit neuron-astrocyte, astrocyte-astrocyte, neuron-oligodendrocyte, and oligodendrocyte-oligodendrocyte cell adhesion.
Glycan involvement in the development of the synaptic junction has been proposed. The neuromuscular junction binds selectively to the lectin DBA, indicating the presence of terminal GalNAc residues. Other lectins specific for different saccharides bind synaptic and extrasynaptic regions specifically. The molecules bearing terminal GalNAc moieties have been reported to include the enzyme acetylcholinesterase and a glycolipid recognized by anti-SSEA-3 antibodies. The muscular sodium channel is found in the vertebrate neuromuscular junction. Sialic acid linkages and their abundance on various ion channels have been studied for more than a decade. Sialidase treatment has indicated that ion channel function assessed by current flow and the voltage dependence of gating are modulated by the presence of sialic acids. Ion channels in the myocardium, neuromuscular junction, and various neural cell types may all be regulated by electrostatic or steric mechanisms dependent on the number and positioning of sialic acid linkages. Some of these channels have been reported to contain oligosialic or polysialic acid.
Perhaps the best studied of glycan structures regulated in the vertebrate nervous system is that of PSA. PSA is a polymer of α2–8-linked sialic acid with chains commonly extending 60–100 residues (see Chapter 15). N-CAM carries the majority of PSA found in embryonic vertebrate tissues. PSA expression is highly restricted and prominent in the developing CNS (Figure 34.8). Most PSA is found on N-glycans of N-CAM. The expression of PSA is highly regulated and is found specifically on the embryonic form of N-CAM and correlates with absence of homotypic N-CAM adhesion. A role for PSA in inhibiting homotypic cell-cell adhesion is indicated. Enzymatic removal of PSA using a phage endoneuraminidase specific for α2–8-linked sialic acid yields evidence that PSA functions in neurite fasciculation (Figure 34.9). PSA has been proposed to regulate axon fasciculation by large steric and negative charge properties. Removal of PSA by neuraminidase injection into chick eyes perturbs genesis of the neural epithelium with a resulting ectopic optic nerve axon fiber layer in the retina. Interestingly, mice engineered with mutations in N-CAM by gene-targeting approaches led to a decreased size of the olfactory bulb with axon accumulation in the ventricular zone and deficits in spatial learning (Figure 34.9). Sialyltransferases involved in the production of PSA have been tentatively identified by in vitro cotransfection studies also using N-CAM cDNAs. At least five sialyltransferases may generate α2–8 sialic acid linkages; however, the STX sialyltransferase (ST8Sia-II) and PST (ST8Sia-IV) are likely responsible for PSA production on N-CAM, and their expression is correlated with the presence of this modification.

Figure 34.8
Expression of PSA is enriched in neural tissue during postimplantation development in the mouse. (Adapted, with permission, from [40] Ong et al. 1998 [© Oxford University Press].)

Figure 34.9
Neuronal fasciculation and olfactory bulb innervation are affected by loss of PSA. Neuronal trajectory of motor axons through hindlimb in sham-treated (A) and treated with α2–8 linked sialic-acid-specific neuraminidase (B). In comparison (more...)
Organogenesis (52–65)
Organogenesis reflects major morphogenic events in tissue pattern formation and limb development. Alterations of these events by modulators of glycan formation can yield clues to glycan roles in embryogenesis. Many such studies have been reported in vertebrate systems, including the mouse. In general, among vertebrates studied, a correlation exists between organogenesis and the increased production of ECM components, including hyaluronan and glycosaminoglycans. Hyaluronan production is high in the developing mouse limb buds, heart, and somites at critical stages in morphogenesis. This ECM component is bound by the hyaluronan receptor CD44, which exists as numerous isoforms from alternate RNA splicing. Chitin oligosaccharide synthetase activity has been discovered in zebrafish embryos during late gastrulation. Injection of antibodies against the DG42 hyaluronan synthase controlling production of this glycan leads to defects in morphogenesis involving trunk and limb development. The biosynthesis of proteoglycans in the developing mouse cornea undergoes a switch from heparan sulfate to keratan sulfate, the latter becoming a major consitutent of the cornea, where it may have a role in corneal transparency. In addition, sulfation patterns on proteoglycans have been found to change during vertebrate organogenesis and cartilage formation. Experiments with lectins, including wheat-germ agglutinin, when applied to the developing chick tail bud, resulted in a range of defects. During organogenesis, PSA has also been observed on N-CAM in a variety of mesodermal and endodermal derivatives. In general, PSA–N-CAM expression is prominent on epithelial cells during epithelium formation and decreases afterward.
The sulfated GalNAc found on a restricted subset of N-glycans controls the half-life of glycoprotein hormones (see Chapter 16). Production of this unique N-glycan appears to modulate endocrine functions and requires a GalNAc transferase specific for glycoprotein hormones and a sulfotransferase specific for terminal-β-linked GalNAc. These two enzymes and the resulting product are coordinately expressed in a subset of organs and their derivatives, including pituitary, submaxillary, and parotid glands and the kidney. In other examples, development of the rat pancreas in embryogenesis has been found to occur with specific changes in lectin reactivity regarding the cytodifferentiation of the three cell types (acinar, endocrine, and centroacinar). The plant lectins RCA II (Ricinus communis agglutinin) and SBA do not bind endocrine or centroacinar cells at any developmental stage, whereas RCA I and WGA bind to all three cell types. This lack of binding appears to be due to masking by sialic acid, as neuraminidase treatment results in exposure of binding epitopes to these two lectins. PSA–N-CAM has also been reported on cells of the developing pancreas. Myocytes in the developing heart preferentially bind the anti-GD antibody specific for Siaα2–3Galβ1–4GlcNAc. During cardiac innervation and morphogenesis, PSA-containing N-CAM is found on various axons and cells contributing to the development of the ventricular septal wall.
Many different glycan changes have been described in the developing lung. These include the increased biosynthesis of proteoglycans and the presence of a sulfated N-glycans. Lewis antigens change dramatically during lung development. In the earliest phase of lung bud development, the Ley antigen is expressed, but Lewis X and sialyl Lewis X are absent. Subsequently, epithelial cells of forming future bronchioles preferentially express Lewis X. Later, sialyl Lewis X is found to be primarily expressed on terminal bud cells, but Lewis X and Ley are absent. Ciliation of epithelial cells occurred with the reappearance of Lewis X and Ley expression. In adult lung, neither of the Lewis antigens is found at appreciable levels on respiratory cells of the lung.
Kidney development is associated with various changes in glycan structure. Two examples include fucosylated polylactosamine and PSA–N-CAM. Antibodies against the fucosylated polylactosamine bind to cells of the ampullae of the ingrowing ureteric branches but not the remainder of the ureteric bud. This glycan is absent following fusion of the ureteric bud with the S-shaped tubule but persists on segments of the convoluted tubule. A correlation is therefore suggested between expression of this fucosylated polylactosamine and cells involved in renal tubulogenesis. PSA expression is also reported in the developing kidney. At early stages, PSA–N-CAM was localized to the ureteric bud and the metanephrogenic mesenchyme. Expression in the ureteric bud declined and was absent prior to birth. PSA–N-CAM antibody reactivity was highest during mesenchymal transformation into epithelium. A correlation is thereby indicated between PSA–N-CAM expression and changes in cell adhesion during kidney organogenesis.
Hematopoiesis and the Immune System (66–74)
Cells of the hematopoietic lineage are relatively well defined with regard to their differentiation into various lineages (Figure 34.10). In ontogeny, hematopoietic stem cells first appear in the embryonic yolk sac at E7 in the mouse and consist only of nucleated red blood cells and macrophages. They are subsequently found in the developing liver of the mammalian embryo beginning at about E10. Liver-based hematopoiesis produces mature anuclear erythrocytes and continues to birth when the majority of hematopoietic stem cells have migrated to the bone marrow, where they then reside throughout adult life.

Figure 34.10
Cell lineage production during hematopoiesis. (CFU-GEMM CFU-mix) Colony-forming unit–granulocyte, erythrocyte, megakaryocyte, monocyte (multiple progenitor cells); (BFU-E) burst-forming unit–erythrocyte (early erythroid progenitor cell); (more...)
Developmental changes in glycan structures have been described on the erythroid, myeloid, and lymphoid lineages. A striking example involves the production of erythroid polylactosamine. In immature and fetal red blood cells of mouse and humans, the majority of polylactosamine is of the unbranched i type and is mostly present on glycolipids. This type of polylactosamine is also observed in periods of hemodynamic stress associated with a high production and turnover of red blood cells. In postnatal development, i antigen decreases significantly, and levels of branched I-type polylactosamines become the predominant species. The human adult erythroid I antigen is found at high levels on the Band-3 anion transporter, although mouse Band-3 may not carry this structure. Alterations of erythroid N-glycans are directly indicated in the etiology of dyserythropoiesis, with resulting high levels of i antigen on glycolipids. In comparison, granulocyte polylactosamines are normally and predominantly of the i type and may be fucosylated to generate the Lewis X antigen. This fucosylation occurs after the myeloblast stage of differentiation, and increased levels of Lewis X are noted with further maturation. The ABH blood group antigens are not found on human myeloid cells at any stage of differentiation. In general, erythroid cells are enriched in blood group antigens, lymphoid cells in Siaα2–6 termini on N-glycans, and myeloid cells in polylactosamines and sialyl Lewis X. The sialyl Lewis X oligosaccharide is detectable on immature and developing avian B and T lymphocytes, however, but is then absent from differentiated cells. Sialyl Lewis X reappears on mature neutrophils and monocytes, consistent with its role in leukocyte cell adhesion and trafficking (see Chapter 26).
Lymphocytes and accessory cell types exhibit specific cell surface glycans. Chondroitin sulfate makes up the majority of glycosaminoglycan structures on proteoglycans of immature B cells, whereas more mature B cells commonly synthesize heparan sulfate. Chondroitin proteoglycan sulfation on B lymphocytes is mostly at the 6-position, with much less 4-O-sulfation. T lymphocytes undergo a series of specific changes in glycosylation during their development in the thymus. This development requires interactions with thymic epithelial and dendritic cells. These accessory cells provide stimuli that regulate positive and negative selection—necessary events to acquire a mature peripheral T-cell repertoire that is both immune-competent and self-tolerant. Hyaluronic acid is produced at high levels by thymic epithelium, whereas thymocytes produce much less in comparison. The ratio is similar to the proportion of sulfated glycosaminoglycans produced by thymic epithelium and thymocytes. Among sulfated glycosaminoglycans, both thymic epithelium and thymocytes predominantly synthesize chondroitin sulfates and not heparan sulfates. Chondroitin sulfates of glycosaminoglycans on T lymphocytes are predominantly modified with 4-O-sulfate and not with 6-O-sulfate. Changes in thymocyte O-glycans have also been reported. Core 2 GlcNAcT expression is found in CD4+CD8+ cortical thymocytes but is absent from the single positive medullary thymocytes, indicating that most O-glycans in immature thymocytes are of the Core 1 subtype.
Sialylation of developing thymocytes is developmentally restricted. Binding of the plant lectin SNA, which requires ST6Gal-I function, is found primarily on mature medullary thymocytes, even though RNA in situ studies have shown ST6Gal-I expression is widespread and includes immature cortical thymocytes. One of the most documented changes in thymocyte oligosaccharide variation involves the lectin peanut agglutinin and the ST3Gal-I sialyltransferase. Immature cortical thymocytes highly express the peanut-agglutinin-binding structure Galβ1–3GalNAcα-Ser/Thr. This correlates with high levels of ST3Gal-I RNA in this immature T-cell population. Absence of peanut-agglutinin reactivity is noted in mature medullary thymic T cells, and this change is abrogated in the genetic absence of ST3Gal-I function (Figure 34.11; see Chapter 23).

Figure 34.11
PNA lectin reactivity (Galβ1–3GalNAcα-Ser/Thr) defines the cortical-medullary boundary in wild-type thymus tissue. PNA binding is high on immature cortical thymocytes and absent from mature medullary thymic T cells. This difference (more...)
Cell Activation and Signal Transduction (75–97)
Investigating glycan function in cell activation and signal transduction is an active research area. The observed changes in glycan production in ontogeny might be expected to occur in part through regulated variations in glycosyltransferase and glycosidase gene expression. Relatively little is known about the mechanisms by which glycosyltransferase and glycosidase genes are transcriptionally regulated. However, genetic loci encoding GlcNAcT-II and GlcNAcT-V are regulated by promoter sequences bearing sites for various transcription factors and growth-responsive elements, some of which either are defined as proto-oncogenes or are regulated by proto-oncogenes. This includes the ets-responsive elements in the GlcNAcT-V promoter, activated by the cellular src oncoprotein. In addition, liver transcription factors including HNF-1, DBP, and LAP have been found to trans-activate the ST6Gal-I promoter. Control of glycan alterations may be found to occur in a cell-cycle-specific manner. Production of the Lewis X determinant by fucosylation was found to be induced upon postmitotic intestinal villus-associated enterocytes that have reentered the cell cycle from exposure to the SV40 large T antigen. Glycosylation variants of human thyroid-stimulating hormone have unique physiological properties that have been shown to selectively activate signal transduction through either the cAMP or inositol phospholipid hydroylsis pathways.
The majority of studies involving glycans in cell activation and signaling have focused on hematopoietic cells because they are easily obtained, can generally be maintained in culture, and can be activated in vitro. Many examples exist where lymphocyte immune activation can be modified by changes in cell surface glycans and can be found in association with changes in endogenous oligosaccharide production. Evidence that lymphocyte signal transduction can be modulated by glycans was gathered in experiments that involved desialylation by sialidase, followed by activation of antigen receptors by cross-linking or by a mixed lymphocyte reaction. A significant increase in lymphocyte proliferation was obtained, suggesting that sialic acids may normally act to dampen immunologic responsiveness. T-cell activation has also been reported to occur with changes in O-glycosylation involving an increase in Core 2 GlcNAcT and Core 2 O-glycans. Additionally, lymphocyte proliferation can be modified by exogenously added proteoglycans, and lymphocyte stimulation itself induces a significant increase in proteoglycan production, especially involving 6-O-sulfated chondroitin. In some systems, tumor cell recognition by T cells leading to T-cell activation appeared to require sialic acids, perhaps of the α2–6-linked variety found mostly on N-glycans, since inhibitors of N-glycan biosynthesis had the same effect. Similar addition of deoxymannojirimycin or swainsonine to inhibit N-glycan formation in B lymphocytes was found to reduce levels of immunoglobulin production upon stimulation with the polyclonal activator pokeweed mitogen.
The sialyl Lewis X oligosaccharide has been studied for effects on leukocytes following binding to the L-selectin. Following treatment of leukocytes with the L-selectin ligand GlyCAM-1, or antibody-mediated cross-linked L-selectin, integrin activation has been observed with increased cell binding to fibronectin. Studies indicate that L-selectin binding to GlyCAM-1 promotes integrin activation, which leads to enhanced recruitment of leukocytes to peripheral lymph nodes. The nature of this integrin-dependent activation mechanism is not fully established, but it appears to include intracellular protein phosphorylation cascades.
Not all effects on vertebrate cell activation appear to be associated with changes among N- or O-glycans. Alterations in cell differentiation have been found to occur with exposure to specific glycolipids. For example, addition of GM3 ganglioside to cultures of the promonocytic HL60 cell line induced differentiation into monocytes, whereas a ganglioside mixture derived from neutrophils induced granulocytic differentiation. In another in vitro differentiation system, the GQ1b ganglioside has been shown to induce neurite outgrowth in some human neuroblastomas. Other glycolipids are able to modulate the activities of receptors for PDEF and EGF, although the mechanism is not understood. Apoptosis is an important event in the development and maintenance of the immune system in vertebrates. Gangliosides may be involved in apoptosis, as overexpression of the GD3 synthase gene induces apoptosis by mediating CD95 (Fas) activation in hematopoietic cells.
A mechanism by which glycans can control the activation and signal transduction of a cell surface receptor has been suggested in a case involving the glycosaminoglycan chains of proteoglycans. Invertebrate models of glycosaminoglycan sulfation deficiency yield severe developmental defects that involve the dysfunction of growth factor receptor signal transduction systems (see Chapter 11). It has long been known that many growth factors are sequestered by glycosaminoglycans following their biosynthesis and secretion. For example, heparan sulfate proteoglycans normally bind a variety of factors including the FGFs. Binding of FGF to glycosaminoglycans has been shown to be necessary for efficient FGF receptor dimerization, activation, and subsequently cell proliferation (Figure 34.12; see Chapter 29). FGF receptor signaling is known to be essential for the development of vertebrate embryos. During ontogeny, a switch in proteoglycan-binding specificity for FGF-1 over FGF-2 has been found to parallel the induction of FGF-1 RNA. Although most receptor tyrosine kinases require dimerization to activate their cytoplasmic tyrosine kinase catalytic domains, the mechanisms by which receptor dimerization occurs are generally unresolved but may involve glycan structure.

Figure 34.12
Glycosaminoglycan dependence on the activation of the FGF receptor. Glycosaminoglycan chains on proteoglycans bind to the FGF and the FGF receptor and are essential for efficient receptor activator with autophosphorylation (P).
Signal transduction events controlled by lectin-ligand interactions may occur between distinct cell surfaces or between molecules on the same cell surface. The CD22 lectin is found exclusively on B lymphocytes and specifically binds to the Siaα2–6Galβ1–4GlcNAc trisaccharide terminus of some N-glycans. It appears that lymphocytes in the mouse are especially enriched for this terminal trisaccharide moiety, including B lymphocytes themselves. CD22-binding activity has been shown to occur not only on the same cell surfaces, but also to CD22 itself as it also carries Siaα2–6Galβ1–4GlcNAc-R. The function of CD22 involves modulating B-lymphocyte immune activation, and thus modification of its lectin activity has been proposed as a mechanism of action. The ST6Gal-I sialyltransferase is required for Siaα2–6Galβ1–4GlcNAc-R production and is also expressed in a tissue-specific manner with high levels in lymphocytes. ST6Gal-I-deficient mice have been generated and are reported to lack the Siaα2–6Galβ1–4GlcNAc-R (see Chapter 33). Although otherwise seemingly unaffected, they exhibit an immunodeficient phenotype with reduced circulating IgM levels and impaired B-lymphocyte function. B lymphocytes from ST6Gal-I-deficient mice are deficient in tyrosine phosphorylation following antigen receptor cross-linking. In B lymphocytes, antigen receptor-induced tyrosine phosphorylation is crucial for the recruitment and activation of various kinases and phosphatases that subsequently become active and transmit signals downstream which promote B-cell immune function.
Future Directions
The large degree of variation in glycan structures found to be associated with ontogeny and cell activation has engendered many hypotheses purporting the presence of glycan-dependent regulatory mechanisms. In some cases, the use of exogenous glycosylation inhibitors or glycosidases has provided compelling evidence that indeed indicates a modulatory role for glycans in cell-cell interactions, cell-migration patterns during development, and receptor activation responses. From research on glycosyltransferase- and glycosidase-deficient cell lines, and the emergence of genetic studies of intact animals, it has become apparent that glycans are important components in a variety of developmental and postnatal processes. Future research in this area will further discriminate between the provocative changes in glycans observed in vivo and those changes that are physiologically significant to the intact organism. It should be of interest and perhaps foretelling to compare variations in glycosylation changes among species, as well as those glycan expression profiles that are most closely conserved in evolution.
References
- 1.
- Bleil J D, Wassarman P M. Galactose at the nonreducing terminus of O-linked oligosaccharides of mouse egg zona pellucida glycoprotein ZP3 is essential for the glycoprotein's sperm receptor activity. Proc. Natl. Acad. Sci. 1988;85:6778–6782. [PMC free article: PMC282061] [PubMed: 2842789]
- 2.
- Florman H M, Wassarman P M. O-linked oligosaccharides of mouse egg ZP3 account for its sperm receptor activity. Cell. 1985;41:313–324. [PMC free article: PMC7133279] [PubMed: 2986849]
- 3.
- Kitazyume-Kawaguchi S, Inoue S, Inoue Y, Lennarz W J. Identification of sulfated oligosialic acid units in the O-linked glycan of the sea urchin egg receptor for sperm. Proc. Natl. Acad. Sci. 1997;94:3650–3655. [PMC free article: PMC20495] [PubMed: 9108032]
- 4.
- Shaper J H. Murine sperm-zona binding: An α3-fucosyl residue is required for the assembly of a high affinity sperm-binding ligand. Glycobiology. 1998;8:A51. [PubMed: 9442021]
- 5.
- Thall A D, Maly P, Lowe J B. Oocyte Galα 1,3Gal epitopes implicated in sperm adhesion to the zona pellucida glycoprotein ZP3 are not required for fertilization in the mouse. J. Biol. Chem. 1995;270:21437–21440. [PubMed: 7545161]
- 6.
- Tian J, Gong H, Thomsen G H, Lennarz W J. Gamete interactions in Xenopus laevis: Identification of sperm binding glycoproteins in the egg vitelline envelope. J. Cell. Biol. 1997;136:1099–1108. [PMC free article: PMC2132474] [PubMed: 9060474]
- 7.
- Vacquier V D. Evolution of gamete recognition proteins. Science. 1998;281:1995–1998. [PubMed: 9748153]
- 8.
- Vacquier V D, Moy G W. The fucose sulfate polymer of egg jelly binds to perm REJ and is the inducer of the sea urchin sperm acrosome reaction. Dev. Biol. 1997;192:125–135. [PubMed: 9405102]
- 9.
- Youakim A, Hathway H J, Miller D J, Gong X, Shur B D. Overexpressing sperm surface β 1,4-galactosyltransferase in transgenic mice affects multiple aspects of sperm-egg interactions. J. Cell Biol. 1994;126:1573–1583. [PMC free article: PMC2290943] [PubMed: 8089187]
- 10.
- Armant D R, Kaplan H A, Lennarz W J. N-linked glycoprotein biosynthesis in the developing mouse embryo. Dev. Biol. 1986;113:228–237. [PubMed: 2417901]
- 11.
- Atienza-Samols S B, Pine P R, Sherman M I. Effects of tunicamycin upon glycoprotein synthesis and development of early mouse embryos. Dev. Biol. 1980;79:19–32. [PubMed: 7409320]
- 12.
- Bourrillon R, Aubery M. Cell surface glycoproteins in embryonic development. Int. Rev. Cytol. 1989;116:257–338. [PubMed: 2670803]
- 13.
- Feizi T. Demonstration by monoclonal antibodies that carbohydrate structures of glycoproteins and glycolipids are onco-developmental antigens. Nature. 1985;314:53–57. [PubMed: 2579340]
- 14.
- Fenderson B A, Eddy E M, Hakomori S -I. Glycoconjugate expression during embryogenesis and its biological significance. BioEssays. 1990;12:173–179. [PubMed: 1970725]
- 15.
- Kannagi R, Cochran N A, Ishigami F, Hakomori S, Andrews P W, Knowles B B, Solter D. Stage-specific embryonic antigens (SSEA-3 and -4) are epitopes of a unique globo-series ganglioside isolated from human teratocarcinoma cells. EMBO J. 1983;2:2355–2361. [PMC free article: PMC555457] [PubMed: 6141938]
- 16.
- Muramatsu T. Developmentally regulated expression of cell surface carbohydrates during mouse embryogenesis. J. Cell. Biochem. 1988;36:1–14. [PubMed: 3277983]
- 17.
- Ozawa M, Muramatsu T, Solter D. Ssea-1, a Stage-specific Embryonic Antigen of the Mouse, is Carried by the Glycoprotein-bound Large Carbohydrate in Embryonal Carcinoma Cells. Cell. Differ. 1985;16:169–173. [PubMed: 2860973]
- 18.
- Pennington J E, Rastan S, Roelcke D, Feizi T. Saccharide structures of the mouse embryo during the first eight days of development. J. Embryol. Exp. Morphol. 1985;90:335–361. [PubMed: 3009679]
- 19.
- Rastan S, Thorpe S J, Scudder P, Brown S, Gooi H C, Feizi T. Cell interactions in preimplantation embryos: Evidence for involvement of saccharides of the poly-N-acetyllactosamine series. J. Embryol. Exp. Morphol. 1985;87:115–128. [PubMed: 3928796]
- 20.
- Sato M, Muramatsu T. Oncodevelopmental Carbohydrate Antigens: Distribution of Ecma 2 and 3 Antigens in Embryonic and Adult Tissues of the Mouse and in Teratocarcinomas. J. Reprod. Immunol. 1986;9:123–135. [PubMed: 2427714]
- 21.
- Solter D, Knowles B B. Monoclonal antibody defining a stage-specific mouse embryonic antigen (SSEA-1). Proc. Natl. Acad. Sci. 1978;75:5565–5569. [PMC free article: PMC393007] [PubMed: 281705]
- 22.
- Thorpe S J, Bellairs R, Feizi T. Developmental patterning of carbohydrate antigens during early embryogenesis of the chick: Expression of antigens of the poly-N-acetyllactosamine series. Development. 1988;102:193–210. [PubMed: 3046907]
- 23.
- Campbell R M, Metzler M, Granovsky M, Dennis J W, Marth J D. Complex asparagine-linked oligosaccharides in Mgat1-null embryos. Glycobiology. 1995;5:535–543. [PubMed: 8563140]
- 24.
- Granovsky M, Fode C, Warren C E, Campbell R M, Marth J D, Pierce M, Friegen N, Dennis J W. GlcNAcTransferase V and core 2 GlcNAcTransferase expression in the developing mouse embryo. Glycobiology. 1995;5:797–806. [PubMed: 8720078]
- 25.
- Mark M P, Baker J R, Kimata K, Ruch J V. Regulated changes in chondroitin sulfation during embryogenesis: An immunohistochemical approach. Int. J. Dev. Biol. 1990;34:191–204. [PubMed: 2118368]
- 26.
- Muramatsu T, Condamine H, Gachelin G, Jacob F. Changes in fucosly-glycopeptides during early post-impantation embryogenesis in the mouse. J. Embryol. Exp. Morphol. 1980;57:25–36. [PubMed: 7430933]
- 27.
- Bennett E, Urcan M S, Tinkle S S, Koszowski A G, Levinson S R. Contribution of sialic acid to the voltage dependence of sodium channel gating. A possible electrostatic mechanism. J. Gen. Physiol. 1997;109:327–343. [PMC free article: PMC2217074] [PubMed: 9089440]
- 28.
- Blum A S, Barnstable C J. O-acetylation of a cell-surface carbohydrate creates discrete molecular patterns during neural development. Proc. Natl. Acad. Sci. 1987;84:8716–8720. [PMC free article: PMC299617] [PubMed: 2446330]
- 29.
- Britis P A, Canning D R, Silver J. Chondroitin sulfate as a regulator of neuronal patterning in the retina. Science. 1992;255:733–736. [PubMed: 1738848]
- 30.
- Castillo C, Diaz M E, Balbi D, Thornhill W B, Recio-Pinto E. Changes in sodium channel function during postnatal brain development reflect increases in the level of channel sialidation. Brain Res. Dev. Brain Res. 1997;104:119–130. [PubMed: 9466714]
- 31.
- Cremer H, Lange R, Christoph A, Plomann M, Vopper G, Roes J, Brown R, Baldwin S, Kraemer P, Scheff S, Barthels D, Rajewsky K, Wille W. Inactivation of the N-CAM gene in mice results in size reduction of the olfactory bulb and deficits in spatial learning. Nature. 1994;367:455–459. [PubMed: 8107803]
- 32.
- Dasgupta S, Hogan E L, Spicer S S. Stage-specific expression of fuco-neolacto- (Lewis X) and ganglio-series neutral glycosphingolipids during brain development: Characterization of Lewis X and related glycosphingolipids in bovine, human and rat brain. Glycoconj. J. 1996;13:367–375. [PubMed: 8781967]
- 33.
- Doherty P, Cohen J, Walsh F S. Neurite outgrowth in response to transfected N-CAM changes during development and is modulated by polysialic acid. Neuron. 1990;5:209–219. [PubMed: 2200449]
- 34.
- Fermini B, Nathan R D. Removal of sialic acid alters both T- and L-type calcium currents in cardiac myocytes. Am. J. Physiol. 1991;260:H735–H743. [PubMed: 2000969]
- 35.
- Jessel T M, Hynes M A, Dodd J. Carbohydrates and carbohydrate-binding proteins in the nervous system. Annu. Rev. Neurosci. 1990;13:227–255. [PubMed: 2183675]
- 36.
- Key B, Akeson R A. Delineation of olfactory pathways in the frog nervous system by unique glycoconjugates and N-CAM glycoforms. Neuron. 1991;6:381–396. [PubMed: 2001285]
- 37.
- Kiss J Z, Rougon G. Cell biology of polysialic acid. Curr. Opin. Neurobiol. 1997;7:640–646. [PubMed: 9384537]
- 38.
- Landmesser L, Dahm L, Tang J, Rutishauser U. Polysialic acid as a regulator of intramuscular nerve branching during embryonic development. Neuron. 1990;4:655–667. [PubMed: 2344405]
- 39.
- McDonagh J C, Nathan R D. Sialic acid and the surface charge of delayed rectifier potassium channels. J. Mol. Cell. Cardiol. 1990;22:1305–1316. [PubMed: 2283687]
- 40.
- Ong E, Nakayama J, Angata K, Reyes L, Katsuyama T, Arai Y, Fukuda M. Developmental regulation of polysialic acid synthesis in mouse directed by two polysialyltransferases, PST and STX. Glycobiology. 1998;8:415–424. [PubMed: 9499389]
- 41.
- Ono K, Tomasiewicz H, Magnuson T, Rutishauser U. N-CAM mutation inhibits tangential neuronal migration and is phenocopied by enzymatic removal of polysialic acid. Neuron. 1994;13:595–609. [PubMed: 7917293]
- 42.
- Phillips G R, Krushel L A, Crossin K L. Developmental expression of two rat sialyltransferases that modify the neural cell adhesion molecule, N-CAM. Brain Res. Dev. Brain Res. 1997;102:143–155. [PubMed: 9352097]
- 43.
- Recio-Pinto E, Thornhill W B, Duch D S, Levinson S R, Urban B W. Neuraminidase treatment modifies the function of electroplax sodium channels in planar lipid bilayers. Neuron. 1990;5:675–684. [PubMed: 2171591]
- 44.
- Rutishauser U. Polysialic acid and the regulation of cell interactions. Curr. Opin. Cell Biol. 1996;8:679–684. [PubMed: 8939657]
- 45.
- Schlosshauer B, Blum A S, Mendez-Otero R, Barnstable C J, Constantine-Paton M. Developmental Regulation of Ganglioside Antigens Recognized by the Jones Antibody. J. Neurosci. 1988;8:580–592. [PMC free article: PMC6569300] [PubMed: 2448435]
- 46.
- Sieber-Blum M. Ssea-1 is a Specific Marker for the Spinal Sensory Neuron Lineage in the Quail Embryo and in Neural Crest Cell Cultures. Dev. Biol. 1989;134:362–375. [PubMed: 2568298]
- 47.
- Tole S, Patterson P H. Regionalization of the Developing Forebrain: a Comparison of Forse-1, Dlx-2, and Bf-1. J. Neurosci. 1995;15:970–980. [PMC free article: PMC6577806] [PubMed: 7869122]
- 48.
- Walsh F S, Parekh R B, Moore S E, Dickson G, Dickson G, Barton C H, Gower H J, Dwek R A, Rademacher T W. Tissue specific O-linked glycosylation of the neural cell adhesion molecule (N-CAM). Development. 1989;105:803–811. [PubMed: 2598815]
- 49.
- Wilson D B, Wyatt D P. Patterns of lectin binding during mammalian neurogenesis. J. Anat. 1995;186:209–216. [PMC free article: PMC1167286] [PubMed: 7649814]
- 50.
- Yee H F Jr, Weiss N J, Langer G A. Neuraminidase selectively enhances transient Ca2+ current in cardiac myocytes. Am. J. Physiol. 1989;256:C1267–C1272. [PubMed: 2544097]
- 51.
- Zuber C, Lackie P M, Catterall W A, Roth J. Polysialic acid is associated with sodium channels and the neural cell adhesion molecule N-CAM in adult rat brain. J. Biol. Chem. 1992;267:9965–9971. [PubMed: 1315775]
- 52.
- Bakkers J, Semino C E, Stroband H, Kijne J W, Robbins P W, Spaink H P. An important developmental role for oligosaccharides during early embryogenesis of cyprinid fish. Proc. Natl. Acad. Sci. U. S. A. 1997;94:7982–7986. [PMC free article: PMC21541] [PubMed: 9223299]
- 53.
- Candelier J J, Mollicone R, Mennesson B, Bergemer A M, Henry S, Coullin P, Oriol R. α-3-fucosyltransferases and their glycoconjugate antigen products in the developing human kidney. Lab. Invest. 1993;69:449–459. [PubMed: 8231113]
- 54.
- Ertl C, Wrobel K H. Distribution of sugar residues in the bovine testis during postnatal ontogenesis demonstrated with lectin-horseradish peroxidase conjugates. Histochemistry. 1992;97:161–171. [PubMed: 1559848]
- 55.
- Fleming A. N-linked oligosaccharides during human renal organogenesis. J. Anat. 1990;170:151–160. [PMC free article: PMC1257071] [PubMed: 1701421]
- 56.
- Fleming S, Brown G. Distribution of fucosylated N-acetyl lactosamine carbohydrate determinants during embryogenesis of the kidney in man. Histochem. J. 1986;18:61–66. [PubMed: 2426222]
- 57.
- Fukushi Y, Orikasa S, Shepard T, Hakomori S. Changes of Lex and dimeric Lex haptens and their sialylated antigens during development of human kidney and kidney tumors. J. Urol. 1986;135:1048–1056. [PubMed: 3007781]
- 58.
- Hart G W. Biosynthesis of glycosaminoglycans during corneal development. J. Biol. Chem. 1976;251:6513–6521. [PubMed: 988023]
- 59.
- Hooper L, Beranek M C, Manzella S M, Baenziger J U. Differential expression of GalNAc-4-sulfotransferase and GalNAc-transferase results in distinct glycoforms of carbonic anhydrase VI in parotid and submaxillary glands. J. Biol. Chem. 1995;270:5985–5993. [PubMed: 7890728]
- 60.
- Kitagawa H, Paulson J C. Differential expression of five sialyltransferase genes in human tissues. J. Biol. Chem. 1994;269:17872–17878. [PubMed: 8027041]
- 61.
- Lackie P M, Zuber C, Roth J. Polysialic acid and N-CAM localisation in embryonic rat kidney: Mesenchymal and epithelial elements show different patterns of expression. Development. 1990;110:933–947. [PubMed: 2088729]
- 62.
- Miyake M, Zenita K, Tanaka O, Okada Y, Kannagi R. Stage-specific Expression of Ssea-1-related Antigens in the Developing Lung of Human Embryos and Its Relation to the Distribution of These Antigens in Lung Cancers. Cancer Res. 1988;48:7150–7158. [PubMed: 2903794]
- 63.
- Serafini-Cessi F. Sialyltransferase activity in regenerating rat liver. Biochem. J. 1977;166:381–386. [PMC free article: PMC1165020] [PubMed: 597233]
- 64.
- Vertino-Bell A, Ren J, Black J D, Lau J T. Developmental regulation of β-galactoside α 2,6-sialyltransferase in small intestine epithelium. Dev. Biol. 1994;165:126–136. [PubMed: 8088431]
- 65.
- Wang X, O'Hanlon T P, Young R F, Lau J T. Rat beta-galactoside α 2,6-sialyltransferase genomic organization: Alternate promoters direct the synthesis of liver and kidney transcripts. Glycobiology. 1990;1:25–31. [PubMed: 1983783]
- 66.
- Baum L G, Derbin K, Perillo N L, Wu T, Pang M, Uittenbogaart C. Characterization of terminal sialic acid linkages on human thymocytes. Correlation between lectin-binding phenotype and sialyltransferase expression. J. Biol. Chem. 1996;271:10793–10799. [PubMed: 8631891]
- 67.
- Britz J S, Hart G W. Biosynthesis of glycosaminoglycans by epithelial and lymphocytic components of murine thymus. J. Immunol. 1983;130:1848–1855. [PubMed: 6601142]
- 68.
- Engelmann S, Ebeling O, Schwartz-Albiez R. Modulated glycosylation of proteoglycans during differentiation of human B lymphocytes. Biochim. Biophys. Acta. 1995;1267:6–14. [PubMed: 7779869]
- 69.
- Fukuda M., ed. 1992. Cell surface carbohydrates and cell development, pp. 127–159. CRC Press, Boca Raton.
- 70.
- Fukuda M, Fukuda M N. Changes in cell surface glycoproteins and carbohydrate structures during the development and differentiation of human erythroid cells. J. Supramol. Struct. Cell. Biochem. 1981;17:313–324. [PubMed: 6799656]
- 71.
- Gillespie W, Paulson J C, Kelm S. Regulation of α 2,3-sialyltransferase expression correlates with conversion of peanut agglutinin (PNA)+ to PNA– phenotype in developing thymocytes. J. Biol. Chem. 1993;268:3801–3804. [PubMed: 8440675]
- 72.
- Palojoki E, Jalkanen S, Toivanen P. Sialyl Lewis X carbohydrate is expressed differentially during avian lymphoid cell development. Eur. J. Immunol. 1995;25:2544–2550. [PubMed: 7589124]
- 73.
- Rider C C, Hart G W. Differential sulphation of chondroitins in murine T and B lymphocytes and lymphoma cells. Mol. Immunol. 1987;24:963–967. [PubMed: 3116414]
- 74.
- Tulp A, Barnhoorn M, Bause E, Ploegh H. Inhibition of N-linked oligosaccharide trimming mannosidases blocks human B cell development. EMBO J. 1986;5:1783–1790. [PMC free article: PMC1167041] [PubMed: 2944744]
- 75.
- Bessler H, Singer R, Raanani P, Levinsky H, Lahav M, Cohen A M. Interferon α-2b modulates β-galactoside α-2,6 sialyltransferase gene expression in rat testes. Biol. Reprod. 1995;53:1474–1477. [PubMed: 8562705]
- 76.
- Bry L, Falk P G, Gordon J L. Genetic engineering of carbohydrate biosynthetic pathways in transgenic mice demonstrates cell cycle-associated regulation of glycoconjugate production in small intestinal epithelial cells. Proc. Natl. Acad. Sci. 1996;93:1161–1166. [PMC free article: PMC40049] [PubMed: 8577733]
- 77.
- Coughlan C M, Breen K C. Glucocorticoid induction of the α 2,6 sialyltransferase enzyme in a mouse neural cell line. J. Neurosci. Res. 1998;51:619–626. [PubMed: 9512006]
- 78.
- Coughlan C M, Seckl J R, Fox D J, Unsworth R, Breen K C. Tissue-specific regulation of sialyltransferase activities in the rat by corticosteroids in vivo. Glycobiology. 1996;6:15–22. [PubMed: 8991504]
- 79.
- De Maria R, Lenti L, Malisan F, d'Agostino F, Tomassini B, Zeuner A, Rippo M R, Testi R. Requirement for GD3 ganglioside in CD95- and ceramide-induced apoptosis. Science. 1997;277:1652–1655. [PubMed: 9287216]
- 80.
- Hanasaki K, Varki A, Stamenkovic I, Bevilacqua M P. Cytokine-induced β-galactoside α-2,6-sialyltransferase in human endothelial cells mediates α 2,6-sialylation of adhesion molecules and CD22 ligands. J. Biol. Chem. 1994;269:10637–10643. [PubMed: 8144653]
- 81.
- Hart G W. Biosynthesis of glycosaminoglycans by thymic lymphocytes. Effects of mitogenic activation. Biochemistry. 1982;21:6088–6096. [PubMed: 7150545]
- 82.
- Hennet T, Chui D, Paulson J C, Marth J D. Immune regulation by the ST6Gal sialyltransferase. Proc. Natl. Acad. Sci. 1998;95:4504–4509. [PMC free article: PMC22519] [PubMed: 9539767]
- 83.
- Kearse K P, Hart G W. Lymphocyte activation induces rapid changes in nuclear and cytoplasmic glycoproteins. Proc. Natl. Acad. Sci. 1991;88:1701–1705. [PMC free article: PMC51092] [PubMed: 2000378]
- 84.
- Nurcombe V, Ford M D, Wildschut J A, Bartlett P F. Developmental regulation of neural response to FGF-1 and FGF-2 by heparan sulfate proteoglycan. Science. 1993;260:103–106. [PubMed: 7682010]
- 85.
- Piller F, Piller V, Fox R I, Fukuda M. Human T-lymphocyte activation is associated with changes in O-glycan biosynthesis. J. Biol. Chem. 1988;263:15146–15150. [PubMed: 2971663]
- 86.
- Powell L D, Bause E, Legler G, Molyneux R J, Hart G W. Influence of asparagine-linked oligosaccahrides on tumor cell recognition in the mixed lymphocyte reaction. J. Immunol. 1985;135:714–724. [PubMed: 3158709]
- 87.
- Powell L D, Whiteheart S W, Hart G W. Cell surface sialic acid influences tumor cell recognition in the mixed lymphocyte reaction. J. Immunol. 1987;139:262–270. [PubMed: 2953814]
- 88.
- Schaaf L, Leiprecht A, Saji M. Glycosylation variants of human TSH selectively activate signal transduction pathways. Mol. Cell. Endocrinol. 1997;132:185–194. [PubMed: 9324060]
- 89.
- Sela B A, Wang J L, Edelman G M. Lymphocyte activation by monovalent fragments of antibodies reactive with cell surface carbohydrates. J. Exp. Med. 1976;143:665–671. [PMC free article: PMC2190129] [PubMed: 1249523]
- 90.
- Spivak-Kroizman T, Lemmon M A, Dikic I, Ladbury J E, Pinchasi D, Huang J, Jaye M, Crumley G, Schlessinger J, Lax I. Heparin-induced oligomerization of FGF molecules is responsible for FGF receptor dimerization, activation, and cell proliferation. Cell. 1994;79:1015–1024. [PubMed: 7528103]
- 91.
- Svensson E C, Conley P B, Paulson J C. Regulated expression of α 2,6-sialyltransferase by the liver-enriched transcription factors HNF-1, DBP, and LAP. J. Biol. Chem. 1992;267:3466–3472. [PubMed: 1737800]
- 92.
- Tsuboi S, Fukuda M. Branched O-linked oligosaccharides ectopically expressed in transgenic mice reduce primary T-cell immune responses. EMBO J. 1997;16:6364–6373. [PMC free article: PMC1170243] [PubMed: 9351819]
- 93.
- Wang X C, O'Hanlon T P, Lau J T. Regulation of β-galactoside α 2,6-sialyltransferase gene expression by dexamethasone. J. Biol. Chem. 1989;264:1854–1859. [PubMed: 2912988]
- 94.
- Wolf M F, Schmitt H R, Schumacher K. Expression of Thomsen-Friedenreich (TF) antigens on lymphocytes. II. Loss of cryptic TF antigens during mitogenic activation of human T and B lymphocytes. Cell. Immunol. 1989;121:366–371. [PubMed: 2786763]
- 95.
- Hogan B., Beddington R., Costantini F., and Lacy E. 1994. Manipulating the mouse embryo: A laboratory manual, 2nd editon. Cold Spring Harbor Laboratory Press, Cold Spring Harbor, New York.
- 96.
- Miltenyi Biotec. 1998. MACS&more, vol. 2, no. 2, pp. 3–4. Miltenyi Biotec, Bergisch Gladbach, Germany .
- 97.
- Fenderson B A, Zehavi U, Hakomori S. A multivalent lacto-N-fucopentaose III-lysyllysine conjugate decompacts preimplantation mouse embryos, while the free oligosaccharide is ineffective. J. Exp. Med. 1984;160:1591–1596. [PMC free article: PMC2187492] [PubMed: 6491606]
- Glycosylation Changes in Ontogeny and Cell Activation - Essentials of Glycobiolo...Glycosylation Changes in Ontogeny and Cell Activation - Essentials of Glycobiology
Your browsing activity is empty.
Activity recording is turned off.
See more...