Open Access: This content is Open Access under the Creative Commons license CC-BY-NC-ND.
NCBI Bookshelf. A service of the National Library of Medicine, National Institutes of Health.
Mattick J, Amaral P. RNA, the Epicenter of Genetic Information: A new understanding of molecular biology. Abingdon (UK): CRC Press; 2022 Sep 20. doi: 10.1201/9781003109242-12
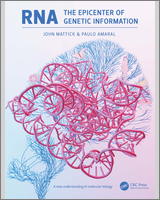
RNA, the Epicenter of Genetic Information: A new understanding of molecular biology.
Show detailsOdd genetic observations in the 1980s and 1990s indicated that RNA may play a general role in regulating gene expression, when it was reported that both sense and antisense RNAs could modulate gene expression transcriptionally and post-transcriptionally, referred to as ‘co-suppression’, ‘gene silencing’ or ‘RNA interference’ (RNAi). The finding that introducing sense and antisense RNAs together resulted in systemic silencing of target genes led to the dissection of the RNAi pathways, showing that dsRNA precursors are processed to form small RNAs that guide DNA methylation and cleavage of orthologous sequences in mRNAs. It was soon discovered that these pathways are used extensively to control gene expression during differentiation and development via ‘microRNAs’ (miRNAs) derived from introns and other non-protein-coding transcripts, the numbers of which increased during animal evolution. Similar regulatory RNA pathways were characterized in plants. Related small RNAs, ‘piRNAs’, were found to be required for germ and stem cell development in animals, some of which are produced from transposon-derived sequences. Other classes of small regulatory RNAs were found to be generated from tRNAs, rRNAs, snoRNAs, snRNAs, gene promoters and splice junctions, and small RNAs were shown to mediate intergenerational and interspecies communication. A related pathway, termed ‘CRISPR’, was found to target cleavage of bacteriophage genomes, manipulation of which has revolutionized genetic analysis and genetic engineering. Both CRISPR and RNAi use RNAs to guide generic effector proteins sequence-specifically to target RNAs and DNAs, a highly efficient and flexible system of gene control.
At the same time as the genome projects were getting into full swing, another revolution was underway, which brought into light the natural capacity of RNA to bind other RNAs and DNA sequence-specifically to combat viruses and to regulate gene expression, 1 , 2 as first proposed decades earlier (Chapters 3, 5 and 9).
Such logical capacity expands enormously the range of regulatory options by using RNA to address different targets and execute specific actions using a generic infrastructure of guidable protein effectors. This is a highly efficient and flexible system of gene control, which has opened up new avenues for gene modulation and genetic engineering. It also lies at the heart of the epigenetic control of gene expression during multicellular differentiation and development (Chapter 16).
Unusual Genetic Phenomena Involving RNA
The demonstration that RNAs can use base pairing to form double-stranded structures dates back to 1956. 3 In the 1960s and 1970s, numerous reports showed the existence of double-stranded RNAs (dsRNAs) derived from viral RNA genomes replicating in eukaryotic cells. It had also been known since the 1960s that synthetic and naturally occurring viral, bacterial and fungal dsRNAs can induce antiviral cytokines called ‘interferons’ a and inhibit protein synthesis in animal cells at low concentrations, an important feature of innate immunity. 8–15 The first candidate RNA drug (reported in 1967) was a fungal extract that contained “an anti-viral agent … [which] is an RNA of viral origin”. 10 , 15 , 16
The discovery of interferons and their induction by dsRNAs explained, at least in part, a phenomenon known since the 1920s as ‘virus interference’, 4 whereby viral infection results in resistance to subsequent infections by the same or related viruses. 17 , 18 The mechanism was subsequently shown to involve two interferon-induced, dsRNA-dependent enzymes that inhibit translation and activate an endoribonuclease. 19 , 20 In 1985, Masayori Inouye and colleagues developed an antisense platform (‘micRNA’) to regulate host gene expression, which has “great potential as a novel cellular immune system for blocking bacteriophage or virus infection”, 21 with Sergio Giunta and Giuseppe Groppa suggesting that
Upon virus infection and exposure to viral nucleic acids, the cell synthesizes micRNAs that … constitute some of the dsRNAs that are involved both in interferon induction and in the cellular metabolic pathways activated by interferon … and may also be preferentially cleaved by cellular enzymes which would account for the still unexplained discrimination between cleavage of cellular and viral mRNAs in interferon treated cells. 22
presaging both the mechanism and the sensing of foreign nucleic acids by the Toll pathway. b
In the late 1980s, Andrew Fire, Craig Mello and others showed that the introduction of DNA constructs containing fragments of genes in C. elegans caused suppression of the endogenous homologous gene. 32 As expected, this silencing was observed when constructs were designed to drive antisense RNA transcription. 33 Surprisingly, however, silencing was also observed when designed to drive ‘sense’ gene expression, which was expected to do the opposite, i.e., increase the mRNA levels. 33 Adding to this puzzle, in 1995, Su Guo and Ken Kemphues obtained the same result by directly introducing in vitro transcribed RNAs in either the sense or antisense orientation, which caused ‘silencing’ of the corresponding gene in C. elegans. 34
Similar silencing effects in plants and fungi were also reported in the 1990s, 35 when it was established that the presence of multiple copies of introduced transgenes resulted in repression not only of the transgenes, but also of the homologous endogenous genes. c
The iconic example is flower color in petunia, where the effect of silencing of endogenous loci by antisense RNAs was already well established, as famously illustrated a decade earlier by the changes of pigmentation in transgenic petunia flowers expressing antisense RNAs targeting genes specifying the anthocyanin biogenesis pathway, 39–42 which appeared to result from “increased RNA turnover”. 43
In 1990, two groups did the opposite experiment but unexpectedly obtained the same result – they overexpressed a pigment synthesis gene hoping to produce deep purple petunia flowers, but instead obtained predominantly white flowers, with remarkable mosaic patterns, including one dubbed “The Cossack Dancer” (Figure 12.1). 41 , 44 , 45

Figure 12.1
Homology-dependent gene silencing. The ‘Cossack Dancer' variegated pattern in transgenic petunia expressing a chimeric chalcone synthase pigmentation gene resulting in co-suppression of the endogenous genes. (Reproduced from Napoli et al. with (more...)
These phenomena were variously termed ‘co-suppression’, ‘homology-dependent gene silencing’, ‘transgene silencing’ (‘quelling’ in fungi) 35 , 46–49 or ‘RNA-mediated interference’ (RNAi), 50 and later shown also to occur in Drosophila. 51 , 52
Despite being initially regarded as a peculiar phenomenon, as early as in 1986 ‘co-suppression’ began to be used as a biotechnological tool to modulate plant phenotypes, 36 , 53–55 only a few years after the first transgenic plants had been constructed by the introduction of foreign genes. 56 Similar to the puzzling RNA interference observed in worms, it was suggested that the silencing caused by introduced copies of ‘sense’ transgenes in plants 44 , 57 , 58 might be triggered by “unexpected” promoter activity in the antisense orientation. 59 , 60 Nevertheless, this remained a matter of discussion, as the mechanisms behind these phenomena were unknown and appeared to be heterogeneous. 60
Several models involving RNA-RNA, RNA-DNA and DNA-DNA pairing interactions (or RNA-DNA triplexes 45 ) were proposed and investigated by the pioneering groups, those of Michael Wassenegger, Marjori and Antonius Matzke, David Baulcombe, Jan Kooter and others. 48 , 61–65 In some cases, silencing appeared to be caused by epigenetic mechanisms; in particular sequence-specific RNA-directed DNA methylation 48 , 65–69 (see below and Chapter 14), which was termed ‘transcriptional gene silencing’ (TGS).
Other evidence suggested that antisense RNAs interfered with translation or directed the degradation of the target mRNA via dsRNA formation, 43 , 54 , 58 , 70 termed ‘post-transcriptional gene silencing’ (PTGS), without a supported idea of how ‘sense’ RNAs might result in the same outcome, or whether there may be more than one RNA-mediated pathway, although there was evidence that TGS and PTGS are mechanistically related. 71 , 72
The finding that the silencing of specific genes was able to spread systemically in plants indicated the participation of a diffusible molecule, 73–76 likely RNA itself, 47 , 54 , 64 , 77 , 78 with the prediction that there existed a “sequence-specific signaling mechanism in plants that may have roles in developmental control as well as in protection against transposons and viruses”. 76
In 1999, Baulcombe and Andrew Hamilton, considering the lack of evidence for long antisense RNAs, hypothesized that low molecular weight RNAs might be involved but had escaped detection due to their small size. They showed that short antisense RNAs (estimated to be ∼25nt) are produced in transgenic or virus-infected plants undergoing PTGS when both antisense and target mRNAs (cellular or viral) are expressed. They proposed that these RNAs acted as guides for PTGS, stressing that small RNAs “are long enough to convey sequence specificity yet small enough to move through plasmodesmata”, and are thus able to act as “the systemic signal and specificity determinants of PTGS” (Figure 12.2). 79

Figure 12.2
The identification of small sense and antisense RNAs in post-transcriptional gene silencing in plants. (Reproduced from Hamilton and Baulcombe with permission of the American Association for the Advancement of Science.) The left panel shows that transgenic (more...)
The RNA Interference Pathway
In 1998, Andrew Fire, Craig Mello and co-workers resolved the sense-antisense conundrum by showing (inadvertently, as part of an experimental control) that addition of sense and antisense RNAs together potently triggered systemic and heritable gene silencing in C. elegans, which “was at least two orders of magnitude more effective than either single strand alone”. 81 Although the mechanism of silencing was still unknown, these observations explained the RNAi phenomenon, suggesting that the previous silencing observed with ‘sense’ RNAs was due to contaminating dsRNAs formed during in vitro RNA synthesis and antisense transcription.
Independently, also in 1998, Peter Waterhouse and colleagues showed that gene silencing and transgene-mediated virus resistance in plants were induced and targeted by dsRNAs, 82 and Elisabetta Ullu and colleagues showed that dsRNA induced mRNA degradation in trypanosomes. 83 It was soon also demonstrated that dsRNAs trigger gene silencing in Drosophila 84–87 and mammalian cells. 88–90
These findings were followed by genetic dissection of the biochemical pathways and proteins involved. 91 , 92 The first identified was an RNA-dependent RNA polymerase (RdRP) named qde-1 (for quelling defective mutant 1), which had been predicted to participate in RNA silencing (as, by definition, they would produce dsRNAs). 93 , 94 Although RdRPs had been detected in prokaryotes and eukaryotes decades before, mostly associated with RNA virus replication, RdRP activity had also been described in tissues of “apparently healthy plants” whose product seemed to be “double-helical RNA”, 95 with other reports showing the existence of non-viral RdRPs. 96 , 97 In 1999, Carlo Cogoni and Giuseppe Macino showed that RdRP was involved in gene silencing, 94 with others subsequently showing that homologs are present not only in fungi, but also in many plants and nematodes, d indicating a common pathway. 101–104
Many other components of the ‘RNAi machinery’ were soon identified and shown to occur in plants, invertebrates and vertebrates. 92 , 105 To cut another long story short, work from the laboratories of David Bartel, Greg Hannon, Thomas Tuschl, Phil Zamore and others showed that long dsRNA is recognized by dsRNA-binding proteins (of which there are many versions) that interact with and activate an enzyme named Dicer e to cleave the bound RNA into short dsRNA fragments of ~20–23nt in length (which can be amplified by RdRP, hence making RNAi auto-catalytic), 91 , 101 , 104 , 107–109 as had been proposed previously, 110 explaining the powerful and relatively stable systemic and transgenerational silencing obtained with small initial amounts of RNAs.
The short dsRNA fragments, called siRNAs (small interfering RNAs), 89 are then unwound and one of the two strands (the ‘guide strand’, determined by the strength of the hydrogen bond interaction at the 5′-ends of the RNA duplex, known as the ‘asymmetry rule’) is loaded into an effector complex, the ‘RNA-induced silencing complex’ (RISC). The guide strand pairs with a complementary sequence in an mRNA (or other target RNA molecule) and induces its cleavage by an ‘Argonaute’ f protein, the catalytic component of the RISC. 89 , 107–109 , 113
There is a large family of Argonaute proteins 114 , 115 and a range of small RNA signaling pathways, especially in plants. 116–118 In eukaryotes, Argonaute proteins are found both in the cytoplasm, where they are involved in target mRNA degradation, whereas others are nuclear. 119 Argonaute orthologs also occur in prokaryotes, 114 where they chop foreign plasmids or phage DNA (or transcribed sequences from foreign DNA) g into small (13–25 nt) guide sequences, which then target and cleave complementary DNA, 120–124 indicating that RNA-guided targeting of genes for viral defense and gene regulation dates back to the dawn of biology. h This system is similar to but distinct from bacterial and archaeal CRISPR-Cas systems that also derive guides from foreign DNA (see below), although some CRISPR-Cas loci encode Argonaute proteins that associate with RNA guides, suggesting that the two mechanisms work synergistically in some organisms. 120 , 124
Moreover, the identification of highly conserved protein complexes that mediate small regulatory RNA functions is consistent with principles found in RNA control of other processes, such as the actions of snRNAs and snoRNAs in eukaryotes (Chapter 8), and sRNAs in bacteria, where Hfq acts as a general cofactor for antisense RNAs that rely on short stretches of base pairing 127–132 (Chapter 9).
Genetic studies showed that membrane-bound dsRNA-binding proteins are required for systemic transmission of RNAi. 133 There are two such proteins in C. elegans, 134 , 135 and two orthologs in vertebrates, the latter of which transport internalized extracellular dsRNAs from endocytic compartments into the cytoplasm for immune activation. 136 , 137 However, while systemic RNAi transmission is well understood in plants, 138 the extent of and mechanisms for exocrine RNA transport and signaling in mammals i are unclear; in part it appears to involve packaging of small RNAs (including microRNAs – see below) into exosomes and other circulating vesicles, 141–144 reported to alter gene expression in immune and other cells, 145–150 and to influence aging, 151 as well as tumor invasion and metastasis in cancer, 152–154 although there are conflicting reports. 155 , 156
Transcriptional Gene Silencing: RNA-Directed DNA Methylation
Consistent with a nuclear role, there is now considerable evidence that TGS (as distinct from PTGS) imposed by dsRNAs involves methylation of cognate sequences in the genome, 69 , 157–160 which, although at that time best documented in plants, led Wassenegger to predict in 2000 that “RNA-directed DNA methylation is involved in epigenetic gene regulation throughout eukaryotes”, including mammalian X-chromosome inactivation and parental imprinting. 161 This prediction was confirmed in 2004 when Manika Pal-Bhadra, Tatsuo Fukagawa and colleagues showed that RNAi is required for heterochromatin formation in Drosophila and vertebrate cells, 162 , 163 and Kevin Morris and colleagues showed that siRNAs can induce methylation and TGS of homologous loci in mammalian cells, 164 since confirmed by many others 165 and shown to also involve Argonaute proteins. 166–168
The groups of Rob Martienssen, Shiv Grewal, Danesh Moazed, Robin Allshire, Caroline Dean and others showed that RNAi pathways also control many aspects of chromosome structure and dynamics. 169–179 They demonstrated that transposon-dense heterochromatic regions are not transcriptionally inert, but express RNAs with key functions, many of which involve targeting of nascent transcripts and chromosome-associated RNAs by small RNA species. 180 , 181 These include regulation of centromere function, 182–185 meiotic and mitotic chromosome segregation, 169 repression of meiotic genes by targeting nearby retrotransposon-derived repeats, 186 other aspects of meiotic progression including chromosome condensation, 169 rectification of copy number imbalances, 169 genome rearrangements 187 , 188 and chromosome reassembly, 189 , 190 plant flowering time 191 and DNA damage responses from fission yeasts to mammals, 169 , 192 , 193 with interconnections to transcription and splicing. 180 , 181 RNAi pathways are required for transposon silencing, thought to protect the genome against uncontrolled transposition of mobile elements, 194–198 but have been adapted to regulate developmental processes. 186 , 199 , 200
In plants, RNA-directed DNA methylation induces TGS through the production by specialized plant RNA polymerases of 24nt RNAs that are loaded into AGO4, 201–203 which then recruits DNA methyltransferase and directs DNA methylation to regulate plant-pathogen interactions, stress responses and development. 159 , 204–207 Similar pathways operate in animals, although less is known about them. 167 , 169 , 196–198 , 205 The notable common feature is the use of embedded transposon-derived sequences as the targets of locus-specific regulation by RNAs, which may explain, in large part, the widespread presence of such sequences in genomes of higher organisms (Chapters 10 and 16).
The observations in C. elegans and other organisms showed that RNA signals also mediate transgenerational epigenetic inheritance, 81 , 208–211 requiring, inter alia, a nuclear Argonaute protein 212 and RNA modification 213 (Chapter 17).
Research and Biotechnology Applications
The ability of natural or modified small siRNAs, delivered directly or via a vector that produces a ‘small/short hairpin RNA’ (shRNA), to inhibit gene expression specifically and potently in plants and animals has been widely adopted to investigate and modulate gene function in vivo and in vitro.
In mammals, siRNA was first used to ‘knock down’ protein-coding genes in oocytes and newly fertilized zygotes by Florence Wianny and Magdalena Zernicka-Goetz, where the phenotypes observed were similar to those observed with null mutants of the endogenous genes. 88 siRNA-directed gene knockdown was later shown to be generally applicable to mammalian cells 89 and applied to systematically target protein-coding genes in culture to identify those involved in various aspects of cell biology (e.g., 214 ), viral replication (e.g., 215 ) and drug responses or sensitivity (e.g., 216 ).
The attraction of RNAi (or any nucleic acid-based system) for human therapy is substantial, as it flexibly employs a relatively common chemistry to inhibit the expression of target genes, notwithstanding the possibility of off-target effects, which are progressively being addressed. 217 The longstanding problem is in vivo delivery, especially tissue specificity and transport across the blood-brain barrier to treat neurological disorders, 218 as dsRNAs are not easily absorbed except by the liver. 219 The delivery problem has been solved in part by utilizing viruses, notably AAV (adenovirus-associated virus, because of its low immunogenicity, many tissue-specific serotypes, and low rate of chromosomal integration), as well as other approaches and vehicles such as artificial exosomes, liposomes, nanoparticles and peptide-conjugates that have been engineered to deliver stably expressed shRNAs and small and large RNAs in vivo. 220–225
There are less technical problems in plants, and shRNA-based transgenes have been widely used to engineer or to exogenously deliver, for instance, viral and fungal resistance in horticultural crops and ornamental plants. 226 , 227
Viral vectors may also be used to deliver normal copies of defective genes, although their payload is limited by the amount of DNA that can be packaged in the virus, and there may be residual immunogenicity and genotoxicity problems. 218 , 228 , 229 Nonetheless, AAV vectors carrying replacement genes or antisense oligonucleotides to rescue or bypass splicing defects have been used successfully to treat different conditions, such as spinal muscular atrophy, 230 , 231 muscular dystrophy 232 and retinal dystrophy. 233
However, the use of siRNA and shRNA in research and gene therapy is being rapidly supplanted by a far more efficient and precise genome manipulation system, based on RNA targeting of engineered nucleases, called CRISPR, discussed later in this chapter.
MicroRNAs
Closely related endogenous RNA regulatory pathways that regulate differentiation and development were waiting in the wings to be discovered. As described in Chapter 9, a 22nt small RNA, lin-4, had been described by Victor Ambros and colleagues in 1993 to control developmental timing in C. elegans. 234 In 2000, a second small (~21-nt) regulatory RNA species, let-7, was identified by Gary Ruvkun and colleagues, produced from a locus that, like lin-4, controls developmental timing by negatively regulating the lin-41 (protein-coding) gene through partial complementarity to target sequences in its 3′UTR. 235 The similarity to the lin-4 system led the authors to conclude that
“the mechanism by which they [lin-4 and let-7] regulate the expression of their target could be related” and that they “may constitute elements of a cascade of stage-specific regulatory RNAs that control the temporal sequence of events in C. elegans development”. 235
Shortly thereafter, Amy Pasquinelli, Ruvkun and colleagues found that the let-7 sequence, size, and ‘hairpin’ structure of its precursor are highly conserved in invertebrates and vertebrates, 236 with the latter having multiple paralogs. 237 The timing of let-7 expression during development and its target sites in the lin-41 mRNA are also conserved. 236 , 238 These “small temporal RNAs” 236 became known as ‘microRNAs’ (miRNAs).
The similarity of size of miRNAs and siRNAs (and the small RNAs described in plant PTGS), suggested a relationship between them, 236 confirmed when Alla Grishok and colleagues found that inactivation of Dicer and other components of the RNAi pathway impaired miRNA processing and activity. 239
After the realization of the possible existence of other small RNA “classes”, and despite “lingering skepticism” and wariness “of what we imagined was a vast detritus of partially degraded RNAs in cells”, 240 the development of strategies for large-scale isolation and sequencing of small RNAs with “probable regulatory roles”, 237 , 241 , 242 as well as bioinformatic searches using the available genome sequences, 243 led to the cloning and characterization of large numbers of small ~21nt RNAs from a variety of organisms, including mammals, 237 , 241 , 242 , 244–249 “deviants no longer”. 250
As Ruvkun observed at the time:
why have more of the miRNAs not been revealed by genetic analysis? …the number of genes in the tiny RNA world may turn out to be very large, numbering in the hundreds or even thousands in each genome. Tiny RNA genes may be the biological equivalent of dark matter — all around us but almost escaping detection, until first revealed by C. elegans genetics. 251
Subsequent studies showed that miRNAs are also produced from processed introns and other non-coding transcripts, j including RNAs derived from repetitive sequences, that form an internally folded dsRNA stem-loop structure with an imperfectly paired double-stranded stem. 254 , 259–265 This structure is recognized and cleaved from precursor host RNAs by a ‘Microprocessor’ complex comprised of the RNaseIII endoribonuclease Drosha and its partner dsRNA-binding protein Pasha/DGCR8, 266–270 or generated directly from the debranching of lariat intermediates formed during intron excision (‘mirtrons’) 271–273 to yield a hairpin-shaped RNA of ~65–70 nucleotides in length. This ‘pre-miRNA’ is then cut by Dicer in the cytoplasm, loaded into the RISC complex, which (after ejection of the ‘passenger’ strand) targets cognate mRNAs for deadenylation of their polyA tails and degradation and/or translational inhibition by binding to complementary sequences primarily in 3′UTRs (Figure 12.3). 91 , 239 , 274–276

Figure 12.3
Biogenesis and mechanism of action of the main classes of small regulatory RNA. (Reproduced from Jinek and Doudna with permission of Springer Nature.)
Thousands of tissue-specific miRNAs 244 , 277–280 – some of which are conserved over long evolutionary distances, 281 whereas others are more lineage-restricted 247–249 , 282 – have been identified and shown to regulate a wide range of differentiation and cellular processes. 274 , 277 , 283 , 284 These include maintenance of pluripotency and stem cell states, 277 , 285 cell proliferation, 286 epithelial to mesenchymal transition, 287 brain morphogenesis, 288 hematopoietic differentiation, 289 neuronal asymmetry, 290 dendritic spine development, 291 muscle development, 292 programmed cell death, 293 innate immune responses, 294 metabolism, 295 leaf development 296 , 297 and flowering time, 298 among many others. k MicroRNAs also undergo developmentally regulated post-transcriptional modifications, 299 , 300 and exhibit altered expression in developmental disorders and cancers 301–303 (dubbed ‘oncoMiRs’ 304 ).
A feature of miRNAs, which generally distinguishes them from siRNAs, is that the precursor dsRNA structure that is recognized by Drosha does not have perfect base complementarity, and either strand may be utilized. Importantly, both miRNAs and siRNAs can repress gene expression without activating the interferon pathway, 89 which is triggered by longer dsRNAs. 305 MicroRNAs appear to have evolved before multicellularity, as they are also found in unicellular algae, 306 although their repertoire expanded greatly during metazoan, vertebrate and mammalian evolution. 307 , 308
Curiously, it appears that an individual miRNA may recognize sites in many mRNAs, 309–311 and that many, if not most, mRNAs, also contain bona fide recognition sites for multiple miRNAs. 312 , 313 The regulatory logic for this reciprocal multiplexing is unclear and, while miRNAs appear to locate their targets through an 8-nucleotide ‘seed’ sequence, 297 , 310 the rules for target recognition are still being teased out, 314–316 as also is the place of miRNAs in decisional hierarchies (Chapter 15). 317
Although miRNAs are thought to operate primarily on mRNAs in the cytoplasm, they also operate in the nucleus to target pre-mRNAs, 318 as well as enhancer RNAs 319 (to modulate chromatin architecture; Chapters 14 and 16) and nuclear RNAs such as 7SK. 320 They also bind to long non-coding RNAs, sometimes thought to function as miRNA ‘sponges’ or ‘decoys’, 321–323 although they are likely legitimate and common targets in their own right. Some miRNAs also trigger the production of 21–22nt siRNAs from transposons, 324 referred to as ‘easiRNAs’ (epigenetically activated small interfering RNAs), to control genome dosage response and hybridization barriers in plants. 325 MicroRNAs also influence nuclear DNA methylation, 326 , 327 but the intersecting small RNA pathways and networks have yet to be understood.
Nonetheless, a sort of consensus has emerged. In general, siRNAs are perfectly complementary to their targets and promote their degradation, and in plants are used for defense against viruses and transposons, as well as adaptive responses to environmental stress. 328 siRNAs are also produced in animals - in oocytes, embryonic and somatic cells, from pseudogenes l and TEs and other naturally formed dsRNAs 331 , 332 - where they regulate transposons, genome dosage response and developmental processes, likely interrelated. 94 , 195 , 198 , 265 , 329 , 330 , 333–337 On the other hand, miRNAs are imperfectly complementary to mRNA (usually) 3′UTR sequences and often direct translation inhibition, as well as less well characterized targets, for the regulation of developmental processes as described above.
Piwi-Associated RNAs
In 2006, a related general class of small RNAs was described, 338–342 although they had been detected a few years earlier by Alexei Aravin and colleagues in analyses of tandem repeat and retrotransposon silencing 333 and small RNA sequencing datasets in the testis. 343 A subgroup of Argonaute proteins, known as the ‘Piwi family’, m was known to be required for germ- and stem-cell development in animals, three homologs (Mili, Miwi and Miwi2) being essential for spermatogenesis in mammals 345 , 346 (Figure 12.4). These proteins bind ‘Piwi-interacting RNAs’, or piRNAs, which are longer than miRNAs, generally ranging from 25 to 33nt, and have a characteristic 2′O-methylation n of the 3′ terminal ribose, 348 which is recognized by the PAZ domain of Piwi proteins, 349 , 350 with those interacting with different Miwi/Mili proteins having a different mean length. 346 , 351 , 352

Figure 12.4
Biphasic production of piRNAs and Miwi expression during mouse sperm development, (Adapted from Zheng and Wang, under Creative Commons Attribution License.)
Thousands of genomic loci have been found to produce piRNAs. o These RNAs are present in millions of copies in testes and are so abundant that, like the discovery of small nuclear RNAs in the 1960s, they were discovered by visualization in gel electrophoretic displays. PiRNAs are also expressed in ovaries 353–358 and somatic cells, 354 , 359 where they regulate gene expression in stem cells and embryonic development, 357 , 358 and in the brain, where they are required for neurogenesis and memory formation 360–363 (Chapter 17). piRNA expression is also dysregulated in cancer. 364 They are not produced by Drosha processing of dsRNA intermediates but the best understood mechanism for piRNAs derived from repeat sequences and present in diverse organisms is through a ‘ping-pong’ amplification system from single-stranded RNA templates. 98–100
piRNAs are thought to be (mainly) involved in the repression of transposons, p likely their ancestral function. 196 , 346 , 352 , 367–371 They are also involved in parental imprinting. 372 However, their range is much greater. For instance, male-specific chromosome Y-derived long non-coding transcripts, named Pirmy and Pirmy-like RNAs, which exhibit a large number of splice variants in testis, act as templates for piRNAs that regulate autosomal genes. 373 piRNAs are also derived from other sequences, including intergenic transcripts, pseudogenes and the 3′UTRs of mRNAs, 112 , 374 , 375 the latter targeting the introns of other genes. 376 Along with their roles in development and brain function, these observations lead to the conclusion that genomes have co‐opted the TE‐derived piRNA system for regulating transposon activity and other dimensions of genome regulation. 375–379 These include the modulation of nuclear architecture at target loci containing TEs, shown in a Drosophila ovarian somatic cell line and involving directing these genomic regions to the nuclear periphery, heterochromatin formation and chromatin conformational changes to promote transcriptional silencing; thus providing a possible mechanism for the proposed role of TEs as “functional motifs” for the dynamic regulation of chromatin state and nuclear architecture 380 , 381 (see Chapters 14 and 16).
In sperm development in Drosophila and mammals, there are two bursts and classes of piRNAs and two corresponding rounds of Piwi protein expression. The first class is expressed before the meiotic ‘pachytene’ stage of spermatogenesis, is derived from transposon‐rich clusters, and associates with Mili and Miwi2. The second class is expressed during the pachytene stage from thousands of genomic loci, is extremely abundant and associates with Mili and Miwi1. 382–384 Their function is unknown, 385 although an intriguing possibility is that this dual system evolved to enhance evolvability (Chapter 18), with quality control, when progeny numbers are small. 386 There is widespread formation of dsRNAs in the testis, different in profile from that observed in somatic cells. 387 Moreover, the loss of fertility in Piwi mutants lacking piRNAs is not due to de-repression of repetitive elements: pachytene piRNAs repress gene expression by directing the cleavage of meiotic transcripts required for sperm function. 388 , 389
It may also be that the phenotypes of the loss of piRNA function are mediated by epigenetic silencing of histone genes, 390 implicating small RNAs in the transmission of epigenetic information across generations (Chapter 17). With many more piRNA loci yet to be studied, there is much to be learned about this large class of small RNAs in animals.
Other Classes of Small RNAs
The landscape is even more complicated, as most RNAs form double-stranded structures that can be and frequently are processed into small RNAs.
A variety of small RNA species are produced from rRNAs (‘rRFs’) in a developmental stage-specific manner in fungi, plants and animals. 391–393 Most derive from 28S rRNA, and have various sizes, some shown to be siRNAs (‘phasiRNAs’), miRNAs and piRNAs, as well as ‘qiRNAs’ 394 that function in DNA damage response. q The 18S rRNA is also the source of smaller RNAs, including miRNAs, as well as a longer species of 130nt that may be an miRNA precursor. miRNAs are also processed from the 5.8S rRNA and the 45S rRNA precursors. 391 The piRNA pathway is required to control the abundance of rRNA-derived siRNAs and the ribosomal RNA pool in C. elegans. 398
In all branches of life, tRNAs are processed into highly abundant smaller species (‘tRFs’ for tRNA-derived fragments, or ‘tsRNAs’ for tRNA-derived small RNAs), 399–403 initially thought to be degradation products, 404 despite their production being cell-state and tissue-specific 399 , 405 , 406 and (at least in some cases) Dicer-dependent, 400 , 407 possibly playing a role in the global regulation of RNA silencing. 408 Some tRNA fragments function as miRNAs. 409–411 The production of tRFs is influenced by methylation of the tRNA by the RNA methyltransferases Dnmt2 412 and Nsun2, which may be involved in transgenerational inheritance (see below) and loss of which causes neurodevelopmental disorders. 413 tRNAs and tRNA fragments are also modified and regulated by TET2, which oxidizes 5-methylcytosine in DNA 414 (Chapter 14). tRFs and modifications thereof are stress-induced and inhibit translation, 415–419 control retrotransposons, 420 , 421 promote cell proliferation, 401 and are depleted in immortalized cell lines. 406
tRFs also convey long distance regulatory signals. Their levels are increased in Arabidopsis roots upon phosphate starvation, 422 and rhizobial (symbiotic bacterial) tRFs modulate host nodulation in legumes via the RNAi pathway. 423 They are present, along with miRNAs, in secreted exosomes 144 from stem cells 424 and activated T-cells. 425 They are also abundant in semen and sperm, 420 , 426 , 427 and have been found to contribute to intergenerational inheritance of acquired metabolic disorders, with evidence that this involves their methylation 428–430 (see Chapter 17). tRFs have also been shown to affect the biogenesis of snoRNAs, scaRNAs and snRNAs, as well as histone levels and chromatin organization 431 (Chapter 14).
tRNA-like small RNAs are also produced from the processing of long non-protein-coding RNAs, such as MALAT1, 432 , 433 one of the most highly expressed genes in the vertebrate genome, which also produces piRNAs. 434
All known snoRNAs (in fungi, plants and animals, from yeast to human) are processed to produce smaller species: those derived from C/D snoRNAs show a bimodal size distribution of 17–19nt and >27nt, the latter similar in size to piRNAs. 436 Those derived from H/ACA snoRNAs are predominantly 20–24nt in length 436 (Figure 12.5), at least some of which have been shown to be Dicer-dependent, bind to Argonaute, function as miRNAs and have developmental effects. 265 , 435–439

Figure 12.5
Processing of an miRNA from snoRNA ACA45. The blue bar shows the sequences of the putative miRNA products and the number of reads in Argonaute-associated small RNA sequencing data, while the red bar shows putative ‘star' product from the stem-loop (more...)
Likewise, spliceosomal snRNAs are processed into smaller fragments, 409 although their significance is unknown. Vault RNAs (Chapter 8) are processed as well, dependent on cytosine methylation, into small RNAs that bind to Argonaute proteins and exhibit miRNA-like functions. 440 Other short (80–100nt) intronically derived RNAs, some of which are highly conserved, also associate with Argonaute proteins and are capable of repressing mRNAs via target sequences in the 3′UTRs. 441 All of these observations indicate deep evolutionary and complex functional connections between regulatory RNA pathways.
Finally, another class of small RNAs with a modal length of 17–18nt is associated with transcription start sites (‘tiRNAs’) and exonic splice sites (‘spliRNAs’) in animals, but not in plants, with evidence that they are associated with binding sites for epigenetic regulators and mark nucleosome positions, 442–444 which may be subject to fine control in animals because of the required precision of ontogeny (Chapter 15).
RNA Communication between Species
Some of the early experimental RNAi methods in C. elegans indicated the potential of interspecies communication by small RNAs: “dsRNAs expressed in Escherichia coli could induce gene silencing in nematode larvae that feed on them … raising the possibility that dsRNAs may be transferred from prokaryotic pathogens to their hosts”. 445
Small RNAs have subsequently been shown to mediate communication between species, for pathogenicity, defense or symbiosis. For example, the small RNA SsrA produced by the bacterium Vibrio fischeri is loaded into outer membrane vesicles that are transported into the epithelial cells in the light organ of the squid and suppress antimicrobial responses and permit light-organ symbiosis between the bacterium and its host. 446
The soil bacterium Pseudomonas aeruginosa, which is pathogenic in many species, releases a small RNA termed P11 into C. elegans that is processed by the RNAi and piRNA machinery, and specifically silences the expression of the protein maco-1, which functions in chemotaxis and neuronal excitability, leading to avoidance behavior that is heritable for four generations, of value to both. 447 C. elegans transmits this memory of learned avoidance to naive animals and four generations of progeny through virus-like particles encoded by the Cer1 retrotransposon. 448
Similarly, the bacterial pathogen Listeria monocytogenes secretes a small RNA-binding protein named Zea that binds a subset of L. monocytogenes RNAs and RIG-I, the non-self-RNA innate immunity sensor in mammalian cells. In mice, this was shown to dampen the immune response and suggested to allow sustainable host-bacterium symbiosis. 449 Zea orthologs occur in other bacteria that are rarely associated with disease, suggesting that this may be a general mechanism to assist co-existence between bacteria and multicellular hosts, 449 especially in view of the growing awareness of the importance of the enteric microbiome in ruminant biology and mammalian physiology.
These may occur between eukaryotic kingdoms, as shown between the mycorrhizal fungus Pisolithus microcarpus and its host Eucalyptus grandis, in which the fungus miRNA Pmic_miR-8 is transported into the plant roots, targeting host genes to promote symbiosis. 450 Such interactions may influence pathogenicity and aggressiveness of parasites, as exemplified by the interaction between the fungal pathogen Botrytis cinerea and host plants, proposed to involve retrotransposon-derived small RNAs derived from the fungus and delivered into plant cells and cross-kingdom RNA interference (ckRNAi) to undermine host defense. 451
Even complex ecological interactions may be affected by regulatory RNAs. This is suggested by the reported effect of short satellite RNAs (satRNAs, a type of subviral agent associated with some viruses, Chapter 8) that accompanies cucumber mosaic virus (CMV) and are processed into small RNA (sRNA) species in infected tobacco leaves. The production of these Y-sat sRNAs affects host gene expression and leads to a change of the color of the leaves from green to bright yellow, which preferentially attracts aphid vectors. Surprisingly, the Y-sat RNAs are also processed in the aphids feeding on the leaves, leading to the production of small RNAs that affect aphid physiology, turning them red and subsequently promoting wing formation. This in turn facilitates CMV and Y-sat RNA spread without apparent detrimental effects on the plant, thus suggesting a potential intricate ‘survival strategy’ by a subviral non-coding RNA, which depends on interaction with (and affects the interactions between) the CMV helper virus, the host plant and the insect vector. 452
It is early days in the exploration and understanding of the extent of communication by “social RNA” (a term coined by Eric Miska) 453 , 454 in the competition and cooperation within and between species in complex environments, but there are further documented examples of RNA exchange, including between parasitic nematodes and mammalian cells, 455 plants and fungal pathogens, 456 , 457 parasitic and host plants, 458 and even between queen and worker bees via royal and worker jellies, 459 , 460 a pathway which has been exploited to artificially introduce virus resistance to commercial hives. 461
CRISPR
The exponential growth and analysis of DNA and RNA sequence data transformed molecular biology, no better demonstration being the serendipitous discovery r of the CRISPR RNA-based immune system in prokaryotes. 466–470
In 1987, Yoshizumi Ishino and colleagues reported the existence of mysterious, partly palindromic ~30nt tandemly repeated sequences, separated by ~30 nt ‘spacers’ in E. coli. 471 A similar peculiar array of interspersed repeats was described in the salt-tolerant archaeon Haloferax mediterranei by Francisco Mojica and colleagues in 1993, who went on to collate instances of such sequences in many different species, which they called ‘Short Regularly Spaced Repeats’. 472 , 473 Others doing related bioinformatic analyses showed that a common set of protein-coding ‘cas’ s genes lay adjacent to these repeats, which they termed ‘Clustered Regularly Interspaced Short Palindromic Repeats’, 474 whose acronym, CRISPR, being more euphonic, became adopted.
The function of CRISPR was revealed in 2005, when three groups, including Mojica’s, t discovered that the spacers separating the repeats corresponded to fragments of bacteriophages and plasmids, indicating that these sequences recorded past invasions and constituted a memory-defense system, 477–479 reinforced by the bioinformatically inferred nuclease function of the CRISPR-associated cas genes and analogies with RNAi. 479 , 480
This conclusion was confirmed when Philippe Horvath, Rodolphe Barrangou, Sylvain Moineau and colleagues working at the French company Danisco u showed in 2007 that recently selected phage-resistant mutants of the lactic acid-producing bacterium Streptococcus thermophilus, used in yogurt and cheese production, had acquired phage-derived sequences at their CRISPR loci and that phage isolates that overcame the immunity had single nucleotide changes that altered the sequences corresponding to the spacers. 481 These and other investigators, notably Luciano Marraffini, John van der Oost and colleagues, also showed that the CRISPR array is transcribed and cleaved within the repeats to produce 61 nt ‘crRNAs’ wherein the spacer (target) sequence is flanked by the palindromic sequences of the repeats. The crRNAs are then loaded into a cas-encoded nuclease, 481–484 which uses the spacer guide sequence to target and introduce a double strand break in cognate DNA via two distinct nuclease domains. v The Cas proteins are essentially RNA-programmable restriction enzymes 487 , 488 (Chapter 6), some of which are also used for transposon homing. 489 , 490
A vital missing link was discovered when Emmanuelle Charpentier and colleagues, who had earlier shown that streptococcal virulence factors are controlled by regulatory RNAs, 491 found that the third most highly expressed sequence (after rRNA and tRNA) in Streptococcus pyogenes is a small RNA that is transcribed from a sequence adjacent to the CRISPR locus and has 25 bases of near-perfect complementary to the repeats. This RNA, termed ‘tracrRNA’ (trans-activating CRISPR RNA), hybridizes to the transcribed CRISPR array to guide cleavage by host-encoded RNaseIII, 492 is loaded into the cas nuclease along with a crRNA, and is essential for its function 485 (Figure 12.6). An endogenous guide RNA autoregulates CRISPR-Cas expression to mitigate autoimmune toxicity. 493

Figure 12.6
The type II CRISPR-Cas9 System from Streptococcus thermophilus. (Reproduced from Lander with permission of Elsevier.) Like RNAi, CRISPR-Cas systems rely on RNA guidance for target specificity. (a) The locus contains a CRISPR array, four protein-coding (more...)
There are a number of variants of CRISPR systems in bacterial and archaeal genomes. 496 , 497 Type I and type III systems employ a large multi-Cas protein complex for crRNA binding and target cleavage. 466 , 467 The simplest, Type II, consists of the CRISPR array, the gene encoding the tracrRNA, and four protein-coding cas genes, three of which are involved in generating new spacers and repeats and the other, cas9, encodes the targeting nuclease. 486
Virginijus Siksnys, Horvath, Barrangou and colleagues showed that the CRISPR system from S. thermophilus could be functionally transferred into E. coli and that all that was required to target and cleave a specific DNA sequence is the tracrRNA, the Cas9 nuclease and the corresponding crRNA, 498 which could be as short as 20 nt as the flanking repeat sequences are not required, paving “the way for engineering of universal programmable RNA-guided DNA endonucleases”. 475
Shortly thereafter, Charpentier, Martin Jinek, Jennifer Doudna and colleagues showed that the normally base-paired cRNA and tracrRNAs could be covalently linked and produced as a single chimeric guide RNA (sgRNA), simplifying delivery of the system. 485 , 486
CRISPR systems have also been coopted naturally for various structural and regulatory functions by repurposing of diverged repeats encoded outside of CRISPR arrays, including by transposons for RNA-guided transposition, by plasmids for interplasmid competition, and by viruses for antidefense and interviral conflicts. There are also multiple highly derived CRISPR variants of yet unknown functions. 499
RNA-Directed Genome Editing
In 2013, the groups of Feng Zhang 500 and George Church 501 demonstrated that the CRISPR system (Cas9 plus sgRNAs) could be used in human and mouse cells to introduce sequence-specific double strand breaks, w which, when repaired in vivo, lead to incorporation of guided mutations, the latest in a long line of ‘homing endonucleases’ with wide practical applications. 504 , 505 Zhang and colleagues showed that they could engineer the Cas9 sequence to disable one of its two nuclease domains, converting it into a ‘nickase’ enzyme that only introduces a single-strand break, facilitating the insertion of new sequences into genomes by homology-directed repair. They also showed that multiple guide sequences can be encoded into a single CRISPR array to enable the simultaneous alteration of several sites. 500
CRISPR technology has proven far more versatile and efficient than the previous methods developed for targeted genome modification, which employed nucleases linked to the DNA-binding domains of transcription factors whose sequence specificity could be engineered by altering amino acids in these domains (called ZFNs x and TALENs), an effective but relatively cumbersome methodology. 506–508
Moreover, CRISPR technology has been adapted by engineering the Cas9 and other Cas nucleases to inactivate, manipulate, image and annotate specific DNA and RNA sequences in a wide variety of organisms, 495 , 509–514 including high-throughput mutagenesis to identify the roles of new genes and genetic pathways in cell and developmental biology. 515 CRISPR has also been widely used in industrial biotechnology 516 , 517 and in agriculture to construct transgenic plants and animals with desirable properties. 518 , 519
The toolkit and applications have been advancing at breathtaking pace. 520 For example, nuclease-inactive and RNA-targeting Cas proteins have been fused to a plethora of different effector proteins to alter gene expression, epigenetic modifications and chromatin interactions. 495 , 521–526 These include the fusion of transcriptional repressors or activators to catalytically inactive Cas9 to repress (‘CRISPRi’) or enhance (‘CRISPRa’) expression of specific protein-coding and non-coding genes (Figure 12.7). 527–531

Figure 12.7
CRISPR applications. Top panel, genome editing; bottom panel, RNA-targeting, tagging and editing. (Reproduced from Pickar-Oliver and Gersbach with permission of Springer Nature.)
They also include the fusion of cytidine deaminase or reverse transcriptase to Cas9 by to enable single base replacement or the insertion of any desired sequence (programmed into the guide RNA) at any specific position in the genome, termed ‘base editing’ and ‘prime editing’, respectively. These have been demonstrated to reverse damaging mutations at high efficiency in cell culture 494 , 532–535 and (to a limited extent, but with increasing success) in vivo including amelioration of deafness and amyloidosis, 536–538 which “in principle could correct up to 89% of known genetic variants associated with human diseases”. 534 CRISPR systems have also been adapted to edit RNA with high specificity 539 to correct a range of genetic defects, 540–543 and to develop multiplexed assays for high sensitivity detection of viral RNAs. 544–546
CRISPR is also being deployed to propagate the ingression of desirable genes into natural populations, first mooted by CF Curtis in 1968 (using translocations) 547 , 548 and again by Austin Burt in 2003 (using “site-specific selfish genes” such as homing endonucleases, group II introns and some types of transposable elements). 505 To date, effective introduction of ‘gene-drive’ systems based on CRISPR-Cas9 has been achieved in yeast, 549 , 550 fruitfly 551 and mosquito, 552–554 among others, with applications including eliminating vector-borne and parasitic diseases, promoting sustainable agriculture and curtailing invasive species, albeit with biosafety and ‘ethical’ concerns, 555 , 556 which in turn have motivated the design of tunable and reversible systems. 557–560
Advanced nanoparticle delivery systems are being developed in parallel to enable high-efficiency tissue-specific delivery in vivo for gene therapy, vaccination, cancer treatment and investigative genetic manipulation. 561–563 The efficiency and specificity of genome editing continues to improve, with constant factor being the flexibility of RNA guides to unlock the potential of targeting generic effector proteins to specific DNA or RNA locations.
A billion years ago, RNA guides also solved the challenges of regulating cell fate decisions and tissue architecture in multicellular organisms (Chapters 15 and 16).
Further Reading
- Abbott A. (2016) The quiet revolutionary: How the co-discovery of CRISPR explosively changed Emmanuelle Charpentier’s life. Nature 532: 432–434. [PubMed: 27121823]
- Castel S.E. and Martienssen R.A. (2013) RNA interference in the nucleus: Roles for small RNAs in transcription, epigenetics and beyond. Nature Reviews Genetics 14: 100–112. [PMC free article: PMC4205957] [PubMed: 23329111]
- Gutbrod M.J. and Martienssen R.A. (2020) Conserved chromosomal functions of RNA interference. Nature Reviews Genetics 21: 311–331. [PMC free article: PMC9478574] [PubMed: 32051563]
- Lander E.S. (2016) The heroes of CRISPR. Cell 164: 18–28. [PubMed: 26771483]
- Ledford H. (2016) The unsung heroes of CRISPR. Nature 535: 342–344. [PubMed: 27443723]
- Maraganore J. (2022) Reflections on Alnylam. Nature Biotechnology 40: 641–650. [PubMed: 35534556]
- Özata D.M., Gainetdinov I., Zoch A., O’Carroll D. and Zamore P.D. (2019) PIWI-interacting RNAs: Small RNAs with big functions. Nature Reviews Genetics 20: 89–108. [PubMed: 30446728]
- Pasquinelli A.E. (2015) MicroRNAs: Heralds of the noncoding RNA revolution. RNA 21: 709–710. [PMC free article: PMC4371344] [PubMed: 25780202]
- Veigl S.J. (2021) Small RNA research and the scientific repertoire: A tale about biochemistry and genetics, crops and worms, development and disease. History and Philosophy of the Life Sciences 43: 30. [PubMed: 33624250]
Footnotes
- a
Interferons were first described and named in 1957 by Alick Isaacs and Jean Lindenmann, who showed inhibition of influenza virus growth by prior exposure of cells to active or heat-inactivated virus. 4 They are now known to comprise three classes of proteins, two of which (interferon types I and III) bind to cell surface receptors and induce expression of proteins that prevent the virus from replicating. 5 , 6 Interferon gamma (type II) enhances specialized subsets of immune responses and is involved in the development of autoimmune disorders such as multiple sclerosis. 7
- b
Animal cells also discriminate foreign nucleic acids by their lack of nucleotide modifications, in DNA by lack of CpG methylation and in RNA by lack of base modifications, sensed by the so-called Toll-like receptors, 23–26 which also play a role in development and brain synaptic architecture. 27–30 The modification of synthetic mRNAs to circumvent the innate immune response was a critical step in the development of mRNA vaccines. 31
- c
- d
Some animals, including mammals, have lost RdRPs but have instead evolved a ‘ping-pong’ amplification system to amplify piRNAs (see below). 98–100
- e
Mammalian stem cells protect themselves from some RNA viruses (including Zika virus and SARS-CoV-2) by expressing an alternatively spliced isoform of Dicer, which potentiates antiviral RNAi, a process similar to that in insects and worms, which also use Dicer-dependent RNA interference in antiviral defense, in contrast to mammalian differentiated cells, which generally rely on the interferon system. 106
- f
- g
It is not yet known how the Argonaute proteins distinguish invading DNA from host sequences, 120 although one possibility is that it involves base modification.
- h
- i
- j
Examples, among many others, include the non-coding RNA BIC (B-cell integration cluster) originally identified as a common site for proviral insertion in lymphomas 252–255 and classic lncRNAs such as H19, 256 , 257 similar to small nucleolar RNA host genes. At least some (like H19) have a dual function as regulatory lncRNAs, such as the miR-205 host transcript that regulates pituitary hormone production. 258
- k
Despite their ubiquity, miRNAs have rarely shown up in genetic screens (see Chapter 13). Indeed, prior to their biochemical discovery, only one miRNA locus had originally been picked up in Drosophila – a gene called bantam – and was not initially recognized as a microRNA. 286
- l
Processed from duplexes between antisense pseudogene (non-coding) RNAs and corresponding mRNAs. 329–331
- m
The name given to the original Drosophila mutant: P-element-induced wimpy testis (Piwi). 344
- n
All miRNAs and siRNAs in plants and siRNAs in Drosophila are 2′-O-methylated at their 3′ ends, apparently to improve their stability. 347
- o
There are over 2,500 miRNA and over 23,000 piRNA candidate loci documented in humans, rivaling the number of protein-coding genes. 351
- p
Involving the long-described but mysterious, germ-cell-specific ‘nuage’ organelle, 365 which forms a phase-separated domain (Chapter 16) that concentrates single-stranded DNA but largely excludes double-stranded DNA. 366
- q
Similar 20–35nt small ‘ddRNAs’ have been reported elsewhere to control the DNA damage response via interaction with damage-induced long non-coding RNAs (Chapter 13) and the formation of phase-separated domains (Chapter 16) at DNA double strand breaks. 395–397
- r
Another serendipitous discovery was bacterial ‘retrons’ which were first discovered in 1989 in myxobacteria and E. coli. 462 , 463 Retrons are chimeric covalently linked RNA/DNA molecules produced by reverse transcriptase, prior to which reverse transcriptases had not been known to exist in bacteria. 464 Later studies showed that retrons form a second line of defense against phages (abortive infection by cell death) that is triggered if the first lines of defense have collapsed. 465
- s
‘CRISPR-associated’.
- t
Mojica’s paper was first submitted for publication in 2003, but not published until early 2005, due to multiple rejections. In fact all three papers were rebuffed by leading journals, being considered by their editors to lack sufficient novelty and importance to send out for peer review. 469 A similar story of editorial rejection and reviewing delays by leading journals robbed Giedrius Gasiunas and colleagues of recognition for the co-discovery of RNA programmable Cas9–crRNA target cleavage. 475 , 476
- u
As in many other cases, like antisense RNAs and RNAi (to generate virus-resistant transgenic plants), some practical applications of CRISPR preceded mechanistic understanding. The company (Danisco), which was acquired by DuPont, announced in 2012 the commercial availability of several S. thermophilus strains immune to various bacteriophages for pizza cheese-making.
- v
- w
Group II introns were the first RNAs to be used for gene/genome targeting. 502–504
- x
Involving ‘zinc fingers’, see Chapter 16.
- Unusual Genetic Phenomena Involving RNA
- The RNA Interference Pathway
- Transcriptional Gene Silencing: RNA-Directed DNA Methylation
- Research and Biotechnology Applications
- MicroRNAs
- Piwi-Associated RNAs
- Other Classes of Small RNAs
- RNA Communication between Species
- CRISPR
- RNA-Directed Genome Editing
- Further Reading
- Small RNAs with Mighty Functions - RNA, the Epicenter of Genetic InformationSmall RNAs with Mighty Functions - RNA, the Epicenter of Genetic Information
- Glimpses of a Modern RNA World - RNA, the Epicenter of Genetic InformationGlimpses of a Modern RNA World - RNA, the Epicenter of Genetic Information
- Overview - RNA, the Epicenter of Genetic InformationOverview - RNA, the Epicenter of Genetic Information
- Asclepias eustegioides (0)OMIM
Your browsing activity is empty.
Activity recording is turned off.
See more...