NCBI Bookshelf. A service of the National Library of Medicine, National Institutes of Health.
Mucignat-Caretta C, editor. Neurobiology of Chemical Communication. Boca Raton (FL): CRC Press/Taylor & Francis; 2014.
10.1. INTRODUCTION
In most species, there are two chemosensory systems, both located in the nasal cavity but physiologically and anatomically distinct (Figure 10.1). The main olfactory epithelium (MOE) is principally involved in the airborne odor perception, whereas the vomeronasal organ (VNO) of Jacobson in the detection of pheromones that are chemical compounds secreted or excreted by individuals of the same species (conspecifics) (Dulac & Torello 2003; Tirindelli et al. 2009). Deficits in social and reproductive behavior are marked in animals underlying the surgical removal of the VNO but also the chemical inactivation of the MOE (Kiyokawa et al. 2007; Kolunie & Stern 1995; Leypold et al. 2002; Wysocki & Lepri 1991). Furthermore, there is evidence that two olfactory structures, namely the septal organ of Masera and the Grueneberg ganglion also contribute to pheromone detection (Ma 2007; Roppolo et al. 2006; Tirindelli et al. 2009) (Figure 10.1).
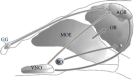
FIGURE 10.1
Chemosensory systems sensing pheromones. The main olfactory system (MOE) and the vomeronasal system (VNO) together with the septal organ of Masera (SO) and the Grueneberg ganglion (GG) are implicated in pheromone detection. Projections of sensory neurons (more...)
Primary sensory VNO neurons (VSNs) send axons to mitral cells in the glomerular region of the accessory olfactory bulb (AOB), whereas olfactory neurons (OSNs) to the mitral cells of the main olfactory bulb (MOB) (Baum & Kelliher 2009; Clapham & Neer 1997) (Figure 10.1). The chemosensory neurons of the VNO and MOE are equipped with specific receptors that activate distinct molecular cascades and mediate sociosexual behaviors (Del Punta et al. 2002; Stowers et al. 2002).
The anatomical components of the VNO firstly developed in a tetrapod ancestor and led to the appearance of a rudimentary structure in amphibians that became highly organized in Squamata and in many mammalian orders as Didelphimorphia, Rodentia, and in primates (prosimians and New World monkeys). In contrast, VNO is virtually absent in birds, bats, Old World monkeys, apes, and humans (Dennis et al. 2004; Grus et al. 2005; Shi & Zhang 2007; Smith et al. 2005; Zhao et al. 2011) (Figure 10.2a).
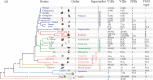
FIGURE 10.2
(a) The phylogenetic tree of mammals. Mammals comprise about 5400 species that are distributed in six superorders including Euarchontoglires (Rodentia, Lagomorpha, primates), Laurasiatheria (Artiodactyla, Carnivora, Perissodactyla, and Chiroptera), Xenarthra (more...)
In Didelphimorphia, Lagomorpha, and Rodentia, the VNO chemosensory epithelium presents two separate neuronal layers (apical and basal) characterized by the expression of different G protein subunits and receptors (Young & Trask 2007). Apical and basal neurons project to two distinct regions (anterior and posterior) of the AOB. From the AOB, the majority of the centripetal projections of the apical and basal neurons remain segregated in the amygdala and hypothalamus (Martinez-Marcos 2009; Yoon et al. 2005).
In other mammalian orders (Carnivora) the dichotomic organization of the vomeronasal projections is not well defined or even absent (Artiodactyla) (Dennis et al. 2003).
10.2. PHEROMONE RECEPTORS
The molecular organization of the rodent vomeronasal neuroepithelium in apical and basal neurons is based on the specific expression pattern of two transduction molecules, G protein Gαi2 and Gαo, and two distinct superfamilies of pheromone receptors, type-1 vomeronasal receptors (V1Rs) (Dulac & Axel 1995) and type-2 vomeronasal receptors (V2Rs) (Herrada & Dulac 1997; Matsunami & Buck 1997; Ryba & Tirindelli 1997).
Gαi2 is expressed in the apical neurons and colocalizes with V1Rs, whereas Gαo coexpresses with V2Rs in the basal neurons (Rodriguez et al. 2002).
Although both are G protein coupled receptors, V2Rs differ from V1Rs for the presence of introns in the genes and for the long N-terminal extracellular region that reflects the chemical properties of their ligands (Boschat et al. 2002; Emes et al. 2004; Ishii & Mombaerts 2008; Isogai et al. 2011; Kimoto et al. 2005). Furthermore, V1Rs and V2Rs display a differential evolutionary expansion in terrestrial vertebrates (Brykczynska et al. 2013; Dong et al. 2012; Grus et al. 2007; Liberles et al. 2009; Ryu et al. 2013; Yang & Shi 2010) (Figure 10.2a, b, and d).
However, although only V1Rs and V2Rs have been proved to respond to pheromones, the vomeronasal expression of a third class of putative pheromone receptors, formyl-peptide receptors (VNO-FPRs), was recently demonstrated (Boschat et al. 2002; Del Punta et al. 2002; Dulac & Torello 2003). FPRs are expressed in both the apical and basal regions of the VNOs of some murine rodents and may represent a link between the Gi and Go subsystems (Liberles et al. 2009; Riviere et al. 2009).
Additionally, basal VNO neurons express a family of nonclassical molecules of the major histocompatibility complex (H2-Mv) (Ishii & Mombaerts 2008; Leinders-Zufall et al. 2009; Loconto et al. 2003). The role of the H2-Mv complex in the VNO is still debated and whether these molecules are to be considered as pheromone receptors remains to be demonstrated (Ishii & Mombaerts 2008; Loconto et al. 2003).
10.2.1. V1Rs
V1Rs are already present in lower vertebrate (fish and frog) genomes (Date-Ito et al. 2008; Grus & Zhang 2009; Hashiguchi et al. 2008; Saraiva & Korsching 2007). In mammals, V1Rs expansion occurred independently from a small number of ancestral genes that pseudogenized or completely disappeared in the Squamata order (Young et al. 2010). Remarkable gene duplications occurred in the Rodentia order with the generation of several subfamilies (Grus & Zhang 2004; Rodriguez et al. 2002) (Figure 10.2b).
The V1R repertoire is characterized by a rapid gene turnover, significant variation of the size among species, positive selection, and lineage-specific clustering (Emes et al. 2004; Shi et al. 2005; Young et al. 2005).
V1R genes diverge among mammals following species-specific duplications that resulted in the formation of distinct clades with the occurrence of very few orthologs only present in closely related species (Park et al. 2011).
The high rate of duplication led to a large degree of pseudogenization also in species that present a considerable number of intact V1R genes and a well-developed VNO. In total, of about 7000 V1R-like sequences that have been identified in 37 species, only 1809 represent functional genes (Young et al. 2010).
In general, V1R genes expanded in mammals that possess an highly organized VNO, such as platypus (283 genes), opossum (98), mouse (239), rat (109), rabbit (159), and mouse lemur (105), whereas they are absent or pseudogenized in Old World monkeys, apes, and humans that possess a vestigial and presumably non-functional VNO (Brykczynska et al. 2013; Grus & Zhang 2004; Grus et al. 2007; Hohenbrink et al. 2013; Rodriguez et al. 2002; Young et al. 2010) (Figure 10.2a). Surprisingly, the dog (Carnivora) genome displays a very small repertoire of intact V1R genes (9) in contrast to a much larger number of pseudogenes (38); a deterioration that does not seem to be correlated with the selective pressure that occurred during domestication, since the wolf genome presents homologous copies of the dog pseudogenes (Young et al. 2010). Interestingly, and in contrast to what was previously described, ruminant species such as cow, sheep, and goat evolved a significant number of V1R orthologs that are expressed in both the MOE and VNO. In cow, 70% of V1R genes has orthologs in the cross-species ruminant counterpart in contrast to the 9% of the mouse V1R orthologs identified in rat (mouse-rat are phylogenetically much closer species than cow-sheep) (Grus et al. 2005; Ohara et al. 2009) (Figure 10.2b). These unusual findings might suggest a possible involvement of both VNO and MOE in the detection of V1R ligands. Moreover, in these ruminant species (herbivores) V1Rs may also subserve to other functions rather than purely pheromonal. For instance, ruminant V1R orthologs could be involved in the detection of the same or closely related chemical cues as heterospecific odorants (allomones) (Ohara et al. 2009; Takigami et al. 2000; Wakabayashi et al. 2002).
In rodents, there are indications that V1Rs originated from an ancestor that probably possessed very few V1R genes. The majority of these genes duplicated independently generating a species-specific lineage. Lineage specificity appears so rigorous that a limited degree of orthology is only detectable in very closely related species such as Mus spretus and Mus musculus that diverged 1 million years ago (Grus & Zhang 2004; Park et al. 2011; Young et al. 2010).
The very large human V1R repertoire shows an almost complete pseudogenization (about 200 pseudogenes and only four intact genes) that occurred before the separation of hominoids (apes and humans) from Old World monkeys and was probably linked to the complete loss of the VNO (Zhang & Webb 2004).
Mouse V1Rs represent the first superfamily of pheromone receptors that have been discovered, and as with olfactory receptors, they show a monogenic expression that means that each VNO neuron expresses only one receptor type. In this murine species, V1Rs account for about 250 functionally receptors expressed in the apical VNO neurons. V1Rs are organized in 12 phylogenetically isolated subfamilies (a to k) with an identity ranging from 40% to 99% within subfamilies and from 15% to 40% between subfamilies (DelPunta et al. 2000; Rodriguez et al. 2002) (Figure 10.2b).
Positive selection (a ratio between nonsynonymous and synonymous amino acid substitution greater than 1) that is an index of a fast gene evolution has been reported acting on the V1R repertoire (Grus & Zhang 2004; Hohenbrink et al. 2012, 2013; Mundy & Cook 2003; Wyckoff et al. 2000). Thus, the rapid diversification of the mouse V1Rs developed in association with an increasingly high predominance of the pheromone-mediated communication. As a consequence, if a pheromone blend evolved rapidly and accumulated chemical changes, the V1Rs recognition system might have been subjected to a strong selective pressure (Shi et al. 2005).
In particular, the analysis of the sequences corresponding to the transmembrane domain 2 and the extracellular loops I and II of these receptors has shown the highest variability within species in combination with a strong positive selection, suggesting that these regions are implicated in pheromone binding (Rodriguez 2004).
Investigations on V1R expressing neurons (apical) led to the identification of four candidate V1R ligands, 2-hepatanone, 6-hydroxyl-6-methyl-3-heptanone, n-pentylacetate and isobutylamine (Table 10.1). These molecules are detectable in the mouse urine and have shown to activate, in a very specific fashion, distinct V1Rs (Boschat et al. 2002).
TABLE 10.1
V1R and V2R Ligands and Their Suggested Function in Pheromonal Communication
In contrast, other V1Rs appear to respond to multiple chemical cues, some of which are also emitted by heterospecific animals (Isogai et al. 2011) (Table 10.1).
From these studies, it is possible to envisage that distinct subpopulations of apical neurons, presumably expressing different V1R subtypes, are tuned to detect either individual or multiple pheromonal molecules (Boschat et al. 2002; Isogai et al. 2011).
A single behavioral study on deficient mice for V1R genes has provided clues on the function of some of these receptors. Mice bearing the complete deletion of the V1Ra and V1Rb gene clusters display a significant reduction of male-to-male and maternal aggressiveness compared to controls. In addition, these mutant mice do not show electrophysiological responses to 6-hydroxyl-6-methyl-3-heptanone, n-pentylacetate and isobutylamine (Del Punta et al. 2002).
In conclusion, a reliable correlation between the size of the V1R repertoire and the habits, the ecological system, and the sociosexual behavior of each species is difficult to establish. There appears to be a weak association between the size of intact V1R genes in terricolous mammals and two ecological factors: spatial (nest-living and open-living behavior) and rhythms (diurnal and nocturnal) activity (Wang et al. 2010). With few but significant exceptions (tenrec, sloth, squirrel, and pika), nest-living and nocturnal mammals (mouse, rat, kangaroo rat, rabbit, and lemur) seem to require more functional genes than open-living and diurnal mammals (among which horse, cow, and apes) perhaps in order to explore restricted and dark environments (Grus et al. 2005, 2007; Wang et al. 2010).
10.2.2. V2Rs
V2R genes have already appeared in fish (46 intact genes) and frog (about 300 intact genes and 450 pseudogenes) that possesses the largest repertoire among all vertebrates (Ji et al. 2009; Shi & Zhang 2007; Young & Trask 2007). A striking variation of V2R genes occurred in reptiles and mammals since (potentially) intact genes have only been reported in Squamata (lizard and snake), Didelphimorpha, (opossum), Rodentia, and Lagomorpha (rabbit). No functional V2Rs have been identified in Carnivora (dog), Artiodactyla (cow), and primates (macaque, chimpanzee, gorilla, and human) with the exception of prosimians (loris, lemur, and tarsier) (Dong et al. 2012; Hohenbrink et al. 2012; Ishii & Mombaerts 2011; Shi & Zhang 2007; Yang et al. 2005; Young & Trask 2007) (Figure 10.2a and d).
Mammalian V2Rs can be classified in five families A to E (Figure 10.2c). Families BCDE V2Rs are phylogenetically ancient and present orthologs in species of several orders. It is likely that all mammalian V2Rs originated from 4–5 ancestral genes (probably precursors of each family) that undertook a different degree of expansion according to each species.
Receptors of family A typically represent the majority of the V2Rs (95% in the mouse) in all species and show a strong lineage specificity so that orthologs can be exclusively found in related species, in which, however, they tend to form small but independent clades (Silvotti et al. 2007).
In murids, family A has further expanded originating two distinct groups including subfamilies A1–A6 (phylogenetically more recent and largely represented in mouse and rat) and subfamilies A8–A9 (with orthologs in Cricetidae and Caviidae) (Silvotti et al. 2011).
Already present in bony fish, family C is considered the most ancient among all other V2R families from which it considerably diverges (Silvotti et al. 2005). Family C-V2Rs are typically represented by a single gene in each species with the exception of Muroidea superfamily (Brykczynska et al. 2013; Rodriguez 2004; Shi & Zhang 2007). Intact genes are found in prosimians whereas Old World monkeys, apes, and humans possess only pseudogenes (Hohenbrink et al. 2013; Martini et al. 2001).
In mouse and rat, family C expanded by gene duplication and inversion originating seven and four homologue receptors, respectively; thus, this family is split into two subfamilies, C1 and C2. This expansion probably originated before the separation of the last common ancestor of Muridae and Caviidae (guinea pig) families and appears to parallel the establishment of subfamilies A1–A6 (Silvotti et al. 2011).
In the VNO, families-ABDE V2Rs are expressed monogenically; however, the basal neurons show a multigenic expression of V2Rs that seem to represent an exception of the one-neuron-one receptor rule (Belluscio et al. 1999; Bozza et al. 2002; Rodriguez et al. 1999; Serizawa et al. 2000). In fact, families-ABDE V2Rs are coexpressed with family-C V2Rs in a specific fashion (Martini et al. 2001; Silvotti et al. 2011). In the rat and mouse, the expansion of family C and family A defined two populations of basal neurons. One population expresses phylogenetically ancient V2Rs (subfamilies A8–A9, families BDE, and subfamily-C1 V2Rs), while the other population expresses multiple combinations of more recent V2R subfamilies (subfamilies A1–A6 and subfamily-C2 V2Rs) (Silvotti et al. 2011).
As for V1Rs, the multiple lineage-specific expansion of V2Rs with the concurrent sequence diversification is probably correlated with the detection of different ligands, resulting in a selective advantage. In particular, the sequence analysis of the extracellular N-terminal domain of V2Rs shows a positive selection that is absent in other region of the receptor. This observation strongly suggests that the extracellular region is involved in pheromone binding (Yang et al. 2005).
Evidence indicates that urinary proteins (MUPs), secretory peptides, and “self” molecules (MHC) are some of the V2R ligands (Ishii & Mombaerts 2008; Kimoto et al. 2005; Leinders-Zufall et al. 2009; Loconto et al. 2003; Papes et al. 2010; Sturm et al. 2013). Recently, the lacrimal peptide (ESP1) has been reported to bind and activate V2Rp5, a member of subfamily A3. ESP1 is secreted by the extraorbital gland of the male mouse and induces a specific sexual behavior (lordosis) in females. A family-B V2R member, V2r1b, is probably responsible for the detection of MHC peptides, some of which are quantifiable in urine and are believed to be involved in the genotype discrimination (Haga et al. 2010; Leinders-Zufall et al. 2004, 2009; Sturm et al. 2013). In addition, MUPs have been demonstrated to activate neurons expressing not yet characterized V2Rs. Since MUPs are involved in male-to-male aggressiveness it is likely that V2Rs are also involved in individual and sexual recognition (Chamero et al. 2007; Ishii & Mombaerts 2008; Leinders-Zufall et al. 2009) (Table 10.1). In light of these findings, V2Rs seem to respond to proteinaceous molecules mainly deriving from bodily secretions. In contrast to V1Rs, each V2R appears to be tuned to recognized one or very few pheromonal molecules (Isogai et al. 2011).
In summary, V1Rs and V2Rs are extremely varied and expanded in different lineages of terrestrial vertebrates, and, in particular, they show a rapid turnover in rodent species (Grus et al. 2005; Young et al. 2010). Interestingly, the appearance of the VNO does not seem to correlate with the appearance of V1R and V2R genes (Yang et al. 2005) (Figure 10.2a,d). Thus, it is possible that V1Rs and V2Rs expression was initially established in other organs like, for example, the main olfactory system where these receptors are indeed reportedly expressed (Pfister et al. 2007; Rodriguez & Mombaerts 2002; Syed et al. 2013).
The ancient evolutionary origin of V1Rs and V2Rs is supported by the recent identification of genes encoding these receptors in zebra fish (Hashiguchi et al. 2008; Grus & Zhang 2009; Saraiva & Korsching 2007). The tetrapod ancestor probably possessed a very small repertoire of V1Rs and V2Rs (probably 3–4 genes). V2Rs underwent a large expansion in amphibians reptiles (snakes and lizards) and in some mammalian orders such as Didelphimorpha (opossum), and Rodentia (Brykczynska et al. 2013; Dong et al. 2012; Ishii & Mombaerts 2011; Ji et al. 2009; Nei et al. 2008; Shi & Zhang 2007; Young & Trask 2007). In contrast, V1Rs scarcely duplicated in amphibians (21 genes) and reptiles (four genes). Interestingly, both V1Rs and V2Rs significantly expanded in family Muridae and reduced (V1Rs) or disappeared (no intact genes) (V2Rs) in Laurasiatheria. Thus, the rapid evolution of both V1R and V2R superfamilies in some genome lineages is interchanged with the loss of these receptors in some other species, an observation confirmed by the high number of pseudogenes (Figure 10.2a, b, and d).
The ratio between the number of V1Rs and V2Rs in vertebrates may also address some questions about the functions of these receptors. In fact, the evolutionary transition of early tetrapods from water to land corresponds to the positive inversion of the ratio between V1R and V2R genes.
This would seem to suggest the prevalence of a chemical communication mediated by airborne pheromones in mammals in terricolous species (Shi & Zhang 2007).
10.2.3. FPRs
FPRs belong to the seven-transmembrane domain G protein coupled receptor superfamily and are present in tissues and cell lines involved in the immune response as lymphocytes, monocytes, macrophages, and microglia of most mammalian genera (Le et al. 2002; Migeotte et al. 2006); however, recent findings indicate that a murine subclass of FPRs are specifically expressed in the VNO (Liberles et al. 2009; Riviere et al. 2009) (Table 10.2).
TABLE 10.2
Expression of FPRs in Humans and Mice
The phylogenetic tree of FPRs suggests a very complex distribution of these receptors. FPRs represent a monophyletic group divided into three clades, FPR1, FPRL1, and FPRL2 (Yang & Shi 2010).
FPR1s are phylogenetically the most ancient as a related gene is present in the lamprey (Petromyzontiformes) genome (Figure 10.2e). In placental mammals FPR1 orthologs are present in many species irrespective of the development of a functional VNO. Interestingly, Laurasiatheria, which include Carnivora (cat and dog), Perissodactyla (horse), Artiodactyla (cow, pig, and sheep), and Chiroptera (bat), have probably lost the fpr1 genes (and pseudogenes) (Figure 10.2e). Didelphimorpha (opossum) possess fpr-like genes with 40% identity with respect to placental mammals.
The second FPR clade, FPRL1, which is phylogenetically more recent, includes receptors already present in Laurasiatheria that forms a lineage-specific gene cluster that was erroneously regarded as VNO specific, since family Muridae predominantly express these receptors in the VNO.
The third FPR clade, FPRL2, which presumably arose from the duplication of the FPR1 gene, appears to be expanded in primates, forming a lineage-specific clade with a homology of 85%–99% between species (Figure 10.2e).
In mice, FPRL1s represent a rodent specific branch that is characterized by seven protein coding genes located on chromosome 17: Fpr-rs1, Fpr-rs2, Fpr-rs3, Fpr-rs4, Fpr-rs6, and Fpr-rs7 (Fpr-rs5 is a pseudogene). These genes show an identity that ranges from 67% to 96%, and with the exclusion of Fpr-rs2, they appear to be exclusively expressed in the VNO. In rat, a similar expansion occurred with the presence of seven functional genes that show a one-to-one ortology with the mouse genes. Moreover, VNO-FPRs appear to be already established in other rodent species of different genera as rat and gerbil (Liberles et al. 2009; Riviere et al. 2009). Thus, it is likely that VNO-FPRs were already present in a Muridae ancestor (Figure 10.2a,e).
FPR-rs3, FPR-rs4, FPR-rs6, and FPR-rs7 are exclusively or predominantly expressed in the apical neurons and coexpressed with Gαi2. In contrast, FPR-rs1 is located more basally and is coexpressed with Gαo (Liberles et al. 2009). The FPR expression pattern appears punctuate and therefore similar to that observed for V1Rs and for most members of V2Rs, thus suggesting a monogenic transcription for these genes (Dulac & Axel 1995; Herrada & Dulac 1997; Ryba & Tirindelli 1997). Since there is no evidence of a coexpression of FRPs with V1Rs or V2Rs, it is likely that specific subsets of VNO neurons exclusively express FPRs (Liberles et al. 2009; Riviere et al. 2009; Yang & Shi 2010). The functional role of both non-VNO and VNO-FPRs is still unclear although a conspicuous number of FPRs agonists and antagonists has been identified (Seifert & Wenzel-Seifert 2003; Thoren et al. 2010).
Human and mouse FPRs are activated by viral and bacterial products (Bae et al. 2003a,b). The most effective ligands of almost all FPRs is the bacterial N-formyl-methionyl-leucyl-phenylalanine (fMLF) that triggers several biological immunological functions in neutrophils, monocytes, and macrophages (Boulay et al. 1990; Cevik-Aras et al. 2012; Le et al. 2002). In particular, the lack of FPR1 gene causes an increase of Listeria monocytogenes sensitivity in mouse (Gao et al. 1999).
As for VNO-FPRs, FPR-rs1 was reportedly identified to be specific to lipoxin A4 (it was in fact named as LXA4R9), a lipid mediator generated at the site of inflammation that both regulates the transport of intermediates between platelets, neutrophils, and natural killer cells and lymphocytes activation in the chronic inflammations (Migeotte et al. 2006; Takano et al. 1997). In contrast, cloned FPR-rs1 and 3 predominantly respond to the cathelin-related antimicrobial peptide (CRAMP) that acts as chemotactic factor in vivo. FPR-rs6 senses fMLF whereas FPR-rs4 and 7 detect both ligands. Moreover, FPR-rs4 also responds to lipoxin A4 (Riviere et al. 2009) (Table 10.1).
Similarly to all other FPRs, each VNO-FPR probably responds to a large array of ligands, most of which have not yet been characterized. Moreover, VNO-FPRs responding to ligands do not show particular selectivity for these receptors, being effective at a similar concentration on several FPR subtypes of different species. This observation appears to contrast with the most recent phylogenetic studies on FPR genes that aim at concluding that the selective pressure exclusively acting on VNO-FPRs rather than all other FPRs is possibly linked to the functional transition from host defense (FPRL1) to vomeronasal chemosensation (VNO-FPRs) (Liberles et al. 2009).
In light of these findings it appears very difficult to define a pheromonal role for these receptors. One possibility is that VNO-FPRs may exploit the specific function of detecting compounds specifically synthesized by the bacterial flora inhabiting the secretions of conspecific or heterospecific animals, thus allowing individual identification (Riviere et al. 2009).
As a summary of vomeronasal receptors, it is possible to envisage that V1Rs recognize volatile molecules also produced by sympatric species whereas V2Rs respond to excretory proteins and peptides exclusively expressed by individuals of the same species. In contrast, FPRs sense microbial peptides that, as suggested above, may also be indirectly involved in both species or individual recognition (Del Punta et al. 2002; Chamero et al. 2007; Isogai et al. 2011; Kimoto et al. 2005; Leinders-Zufall et al. 2000, 2004; Liberles et al. 2009; Riviere et al. 2009).
10.3. SIGNAL TRANSDUCTION MECHANISMS
Binding of pheromones to vomeronasal receptors initiates a transduction cascade that produces an excitatory response. Pheromonal stimuli cause membrane depolarization and increase the action potential firing rate and the intracellular calcium concentration in VSNs (Celsi et al. 2012; Holy et al. 2000; Inamura & Kashiwayanagi 2000; Leinders-Zufall et al. 2000; Nodari et al. 2008; Shimazaki et al. 2006; Spehr et al. 2002). Despite several similarities in response to stimuli are shared between vomeronasal and olfactory sensory neurons, the transduction mechanisms used by these chemosensory cells are distinct (Berghard & Buck 1996; Wu et al. 1996).
10.3.1. G Proteins
As previously described, the two subsets of apical and basal VSNs expressing V1Rs or V2Rs and FPRs also express different G proteins (Figure 10.3). Berghard and Buck identified two types of G protein α subunits in basal, Gαo, and apical, Gαi2, mouse VSNs (Berghard & Buck 1996). Ultrastructural studies showed that both Gαo and Gαi2 are located in the microvilli where the vomeronasal transduction occurs (Menco et al. 2001). The activation of GPRCs causes the release of the βγ complex of the heterotrimeric G protein Go and Gi2 responsible for phospholipase C (PLC) activation, indicating that β and γ subunits play a pivotal role in vomeronasal sensory transduction (Clapham & Neer 1997). Runnenburger and colleagues reported that one type of β subunit, β2, is expressed in the vomeronasal sensory epithelium and that an antibody against β2 caused a reduction of IP3 production in microvilli extracts stimulated with urine (Runnenburger et al. 2002). Moreover, two γ subunits, γ2 and γ8, are expressed in VSNs (Runnenburger et al. 2002; Ryba & Tirindelli 1995; Tirindelli & Ryba 1996). Interestingly, the expression of the γ2 subunit is confined to apical neurons, whereas the γ8 subunit is preferentially expressed in the basal neuronal population. Treatment of microvilli extract with specific antibodies against γ2 and γ8 reduced the production of IP3 upon urine stimulation, suggesting that both subunits play a role in sensory transduction (Runnenburger et al. 2002).
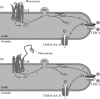
FIGURE 10.3
Signaling molecules involved in the transduction of pheromonal stimuli. (a) The binding of pheromones to V1R receptors in the microvilli activates PLC through the G protein Goβ2γ8. PLC produces the second messengers DAG and IP3. The gating (more...)
The functional role of G proteins expressed in the VNO has been investigated in knockout mice. Gαi2-deficient animals produced a severe postnatal neurodegeneration of apical VSNs, causing the death of about 50% of VSNs and a consequent reduction of about 50% of the glomerular layer of the anterior AOB (Norlin et al. 2003). Using c-Fos as a marker for neuronal activity, it has been reported that the lack of Gαi2 caused a fivefold reduction of c-Fos expression in the anterior AOB of male mice exposed to bedding soiled by female mice. Behavioral studies have revealed that male mice display normal sexual behaviors whereas females present a strong reduction of maternal aggression, indicating that Gαi2-expressing VSNs mediated these processes (Norlin et al. 2003). Direct measurements of responses to stimuli of VSNs lacking Gαi2 would help clarify its role in sensory transduction, but at present they are not available.
The role of G proteins in survival of VSNs was confirmed also in conditional and general knockout mice for Gαo: a severe postnatal neurodegeneration of basal VSNs caused the death of about 50% VSNs (Chamero et al. 2011; Tanaka et al. 1999). Chamero and colleagues (2011) measured the responses of VSNs with electrovomeronasogram (EVG) recordings and Ca2+ imaging assay. These authors reported that VSNs from Gαo knockout mice did not respond to stimulation with known V2R ligands, such as MHC class I peptides, ESPs, and MUPs, and with FPR-rs1 agonists. In contrast, Gαo-deficient VSNs present normal responses to V1R ligands such as 2-heptanone and isobutylamine and to FPR-rs2-5 (Chamero et al. 2011). Furthermore, behavioral studies showed a decreased male-to-male aggressiveness mediated by MHC class I peptides and MUPs, and surprisingly, a reduction of maternal aggression, a behavior that is also mediated by V2R-Gαi2-expressing neurons (Chamero et al. 2011).
10.3.2. Phospholipase C
While the molecular identity of the phospholipase C (PLC) subtypes expressed in the VNO is not identified, functional studies have indicated that some responses of VSNs to stimuli are mediated by PLC activation. Holy and colleagues (2000) measured the electrical activity of mouse VSNs in response to urine using multielectrode array recordings and showed that the PLC inhibitor U73122 blocked spiking responses to urine, whereas the inactive structural analogous U73343 did not produce a measurable effect. Similar results were obtained by Spehr and colleagues (2002) monitoring the activity of rat VSNs upon urine stimulation using Ca2+-imaging. PLC hydrolizes PIP2 into IP3 and diacylglycerol (DAG) (Figure 10.3). Additional evidence of the involvement of PLC in the transduction cascade comes from biochemical studies showing that stimulation of VSNs with urine induced the production of IP3 in several species (Krieger et al. 1999; Kroner et al. 1996; Sasaki et al. 1999; Thompson et al. 2004). However, the role of IP3 in signal transduction is not clear. Indeed, urine-stimulated Ca2+ increase in rat VSNs was not affected by the block of IP3 receptors (Spehr et al. 2002), although the presence of IP3 in the patch pipette induced a nonselective cation current in about 50% of the recorded rat VSNs (Inamura et al. 1997).
10.3.3. Ion Channels
10.3.3.1. TRPC2
Liman and colleagues (1999) first showed that the transient receptor potential canonical 2 (TRPC2) is expressed in VSNs. TRPC2 localizes in the microvillar region of the VNO (Dibattista et al. 2012; Liman et al. 1999; Menco et al. 2001; Stowers et al. 2002). This molecule belongs to the TRPC subfamily, a class of cation channels that are characterized by PLC-dependent Ca2+ permeability. Drosophila homologues of mammalian TRPC, TRP and TRPL, are activated during the PLC-mediated phototransduction cascade (Yau & Hardie 2009). In Old World monkeys and in humans the TRPC2 gene has pseudogenized along with the loss of a functional VNO (Liman & Innan 2003; Zhang & Webb 2003), suggesting that the evolution of the trichromatic vision releases the selective pressure of signaling mediated by pheromones (Liman & Innan 2003; Zhang & Webb 2003).
The involvement of TRPC2 in vomeronasal sensory transduction has been clearly demonstrated using TRPC2 knockout mice. The lack of TRPC2 caused a strong postnatal degeneration of VSNs, with the loss of about 75% V2R-expressing neurons and 50% V1R-expressing neurons at 2 months of age (Stowers et al. 2002). This phenotype mirrors the effects of the deletion of the G protein α subunits (Chamero et al. 2011; Norlin et al. 2003; Tanaka et al. 1999), confirming the importance of a functional transduction machinery for survival of VSNs.
Functional properties of VSNs in TRPC2 knockout mice showed discrepancies among different studies (Kelliher et al. 2006; Kim et al. 2011; Leypold et al. 2002; Stowers et al. 2002). Some authors reported a strong reduction of the response to urine stimulation measured with EVG recordings (Leypold et al. 2002), whereas others showed the complete loss of neuronal activation by urine using multielectrode array (Stowers et al. 2002). Moreover, Kelliher and colleagues (2006) found that the EVG response to MHC class I peptides as well as the Bruce effect (pregnancy failure induced by exposure to an unrelated male (Bruce 1959)) that is mediated by this class of molecules was unaffected in TRPC2-deficient mice, indicating that TRPC2 is not involved in the mediation of some V2R-dependent processes (see Section 10.2.2). TRPC2-deficient males also show a strong decrease in the male-to-male and maternal aggressive (towards males) behavior, which can be explained as the inability of these mice to discriminate the gender (Leypold et al. 2002; Stowers et al. 2002).
Some studies indicate that TRPC2 activation involved the PLC-mediated DAG production. Indeed, experiments using inside-out excised patches from dendritic knob of mouse VSNs showed the presence of a nonselective cation current activated by DAG analogues and absent in TRPC2 deficient VSNs (Lucas et al. 2003). Moreover, whole-cell patch-clamp recordings have revealed that urine was able to induce a similar nonselective cation current blocked by the TRP channel blocker, A2B (Lucas et al. 2003). Finally, the pharmacological block of DAG degradation through DAG kinase induced the activation of TRPC2 current by PLC basal activity (Lucas et al. 2003). At present, it is unclear whether DAG directly or indirectly activates TRPC2. It is noteworthy that in Drosophila, TRP/TRPL channels are gated by protons produced by the hydrolysis of phosphoinositides to DAG (Huang et al. 2010).
10.3.3.2. Ca2+-Activated Cl− Channels
Recent studies indicate that Ca2+-activated Cl− channels are expressed in VSNs (Dibattista et al. 2012; Kim et al. 2011; Pifferi et al. 2012; Yang & Delay 2010). Yang and Delay (2010) reported that 80% of the urine-activated current, measured with perforated patch recording on dissociated mouse VSNs, is carried by Ca2+-activated Cl− channels and that a Ca2+ influx is necessary to activate the Cl− channels.
Kim and colleagues (2011) confirmed that a Ca2+ -activated Cl− current contributes to responses to urine, but they also suggested that this current can be activated both by Ca2+ influx through the TRPC2 channel and by Ca2+ release from internal stores mediated by IP3 in a TRPC2-independent manner. Interestingly, Kim and colleagues (2011) obtained extracellular recordings in VNO slices and showed that both spontaneous and urine-evoked firing activities were abolished by NFA and SITS, two commonly used blockers of Ca2+-activated Cl− channels, indicating that the activation of these channels is required for neuronal activity. The presence of Ca2+-activated Cl− channels in the apical portion of VSNs was confirmed by local photo-release of Ca2+ from caged Ca2+ (Dibattista et al. 2012).
RT-PCR experiments showed that some members of the TMEM16/anoctamin family are expressed in the VNO (Billig et al. 2011; Dibattista et al. 2012; Yang & Delay 2010). TMEM16A/anoctamin1 and TMEM16B/anoctamin2 have been shown to be Ca2+-activated Cl− channels (Caputo et al. 2008; Pifferi et al. 2009; Schroeder et al. 2008; Scudieri et al. 2012; Stephan et al. 2009; Stöhr et al. 2009; Yang et al. 2008). Immunohistological data has established that both TMEM16A and TMEM16B are expressed in the apical region of the VNO (Billig et al. 2011; Dauner et al. 2012; Dibattista et al. 2012; Rasche et al. 2010). Dibattista et al. (2012) demonstrated that TMEM16A and TMEM16B largely colocalize with TRPC2 at the apical surface of the vomeronasal epithelium, and that TMEM16A and TMEM16B are coexpressed in microvilli of both apical and basal isolated vomeronasal sensory neurons, therefore suggesting that these two anion channels are likely to be involved in vomeronasal transduction (Figure 10.3). However, as an estimate of the Cl− concentration in fluid filling the VNO lumen and inside VSNs is not currently available, the direction of the flux of Cl− ions cannot be determined. Thus, depending on the Cl− equilibrium potential, Ca2+-activated Cl− channels may contribute to VSNs depolarization or hyperpolarization.
10.3.3.3. Other Ion Channels
Several other ion channels are expressed in VSNs and have been proposed to be involved in signal transduction.
Kim and colleagues (2012) reported that calcium-activated and G-protein-activated inwardly rectifying potassium channels SK3 and GIRK1 contribute to the urine-mediated response in mouse VSNs. Interestingly, the depolarizing effect of these channels in vivo was due to an unusual high K+ concentration (about 66 mM) in the fluid filling the lumen of VNO, resembling the well-known high K+ concentration in the cochlear endolymph. Moreover, a decreased aggressiveness and an impairment of sociosexual behavior is associated with the deletion of SK3 and GIRK1genes (Kim et al. 2012).
Zhang and colleagues (2008) reported that the blockage of a large-conductance calcium-activated potassium channel (BK) by iberiotoxin (Ibx) induced an increase of the transduction current amplitude and of the firing rates in mouse VSNs stimulated with urine, suggesting that BK channels are also involved in vomeronasal signal transduction (Zhang et al. 2008). This data could be interpreted in light of the recent finding about the high extracellular K+ concentration in the VNO lumen, suggesting an inhibitory role of BK channels.
An interesting open question is the possible role of arachidonic acid (AA) in sensory transduction. Spehr and colleagues (2002) first showed that AA was able to produce an increase in intracellular Ca2+ concentration in rat VSNs. Zhang and colleagues (2010) reported that AA was able to activate a Ca2+-dependent Ca2+ permeable channel in inside-out patches excised from dendritic knob of mouse VSNs that is independent on TRPC2. Moreover, pharmacological blockage of DAG lipase, an enzyme necessary to the production of AA upon PLC activation, induced a reduction of urine-induced current (Zhang et al. 2010), although another report failed to detect any effect of DAG lipase inhibition (Lucas et al. 2003).
Another Ca2+-dependent conductance was studied by Liman (2003) in the knob/microvilli of hamster VNSs. This author reported the presence of a Ca2+-activated nonselective cation channel with a single-channel conductance of about 25 pS and with an EC50 for Ca2+ of 0.51 mM (at −80 mV). Moreover, the activity of this channel was inhibited by cAMP with an IC50 of 43 µM (at −80 mV). It has been suggested that TRPM4 is responsible for this conductance but a definitive proof is still missing (Liman 2003). Interestingly, Spehr and colleagues (2009) reported that a Ca2+-activated nonselective cation channel was also present in the knob/microvilli of mouse VSNs with sensitivity to Ca2+ in micromolar range. In contrast to these results, the local photo-release of intracellular Ca2+ from caged compounds did not elicit any cation current in mouse VSNs (Dibattista et al. 2012). It is possible that these discrepancies could be partially due to species-specificity or to the presence of different populations of VSNs using different transduction mechanisms.
The involvement of cAMP or cGMP in the mediation of pheromonal sensation is still under debate. It has been reported that some pheromones induce a reduction of cAMP concentration (Rossler et al. 2000; Zhou & Moss 1997). However, the decrease in cAMP has a slow kinetics compared with sensory stimulation, suggesting that cAMP could have a long-lasting effect in modulation of VSNs responses. Some components of the cAMP signaling cascade have been identified in VSNs as adenylyl cyclase types 2, 4, 5, and 6 (Berghard & Buck 1996; Lee et al. 2008; Rossler et al. 2000), the subunit CNGA4 of the olfactory cyclic nucleotide-gated channel (Berghard & Buck 1996; Lee et al. 2008), and phosphodiesterase types 1 and 4 (Cherry & Pho 2002; Lau & Cherry 2000). Possible targets for modulatory actions by cAMP include the Ca2+-activated cation channel (Liman & Innan 2003) and the hyperpolarization-activated cyclic nucleotide-gated (HCN) cation channels that contribute to setting the resting membrane potential and to increasing excitability at stimulus threshold (Dibattista et al. 2012).
REFERENCES
- Bae Y.S, Song J.Y, Kim Y, He R, Ye R.D, Kwak J.Y, Suh P.G, Ryu S.H. Differential activation of formyl peptide receptor signaling by peptide ligands. Mol Pharmacol. 2003a;64:841–847. [PubMed: 14500740]
- Bae Y.S, Yi H.J, Lee H.Y, Jo E.J, Kim J.I, Lee T.G, Ye R.D, Kwak J.Y, Ryu S.H. Differential activation of formyl peptide receptor-like 1 by peptide ligands. J Immunol. 2003b;171:6807–6813. [PubMed: 14662886]
- Baum M.J, Kelliher K.R. Complementary roles of the main and accessory olfactory systems in mammalian mate recognition. Annu Rev Physiol. 2009;71:141–160. [PubMed: 18817511]
- Belluscio L, Koentges G, Axel R, Dulac C. A map of pheromone receptor activation in the mammalian brain. Cell. 1999;97:209–220. [PubMed: 10219242]
- Berghard A, Buck L.B. Sensory transduction in vomeronasal neurons: Evidence for G alpha o, G alpha i2, and adenylyl cyclase II as major components of a pheromone signaling cascade. J Neurosci. 1996;16:909–918. [PMC free article: PMC6578816] [PubMed: 8558259]
- Billig G.M, Pal B, Fidzinski P, Jentsch T.J. Ca2+-activated C1− currents are dispensable for olfaction. Nat Neurosci. 2011;14:763–769. [PubMed: 21516098]
- Boschat C, Pelofi C, Randin O, Roppolo D, Luscher C, Broillet M.C, Rodriguez I. Pheromone detection mediated by a V1r vomeronasal receptor. Nat Neurosci. 2002;5:1261–1262. [PubMed: 12436115]
- Boulay F, Tardif M, Brouchon L, Vignais P. Synthesis and use of a novel N-formyl peptide derivative to isolate a human N-formyl peptide receptor cDNA. Biochem Biophys Res Commun. 1990;168:1103–1109. [PubMed: 2161213]
- Bozza T, Feinstein P, Zheng C, Mombaerts P. Odorant receptor expression defines functional units in the mouse olfactory system. J Neurosci. 2002;22:3033–3043. [PMC free article: PMC6757547] [PubMed: 11943806]
- Bruce H.M. An exteroceptive block to pregnancy in the mouse. Nature. 1959;184:105. [PubMed: 13805128]
- Brykczynska U, Tzika A.C, Rodriguez I, Milinkovitch M.C. Contrasted evolution of the vomeronasal receptor repertoires in mammals and squamate reptiles. Genome Biol Evol. 2013;5:389–401. [PMC free article: PMC3590772] [PubMed: 23348039]
- Caputo A, Caci E, Ferrera L, Pedemonte N, Barsanti C, Sondo E, Pfeffer U, Ravazzolo R, Zegarra-Moran O, Galietta L.J.V. TMEM16A, a membrane protein associated with calcium-dependent chloride channel activity. Science. 2008;322:590–594. [PubMed: 18772398]
- Celsi F, D’Errico A, Menini A. Responses to sulfated steroids of female mouse vomeronasal sensory neurons. Chem Senses. 2012;37:849–858. [PubMed: 22923146]
- Cevik-Aras H, Kalderen C, Jenmalm Jensen A, Oprea T, Dahlgren C, Forsman H. A non-peptide receptor inhibitor with selectivity for one of the neutrophil formyl peptide receptors, FPR 1. Biochem Pharmacol. 2012;83:1655–1662. [PubMed: 22410002]
- Chamero P, Katsoulidou V, Hendrix P, Bufe B, Roberts R, Matsunami H, Abramowitz J, Birnbaumer L, Zufall F, Leinders-Zufall T. G protein G(alpha)o is essential for vomeronasal function and aggressive behavior in mice. Proceedings of the National Academy of Sciences of the United States of America. 2011;108:12898–12903. [PMC free article: PMC3150917] [PubMed: 21768373]
- Chamero P, Marton T.F, Logan D.W, Flanagan K, Cruz J.R, Saghatelian A, Cravatt B.F, Stowers L. Identification of protein pheromones that promote aggressive behaviour. Nature. 2007;450:899–902. [PubMed: 18064011]
- Cherry J.A, Pho V. Characterization of cAMP degradation by phosphodiesterases in the accessory olfactory system. Chem Senses. 2002;27:643–652. [PubMed: 12200345]
- Churakov G, Sadasivuni M.K, Rosenbloom K.R, Huchon D, Brosius J, Schmitz J. Rodent evolution: Back to the root. Mol Biol Evol. 2010;27:1315–1326. [PubMed: 20100942]
- Clapham D.E, Neer E.J. G protein beta gamma subunits. Annu Rev Pharmacol Toxicol. 1997;37:167–203. [PubMed: 9131251]
- Date-Ito A, Ohara H, Ichikawa M, Mori Y, Hagino-Yamagishi K. Xenopus V1R vomeronasal receptor family is expressed in the main olfactory system. Chem Senses. 2008;33:339–346. [PubMed: 18238827]
- Dauner K, Lissmann J, Jeridi S, Frings S, Mohrlen F. Expression patterns of anoctamin 1 and anoctamin 2 chloride channels in the mammalian nose. Cell Tissue Res. 2012;347:327–341. [PubMed: 22314846]
- Del Punta K, Leinders-Zufall T, Rodriguez I, Jukam D, Wysocki C.J, Ogawa S, Zufall F, Mombaerts P. Deficient pheromone responses in mice lacking a cluster of vomeronasal receptor genes. Nature. 2002;419:70–74. [PubMed: 12214233]
- Del Punta K, Rothman A, Rodriguez I, Mombaerts P. Sequence diversity and genomic organization of vomeronasal receptor genes in the mouse. Genome Res. 2000;10:1958–1967. [PMC free article: PMC313053] [PubMed: 11116090]
- Dennis J.C, Allgier J.G, Desouza L.S, Eward W.C, Morrison E.E. Immunohistochemistry of the canine vomeronasal organ. J Anat. 2003;202:515–524. [PMC free article: PMC1571110] [PubMed: 12846473]
- Dennis J.C, Smith T.D, Bhatnagar K.P, Bonar C.J, Burrows A.M, Morrison E.E. Expression of neuron-specific markers by the vomeronasal neuroepithelium in six species of primates. Anat Rec A Discov Mol Cell Evol Biol. 2004;281:1190–1200. [PubMed: 15470676]
- Dibattista M, Amjad A, Maurya D.K, Sagheddu C, Montani G, Tirindelli R, Menini A. Calcium-activated chloride channels in the apical region of mouse vomeronasal sensory neurons. J Gen Physiol. 2012;140:3–15. [PMC free article: PMC3382724] [PubMed: 22732308]
- Dong D, Jin K, Wu X, Zhong Y. CRDB: Database of chemosensory receptor gene families in vertebrate. PLoS One. 2012;7:e31540. [PMC free article: PMC3290609] [PubMed: 22393364]
- Dulac C, Axel R. A novel family of genes encoding putative pheromone receptors in mammals. Cell. 1995;83:195–206. [PubMed: 7585937]
- Dulac C, Torello A.T. Molecular detection of pheromone signals in mammals: From genes to behaviour. Nat Rev Neurosci. 2003;4:551–562. [PubMed: 12838330]
- Emes R.D, Beatson S.A, Ponting C.P, Goodstadt L. Evolution and comparative genomics of odorant- and pheromone-associated genes in rodents. Genome Res. 2004;14:591–602. [PMC free article: PMC383303] [PubMed: 15060000]
- Gao J.L, Lee E.J, Murphy P.M. Impaired antibacterial host defense in mice lacking the N-formylpeptide receptor. J Exp Med. 1999;189:657–662. [PMC free article: PMC2192926] [PubMed: 9989980]
- Grus W.E, Zhang J. Rapid turnover and species-specificity of vomeronasal pheromone receptor genes in mice and rats. Gene. 2004;340:303–312. [PubMed: 15475172]
- Grus W.E, Zhang J. Origin of the genetic components of the vomeronasal system in the common ancestor of all extant vertebrates. Mol Biol Evol. 2009;26:407–419. [PMC free article: PMC2727394] [PubMed: 19008528]
- Grus W.E, Shi P, Zhang J. Largest vertebrate vomeronasal type 1 receptor gene repertoire in the semiaquatic platypus. Mol Biol Evol. 2007;24:2153–2157. [PubMed: 17666439]
- Grus W.E, Shi P, Zhang Y.P, Zhang J. Dramatic variation of the vomeronasal pheromone receptor gene repertoire among five orders of placental and marsupial mammals. Proc Natl Acad Sci U S A. 2005;102:5767–5772. [PMC free article: PMC556306] [PubMed: 15790682]
- Haga S, Hattori T, Sato T, Sato K, Matsuda S, Kobaya-kawa R, Sakano H, Yoshihara Y, Kikusui T, Touha-ra K. The male mouse pheromone ESP1 enhances female sexual receptive behaviour through a specific vomeronasal receptor. Nature. 2010;466:118–122. [PubMed: 20596023]
- Hashiguchi Y, Furuta Y, Nishida M. Evolutionary patterns and selective pressures of odorant/pheromone receptor gene families in teleost fishes. PloS One. 2008;3:e4083. [PMC free article: PMC2605262] [PubMed: 19116654]
- Herrada G, Dulac C. A novel family of putative pheromone receptors in mammals with a topographically organized and sexually dimorphic distribution. Cell. 1997;90:763–773. [PubMed: 9288755]
- Hohenbrink P, Mundy N.I, Zimmermann E, Radespiel U. First evidence for functional vomeronasal 2 receptor genes in primates. Biol Lett. 2013;9:20121006. [PMC free article: PMC3565523] [PubMed: 23269843]
- Hohenbrink P, Radespiel U, Mundy N.I. Pervasive and ongoing positive selection in the vomeronasal-1 receptor (V1R) repertoire of mouse lemurs. Mol Biol Evol. 2012;29:3807–3816. [PubMed: 22821010]
- Holy T.E, Dulac C, Meister M. Responses of vomeronasal neurons to natural stimuli. Science. 2000;289:1569–1572. [PubMed: 10968796]
- Huang J, Liu C.-H, Hughes S.A, Postma M, Schwiening C.J, Hardie R.C. Activation of TRP channels by protons and phosphoinositide depletion in Drosophila photoreceptors. Curr Biol. 2010;20:189–197. [PubMed: 20116246]
- Huchon D, Chevret P, Jordan U, Kilpatrick C.W, Ranwez V, Jenkins P.D, Brosius J, Schmitz J. Multiple molecular evidences for a living mammalian fossil. Proc Natl Acad Sci U S A. 2007;104:7495–7499. [PMC free article: PMC1863447] [PubMed: 17452635]
- Inamura K, Kashiwayanagi M. Inward current res-ponses to urinary substances in rat vomeronasal sensory neurons. Eur J Neurosci. 2000;12:3529–3536. [PubMed: 11029622]
- Inamura K, Kashiwayanagi M, Kurihara K. Inositol-1,4,5-trisphosphate induces responses in receptor neurons in rat vomeronasal sensory slices. Chem Senses. 1997;22:93–103. [PubMed: 9056089]
- Ishii T, Mombaerts P. Expression of nonclassical class I major histocompatibility genes defines a tripartite organization of the mouse vomeronasal system. J Neurosci. 2008;28:2332–2341. [PMC free article: PMC6671199] [PubMed: 18322080]
- Ishii T, Mombaerts P. Coordinated coexpression of two vomeronasal receptor V2R genes per neuron in the mouse. Mol Cell Neurosci. 2011;46:397–408. [PubMed: 21112400]
- Isogai Y, Si S, Pont-Lezica L, Tan T, Kapoor V, Murthy V.N, Dulac C. Molecular organization of vomeronasal chemoreception. Nature. 2011;478:241–245. [PMC free article: PMC3192931] [PubMed: 21937988]
- Jemiolo B, Andreolini F, Xie T.M, Wiesler D, Novotny M. Puberty-affecting synthetic analogs of urinary chemosignals in the house mouse, Mus domesticus. Physiol Behav. 1989;46:293–298. [PubMed: 2602471]
- Ji Y, Zhang Z, Hu Y. The repertoire of G-protein-coupled receptors in Xenopus tropicalis . BMC Genomics. 2009;10:263. [PMC free article: PMC2709155] [PubMed: 19508718]
- Kelliher K.R, Spehr M, Li X.H, Zufall F, Leinders-Zufall T. Pheromonal recognition memory induced by TRPC2-independent vomeronasal sensing. Eur J Neurosci. 2006;23:3385–3390. [PubMed: 16820028]
- Kim S, Ma L, Jensen K.L, Kim M.M, Bond C.T, Adelman J.P, Yu C.R. Paradoxical contribution of SK3 and GIRK channels to the activation of mouse vomeronasal organ. Nat Neurosci. 2012;15:1236–1244. [PMC free article: PMC3431453] [PubMed: 22842147]
- Kim S, Ma L, Yu C.R. Requirement of calcium-activated chloride channels in the activation of mouse vomeronasal neurons. Nat Commun. 2011;2:365. [PMC free article: PMC3156823] [PubMed: 21694713]
- Kimoto H, Haga S, Sato K, Touhara K. Sex-specific peptides from exocrine glands stimulate mouse vomeronasal sensory neurons. Nature. 2005;437:898–901. [PubMed: 16208374]
- Kimoto H, Sato K, Nodari F, Haga S, Holy T.E, Touhara K. Sex- and strain-specific expression and vomeronasal activity of mouse ESP family peptides. Curr Biol. 2007;17:1879–1884. [PubMed: 17935991]
- Kiyokawa Y, Kikusui T, Takeuchi Y, Mori Y. Removal of the vomeronasal organ blocks the stress-induced hyperthermia response to alarm pheromone in male rats. Chem Senses. 2007;32:57–64. [PubMed: 17071943]
- Kolunie J.M, Stern J.M. Maternal aggression in rats: Effects of olfactory bulbectomy, ZnSO4-induced anosmia, and vomeronasal organ removal. Horm Behav. 1995;29:492–518. [PubMed: 8748510]
- Krieger J, Schmitt A, Lobel D, Gudermann T, Schultz G, Breer H, Boekhoff I. Selective activation of G protein subtypes in the vomeronasal organ upon stimulation with urine-derived compounds. J Biol Chem. 1999;274:4655–4662. [PubMed: 9988702]
- Kroner C, Breer H, Singer A.G, O’Connell R.J. Pheromone-induced second messenger signaling in the hamster vomeronasal organ. Neuroreport. 1996;7:2989–2992. [PubMed: 9116225]
- Lau Y.E, Cherry J.A. Distribution of PDE4A and G(o) alpha immunoreactivity in the accessory olfactory system of the mouse. Neuroreport. 2000;11:27–32. [PubMed: 10683824]
- Le Y, Murphy P.M, Wang J.M. Formyl-peptide receptors revisited. Trends Immunol. 2002;23:541–548. [PubMed: 12401407]
- Lee S.J, Mammen A, Kim E.J, Kim S.Y, Park Y.J, Park M, Han H.S, Bae Y.C, Ronnett G.V, Moon C. The vomeronasal organ and adjacent glands express components of signaling cascades found in sensory neurons in the main olfactory system. Mol Cells. 2008;26:503–513. [PubMed: 18711317]
- Leinders-Zufall T, Brennan P, Widmayer P, S P.C, Maul-Pavicic A, Jager M, Li X.H, Breer H, Zufall F, Boehm T. MHC class I peptides as chemosensory signals in the vomeronasal organ. Science. 2004;306:1033–1037. [PubMed: 15528444]
- Leinders-Zufall T, Ishii T, Mombaerts P, Zufall F, Boehm T. Structural requirements for the activation of vomeronasal sensory neurons by MHC peptides. Nat Neurosci. 2009;12:1551–1558. [PubMed: 19935653]
- Leinders-Zufall T, Lane A.P, Puche A.C, Ma W, Novotny M.V, Shipley M.T, Zufall F. Ultrasensitive pheromone detection by mammalian vomeronasal neurons. Nature. 2000;405:792–796. [PubMed: 10866200]
- Leypold B.G, Yu C.R, Leinders-Zufall T, Kim M.M, Zufall F, Axel R. Altered sexual and social behaviors in trp2 mutant mice. Proc Natl Acad Sci U S A. 2002;99:6376–6381. [PMC free article: PMC122956] [PubMed: 11972034]
- Liberles S.D, Horowitz L.F, Kuang D, Contos J.J, Wilson K.L, Siltberg-Liberles J, Liberles D.A, Buck L.B. Formyl peptide receptors are candidate chemosensory receptors in the vomeronasal organ. Proc Natl Acad Sci U S A. 2009;106:9842–9847. [PMC free article: PMC2690606] [PubMed: 19497865]
- Liman E.R. Regulation by voltage and adenine nucleotides of a Ca2+-activated cation channel from hamster vomeronasal sensory neurons. J Physiol. 2003;548:777–787. [PMC free article: PMC2342889] [PubMed: 12640014]
- Liman E.R, Innan H. Relaxed selective pressure on an essential component of pheromone transduction in primate evolution. Proc Natl Acad Sci U S A. 2003;100:3328–3332. [PMC free article: PMC152292] [PubMed: 12631698]
- Liman E.R, Corey D.P, Dulac C. TRP2: A candidate transduction channel for mammalian pheromone sensory signaling. Proc Natl Acad Sci U S A. 1999;96:5791–5796. [PMC free article: PMC21939] [PubMed: 10318963]
- Loconto J, Papes F, Chang E, Stowers L, Jones E.P, Takada T, Kumanovics A, Fischer Lindahl K, Dulac C. Functional expression of murine V2R pheromone receptors involves selective association with the M10 and M1 families of MHC class Ib molecules. Cell. 2003;112:607–618. [PubMed: 12628182]
- Logan D.W, Marton T.F, Stowers L. Species specificity in major urinary proteins by parallel evolution. PloS One. 2008;3:e3280. [PMC free article: PMC2533699] [PubMed: 18815613]
- Lucas P, Ukhanov K, Leinders-Zufall T, Zufall F. A diacylglycerol-gated cation channel in vomeronasal neuron dendrites is impaired in TRPC2 mutant mice: Mechanism of pheromone transduction. Neuron. 2003;40:551–561. [PubMed: 14642279]
- Luo H, Arndt W, Zhang Y, Shi G, Alekseyev M.A, Tang J, Hughes A.L, Friedman R. Phylogenetic analysis of genome rearrangements among five mammalian or-ders. Mol Phylogenet Evol. 2012;65:871–882. [PMC free article: PMC4425404] [PubMed: 22929217]
- Ma M. Encoding olfactory signals via multiple chemosensory systems. Crit Rev Biochem Mol Biol. 2007;42:463–480. [PubMed: 18066954]
- Martinez-Marcos A. On the organization of olfactory and vomeronasal cortices. Prog Neurobiol. 2009;87:21–30. [PubMed: 18929620]
- Martini S, Silvotti L, Shirazi A, Ryba N.J, Tirindelli R. Co-expression of putative pheromone receptors in the sensory neurons of the vomeronasal organ. J Neurosci. 2001;21:843–848. [PMC free article: PMC6762303] [PubMed: 11157070]
- Matsunami H, Buck L.B. A multigene family encoding a diverse array of putative pheromone receptors in mammals. Cell. 1997;90:775–784. [PubMed: 9288756]
- Menco B.P, Carr V.M, Ezeh P.I, Liman E.R, Yankova M.P. Ultrastructural localization of G-proteins and the channel protein TRP2 to microvilli of rat vomeronasal receptor cells. J. Comp Neurol. 2001;438:468–489. [PubMed: 11559902]
- Michaux J, Reyes A, Catzeflis F. Evolutionary history of the most speciose mammals: Molecular phylogeny of muroid rodents. Mol Biol Evol. 2001;18:2017–2031. [PubMed: 11606698]
- Migeotte I, Communi D, Parmentier M. Formyl peptide receptors: A promiscuous subfamily of G protein-coupled receptors controlling immune responses. Cytokine Growth Factor Rev. 2006;17:501–519. [PubMed: 17084101]
- Mucignat-Caretta C, Caretta A, Cavaggioni A. Acceleration of puberty onset in female mice by male urinary proteins. J Physiol. 1995;486 (Pt 2):517–522. [PMC free article: PMC1156539] [PubMed: 7473215]
- Mundy N.I, Cook S. Positive selection during the diversification of class I vomeronasal receptor-like (V1RL) genes, putative pheromone receptor genes, in human and primate evolution. Mol Biol Evol. 2003;20:1805–1810. [PubMed: 12832635]
- Nei M, Niimura Y, Nozawa M. The evolution of animal chemosensory receptor gene repertoires: Roles of chance and necessity. Nat Rev Genet. 2008;9:951–963. [PubMed: 19002141]
- Nikolaev S, Montoya-Burgos J.I, Margulies E.H, Rougemont J, Nyffeler B, Antonarakis S.E. Early history of mammals is elucidated with the ENCODE multiple species sequencing data. PLoS Genet. 2007;3:e2. [PMC free article: PMC1761045] [PubMed: 17206863]
- Nodari F, Hsu F.F, Fu X, Holekamp T.F, Kao L.F, Turk J, Holy T.E. Sulfated steroids as natural ligands of mouse pheromone-sensing neurons. J Neurosci. 2008;28:6407–6418. [PMC free article: PMC2726112] [PubMed: 18562612]
- Norlin E.M, Gussing F, Berghard A. Vomeronasal phenotype and behavioral alterations in G alpha i2 mutant mice. Curr Biol. 2003;13:1214–1219. [PubMed: 12867032]
- Novotny M.V, Jemiolo B, Wiesler D, Ma W, Harvey S, Xu F, Xie T.M, Carmack M. A unique urinary constituent, 6-hydroxy-6-methyl-3-heptanone, is a pheromone that accelerates puberty in female mice. Chem Biol. 1999;6:377–383. [PubMed: 10375540]
- Ohara H, Nikaido M, Date-Ito A, Mogi K, Okamura H, Okada N, Takeuchi Y, Mori Y, Hagino-Yamagishi K. Conserved repertoire of orthologous vomeronasal type 1 receptor genes in ruminant species. BMC Evol Biol. 2009;9:233. [PMC free article: PMC2758851] [PubMed: 19751533]
- Papes F, Logan D.W, Stowers L. The vomeronasal organ mediates interspecies defensive behaviors through detection of protein pheromone homologs. Cell. 2010;141:692–703. [PMC free article: PMC2873972] [PubMed: 20478258]
- Park S.H, Podlaha O, Grus W.E, Zhang J. The microevolution of V1r vomeronasal receptor genes in mice. Genome Biol Evol. 2011;3:401–412. [PMC free article: PMC3114644] [PubMed: 21551350]
- Pfister P, Randall J, Montoya-Burgos J.I, Rodriguez I. Divergent evolution among teleost V1r receptor genes. PloS One. 2007;2:e379. [PMC free article: PMC1849887] [PubMed: 17440615]
- Pifferi S, Cenedese V, Menini A. Anoctamin 2/TMEM16B: A calcium-activated chloride channel in olfactory transduction. Exp Physiol. 2012;97:193–199. [PubMed: 21890523]
- Pifferi S, Dibattista M, Menini A. TMEM16B induces chloride currents activated by calcium in mammalian cells. Pflugers Arch. 2009;458:1023–1038. [PubMed: 19475416]
- Rasche S, Toetter B, Adler J, Tschapek A, Doerner J.F, Kurtenbach S, Hatt H, Meyer H, Warscheid B, Neuhaus E.M. Tmem16b is specifically expressed in the cilia of olfactory sensory neurons. Chem Senses. 2010;35:239–245. [PubMed: 20100788]
- Riviere S, Challet L, Fluegge D, Spehr M, Rodriguez I. Formyl peptide receptor-like proteins are a novel family of vomeronasal chemosensors. Nature. 2009;459:574–577. [PubMed: 19387439]
- Roberts S.A, Simpson D.M, Armstrong S.D, Davidson A.J, Robertson D.H, McLean L, Beynon R.J, Hurst J.L. Darcin: A male pheromone that stimulates female memory and sexual attraction to an individual male’s odour. BMC Biol. 2010;8:75. [PMC free article: PMC2890510] [PubMed: 20525243]
- Rodriguez I. Pheromone receptors in mammals. Horm Behav. 2004;46:219–230. [PubMed: 15325223]
- Rodriguez I, Mombaerts P. Novel human vomeronasal receptor-like genes reveal species-specific families. Curr Biol. 2002;12:R409–411. [PubMed: 12123587]
- Rodriguez I, Del Punta K, Rothman A, Ishii T, Mombaerts P. Multiple new and isolated families within the mouse superfamily of V1r vomeronasal receptors. Nat Neurosci. 2002;5:134–140. [PubMed: 11802169]
- Rodriguez I, Feinstein P, Mombaerts P. Variable patterns of axonal projections of sensory neurons in the mouse vomeronasal system. Cell. 1999;97:199–208. [PubMed: 10219241]
- Roppolo D, Ribaud V, Jungo V.P, Luscher C, Rodriguez I. Projection of the Gruneberg ganglion to the mouse olfactory bulb. Eur J Neurosci. 2006;23:2887–2894. [PubMed: 16819977]
- Rossler P, Kroner C, Krieger J, Lobel D, Breer H, Boekhoff I. Cyclic adenosine monophosphate signaling in the rat vomeronasal organ: Role of an adenylyl cyclase type VI. Chem Senses. 2000;25:313–322. [PubMed: 10866989]
- Runnenburger K, Breer H, Boekhoff I. Selective G protein beta gamma-subunit compositions mediate phospholipase C activation in the vomeronasal organ. Eur J Cell Biol. 2002;81:539–547. [PubMed: 12437188]
- Ryba N.J, Tirindelli R. A novel GTP-binding protein gamma-subunit, G gamma 8, is expressed during neurogenesis in the olfactory and vomeronasal neuroepithelia. J Biol Chem. 1995;270:6757–6767. [PubMed: 7896821]
- Ryba N.J, Tirindelli R. A new multigene family of putative pheromone receptors. Neuron. 1997;19:371–379. [PubMed: 9292726]
- Ryu S.H, Kwak M.J, Hwang U.W. Complete mitochondrial genome of the Eurasian flying squirrel Pteromys volans (Sciuromorpha, Sciuridae) and revision of rodent phylogeny. Mol Biol Rep. 2013;40:1917–1926. [PubMed: 23114915]
- Saraiva L.R, Korsching S.I. A novel olfactory receptor gene family in teleost fish. Genome Res. 2007;17:1448–1457. [PMC free article: PMC1987349] [PubMed: 17717047]
- Sasaki K, Okamoto K, Inamura K, Tokumitsu Y, Kashiwayanagi M. Inositol-1,4,5-trisphosphate accumulation induced by urinary pheromones in female rat vomeronasal epithelium. Brain Res. 1999;823:161–168. [PubMed: 10095022]
- Schroeder B.C, Cheng T, Jan Y.N, Jan L.Y. Expression cloning of TMEM16A as a calcium-activated chloride channel subunit. Cell. 2008;134:1019–1029. [PMC free article: PMC2651354] [PubMed: 18805094]
- Scudieri P, Sondo E, Ferrera L, Galietta L.J.V. The anoctamin family: TMEM16A and TMEM16B as calcium-activated chloride channels. Exp Physiol. 2012;97:177–183. [PubMed: 21984732]
- Seifert R, Wenzel-Seifert K. The human formyl peptide receptor as model system for constitutively active G-protein-coupled receptors. Life Sci. 2003;73:2263–2280. [PubMed: 12941430]
- Serizawa S, Ishii T, Nakatani H, Tsuboi A, Nagawa F, Asano M, Sudo K, Sakagami J, Sakano H, Ijiri T, Matsuda Y, Suzuki M, Yamamori T, Iwakura Y. Mutually exclusive expression of odorant receptor transgenes. Nat Neurosci. 2000;3:687–693. [PubMed: 10862701]
- Shi P, Zhang J. Comparative genomic analysis identifies an evolutionary shift of vomeronasal receptor gene repertoires in the vertebrate transition from water to land. Genome Res. 2007;17:166–174. [PMC free article: PMC1781348] [PubMed: 17210926]
- Shi P, Bielawski J.P, Yang H, Zhang Y.P. Adaptive diversification of vomeronasal receptor 1 genes in rodents. J Mol Evol. 2005;60:566–576. [PubMed: 15983866]
- Shimazaki R, Boccaccio A, Mazzatenta A, Pinato G, Migliore M, Menini A. Electrophysiological properties and modeling of murine vomeronasal sensory neurons in acute slice preparations. Chem Senses. 2006;31:425–435. [PubMed: 16547196]
- Silvotti L, Cavalca E, Gatti R, Percudani R, Tirindelli R. A recent class of chemosensory neurons developed in mouse and rat. PloS One. 2011;6:e24462. [PMC free article: PMC3170373] [PubMed: 21931725]
- Silvotti L, Giannini G, Tirindelli R. The vomeronasal receptor V2R2 does not require escort molecules for expression in heterologous systems. Chem Senses. 2005;30:1–8. [PubMed: 15647459]
- Silvotti L, Moiani A, Gatti R, Tirindelli R. Combinatorial co-expression of pheromone receptors, V2Rs. J Neurochem. 2007;103:1753–1763. [PubMed: 17854397]
- Singer A.G, Macrides F, Clancy A.N, Agosta W.C. Purification and analysis of a proteinaceous aphrodisiac pheromone from hamster vaginal discharge. J Biol Chem. 1986;261:13323–13326. [PubMed: 3759967]
- Smith T.D, Bhatnagar K.P, Burrows A.M, Shimp K.L, Dennis J.C, Smith M.A, Maico-Tan L, Morrison E.E. The vomeronasal organ of greater bushbabies (Otolemur spp.): Species, sex, and age differences. J Neurocytol. 2005;34:135–147. [PubMed: 16374715]
- Spehr M, Hatt H, Wetzel C.H. Arachidonic acid plays a role in rat vomeronasal signal transduction. J Neurosci. 2002;22:8429–8437. [PMC free article: PMC6757791] [PubMed: 12351717]
- Stephan A.B, Shum E.Y, Hirsh S, Cygnar K.D, Reisert J, Zhao H. ANO2 is the cilial calcium-activated chloride channel that may mediate olfactory amplification. Proc Natl Acad Sci U S A. 2009;106:11776–11781. [PMC free article: PMC2702256] [PubMed: 19561302]
- Stöhr H, Heisig J.B, Benz P.M, Schöberl S, Milenkovic V.M, Strauss O, Aartsen W.M, Wijnholds J, Weber B.H.F, Schulz H.L. TMEM16B, a novel protein with calcium-dependent chloride channel activity, associates with a presynaptic protein complex in photoreceptor terminals. J Neurosci. 2009;29:6809–6818. [PMC free article: PMC6665584] [PubMed: 19474308]
- Stowers L, Holy T.E, Meister M, Dulac C, Koentges G. Loss of sex discrimination and male-male aggression in mice deficient for TRP2. Science. 2002;295:1493–1500. [PubMed: 11823606]
- Sturm T, Leinders-Zufall T, Macek B, Walzer M, Jung S, Pommerl B, Stevanovic S, Zufall F, Overath P, Rammensee H.G. Mouse urinary peptides provide a molecular basis for genotype discrimination by nasal sensory neurons. Nat Commun. 2013;4:1616. [PubMed: 23511480]
- Syed A.S, Sansone A, Nadler W, Manzini I, Korsching S.I. Ancestral amphibian v2rs are expressed in the main olfactory epithelium. Proc Natl Acad Sci U S A. 2013;110:7714–7719. [PMC free article: PMC3651509] [PubMed: 23613591]
- Takami S. Recent progress in the neurobiology of the vomeronasal organ. Microsc Res Tech. 2002;58:228–250. [PubMed: 12203701]
- Takano T, Fiore S, Maddox J.F, Brady H.R, Petasis N.A, Serhan C.N. Aspirin-triggered 15-epilipoxin A4 (LXA4) and LXA4 stable analogues are potent inhibitors of acute inflammation: Evidence for anti-inflammatory receptors. J Exp Med. 1997;185:1693–1704. [PMC free article: PMC2196289] [PubMed: 9151906]
- Takigami S, Mori Y, Ichikawa M. Projection pattern of vomeronasal neurons to the accessory olfactory bulb in goats. Chem Senses. 2000;25:387–393. [PubMed: 10944501]
- Tanaka M, Treloar H, Kalb R.G, Greer C.A, Strittmatter S.M. G(o) protein-dependent survival of primary accessory olfactory neurons. Proc Natl Acad Sci U S A. 1999;96:14106–14111. [PMC free article: PMC24198] [PubMed: 10570206]
- Thompson R.N, McMillon R, Napier A, Wekesa K.S. Pregnancy block by MHC class I peptides is mediated via the production of inositol 1,4,5-trisphosphate in the mouse vomeronasal organ. J Exp Biol. 2007;210:1406–1412. [PubMed: 17401123]
- Thompson R.N, Robertson B.K, Napier A, Wekesa K.S. Sex-specific responses to urinary chemicals by the mouse vomeronasal organ. Chem Senses. 2004;29:749–754. [PubMed: 15574810]
- Thoren F.B, Karlsson J, Dahlgren C, Forsman H. The anionic amphiphile SDS is an antagonist for the human neutrophil formyl peptide receptor 1. Biochem Pharmacol. 2010;80:389–395. [PubMed: 20394736]
- Tirindelli R, Ryba N.J. The G-protein gamma-subunit G gamma 8 is expressed in the developing axons of olfactory and vomeronasal neurons. Eur J Neurosci. 1996;8:2388–2398. [PubMed: 8950102]
- Tirindelli R, Dibattista M, Pifferi S, Menini A. From pheromones to behavior. Physiol Rev. 2009;89:921–956. [PubMed: 19584317]
- Wakabayashi Y, Mori Y, Ichikawa M, Yazaki K, Hagino-Yamagishi K. A putative pheromone receptor gene is expressed in two distinct olfactory organs in goats. Chem Senses. 2002;27:207–213. [PubMed: 11923183]
- Wang G, Shi P, Zhu Z, Zhang Y.P. More functional V1R genes occur in nest-living and nocturnal terricolous mammals. Genome Biol Evol. 2010;2:277–283. [PMC free article: PMC2997545] [PubMed: 20624732]
- Wu Y, Tirindelli R, Ryba N.J. Evidence for different chemosensory signal transduction pathways in olfactory and vomeronasal neurons. Biochem Biophys Res Commun. 1996;220:900–904. [PubMed: 8607864]
- Wysocki C.J, Lepri J.J. Consequences of removing the vomeronasal organ. J Steroid Biochem Mol Biol. 1991;39:661–669. [PubMed: 1892795]
- Wyckoff G.J, Wang W, Wu C.I. Rapid evolution of male reproductive genes in the descent of man. Nature. 2000;403:304–309. [PubMed: 10659848]
- Yang C, Delay R.J. Calcium-activated chloride current amplifies the response to urine in mouse vomeronasal sensory neurons. J Gen Physiol. 2010;135:3–13. [PMC free article: PMC2806418] [PubMed: 20038523]
- Yang H, Shi P. Molecular and evolutionary analyses of formyl peptide receptors suggest the absence of VNO-specific FPRs in primates. J Genet Genomics. 2010;37:771–778. [PubMed: 21193155]
- Yang H, Shi P, Zhang Y.P, Zhang J. Composition and evolution of the V2r vomeronasal receptor gene repertoire in mice and rats. Genomics. 2005;86:306–315. [PubMed: 16024217]
- Yang Y.D, Cho H, Koo J.Y, Tak M.H, Cho Y, Shim W.-S, Park S.P, Lee J, Lee B, Kim B.-M, Raouf R, Shin Y.K, Oh U. TMEM16A confers receptor-activated calcium-dependent chloride conductance. Nature. 2008;455:1210–1215. [PubMed: 18724360]
- Yau K.W, Hardie R.C. Phototransduction motifs and variations. Cell. 2009;139:246–264. [PMC free article: PMC2885920] [PubMed: 19837030]
- Yoon H, Enquist L.W, Dulac C. Olfactory inputs to hypothalamic neurons controlling reproduction and fertility. Cell. 2005;123:669–682. [PubMed: 16290037]
- Young J.M, Trask B.J. V2R gene families degenerated in primates, dog and cow, but expanded in opossum. Trends Genet. 2007;23:212–215. [PubMed: 17382427]
- Young J.M, Kambere M, Trask B.J, Lane R.P. Divergent V1R repertoires in five species: Amplification in rodents, decimation in primates, and a surprisingly small repertoire in dogs. Genome Res. 2005;15:231–240. [PMC free article: PMC546524] [PubMed: 15653832]
- Young J.M, Massa H.F, Hsu L, Trask B.J. Extreme variability among mammalian V1R gene families. Genome Res. 2010;20:10–18. [PMC free article: PMC2798821] [PubMed: 19952141]
- Zhang J, Webb D.M. Evolutionary deterioration of the vomeronasal pheromone transduction pathway in catarrhine primates. Proc Natl Acad Sci U S A. 2003;100:8337–8341. [PMC free article: PMC166230] [PubMed: 12826614]
- Zhang J, Webb D.M. Rapid evolution of primate antiviral enzyme APOBEC3G. Hum Mol Genet. 2004;13:1785–1791. [PubMed: 15198990]
- Zhang P, Yang C, Delay R.J. Urine stimulation activates BK channels in mouse vomeronasal neurons. J Neurophysiol. 2008;100:1824–1834. [PMC free article: PMC2576191] [PubMed: 18701755]
- Zhang P, Yang C, Delay R.J. Odors activate dual pathways, a TRPC2 and a AA-dependent pathway, in mouse vomeronasal neurons. Am J Physiol. 2010;298:C1253–1264. [PMC free article: PMC2867386] [PubMed: 20147653]
- Zhao H, Xu D, Zhang S, Zhang J. Widespread losses of vomeronasal signal transduction in bats. Mol Biol Evol. 2011;28:7–12. [PMC free article: PMC3108603] [PubMed: 20693241]
- Zhou A, Moss R.L. Effect of urine-derived compounds on cAMP accumulation in mouse vomeronasal cells. Neuroreport. 1997;8:2173–2177. [PubMed: 9243606]
- Review Multiple Olfactory Subsystems Convey Various Sensory Signals.[The Neurobiology of Olfaction....]Review Multiple Olfactory Subsystems Convey Various Sensory Signals.Ma M. The Neurobiology of Olfaction. 2010
- Subclasses of vomeronasal receptor neurons: differential expression of G proteins (Gi alpha 2 and G(o alpha)) and segregated projections to the accessory olfactory bulb.[Brain Res. 1996]Subclasses of vomeronasal receptor neurons: differential expression of G proteins (Gi alpha 2 and G(o alpha)) and segregated projections to the accessory olfactory bulb.Jia C, Halpern M. Brain Res. 1996 May 6; 719(1-2):117-28.
- Review Influence of Cat Odor on Reproductive Behavior and Physiology in the House Mouse: (Mus Musculus).[Neurobiology of Chemical Commu...]Review Influence of Cat Odor on Reproductive Behavior and Physiology in the House Mouse: (Mus Musculus).Voznessenskaya VV. Neurobiology of Chemical Communication. 2014
- Cells in the vomeronasal organ express odorant receptors but project to the accessory olfactory bulb.[J Comp Neurol. 2006]Cells in the vomeronasal organ express odorant receptors but project to the accessory olfactory bulb.Lévai O, Feistel T, Breer H, Strotmann J. J Comp Neurol. 2006 Oct 1; 498(4):476-90.
- Review Molecular and Neural Mechanisms of Pheromone Reception in the Rat Vomeronasal System and Changes in the Pheromonal Reception by the Maturation and Sexual Experiences.[Neurobiology of Chemical Commu...]Review Molecular and Neural Mechanisms of Pheromone Reception in the Rat Vomeronasal System and Changes in the Pheromonal Reception by the Maturation and Sexual Experiences.Kashiwayanagi M. Neurobiology of Chemical Communication. 2014
- Vomeronasal Receptors and Signal Transduction in the Vomeronasal Organ of Mammal...Vomeronasal Receptors and Signal Transduction in the Vomeronasal Organ of Mammals - Neurobiology of Chemical Communication
Your browsing activity is empty.
Activity recording is turned off.
See more...