By agreement with the publisher, this book is accessible by the search feature, but cannot be browsed.
NCBI Bookshelf. A service of the National Library of Medicine, National Institutes of Health.
Alberts B, Johnson A, Lewis J, et al. Molecular Biology of the Cell. 4th edition. New York: Garland Science; 2002.
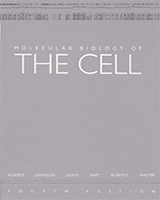
Molecular Biology of the Cell. 4th edition.
Show detailsTransport processes mediate a continual exchange of components between the ten or more chemically distinct, membrane-enclosed compartments that collectively comprise the biosynthetic-secretory and endocytic pathways. In the presence of this massive exchange, how can each compartment maintain its specialized character? To answer this question, we must first consider what defines the character of a compartment. Above all, it is the composition of the enclosing membrane: molecular markers displayed on the cytosolic surface of this membrane serve as guidance cues for incoming traffic and ensure that transport vesicles fuse only with the correct compartment, thereby dictating the pattern of traffic between one compartment and another. Many membrane markers, however, are found on more than one organelle, and thus it is the specific combination of marker molecules that gives each organelle its unique molecular address.
How are these membrane markers kept at high concentration on one compartment and at low concentration on another? To answer this question, we need to consider how patches of membrane, enriched or depleted in specific components, bud off from one compartment and transfer to another. In this section we describe how this is achieved. Some of the basic genetic and biochemical strategies that have been used to study the molecular machinery involved in vesicular transport are outlined in Panel 13-1.
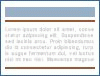
Panel 13-1
Strategies Used to Study the Molecular Mechanisms Involved in Vesicular Transport.
We begin by discussing the sorting events that underlie the segregation of proteins into separate membrane domains. This sorting process depends on the assembly of a special protein coat on the cytosolic face of the donor membrane. We shall therefore consider how coats form, what they are made of, and how they enable specific components of a membrane to be extracted and delivered to another membrane. Finally, we discuss how transport vesicles dock at the appropriate target membrane and fuse with it to deliver the contents to their target organelle.
There Are Various Types of Coated Vesicles
Most transport vesicles form from specialized, coated regions of membranes. They bud off as coated vesicles that have a distinctive cage of proteins covering their cytosolic surface. Before the vesicle fuses with a target membrane, the coat is discarded, as is required to allow the two cytosolic membrane surfaces to interact directly and fuse.
The coat is thought to perform two principal functions. First, it concentrates specific membrane proteins in a specialized membrane patch that then gives rise to the vesicle membrane. It thus helps select the appropriate molecules for transport. Second, the assembly of the coat proteins into curved, basketlike lattices deforms the membrane patch and thereby molds the forming vesicles, which explains why vesicles with the same type of coat have a relatively uniform size.
There are three well-characterized types of coated vesicles, which differ in their coat proteins: clathrin-coated, COPI-coated, and COPII-coated vesicles (Figure 13-4). Each type is used for different transport steps in the cell. Clathrin-coated vesicles, for example, mediate transport from the Golgi apparatus and from the plasma membrane, whereas COPI- and COPII-coated vesicles most commonly mediate transport from the ER and the Golgi cisternae (Figure 13-5). There is, however, much more variety than this short list suggests. As we discuss below, there are at least three types of clathrin-coated vesicles, each specialized for a different transport step, and the COPI-coated vesicles may be similarly diverse. Moreover, still other coats have been seen in the electron microscope, whose molecular compositions and functions are not yet known.

Figure 13-4
Electron micrograph of clathrin-coated, COPI-coated, and COPII-coated vesicles. All are shown in electron micrographs at the same scale. (A) Clathrin-coated vesicles. (B) Golgi cisternae from a cell-free system in which COPI-coated vesicles bud in the (more...)

Figure 13-5
Utilization of different coats in vesicular traffic. Different coat proteins select different cargo and shape the transport vesicles that mediate the various steps in the biosynthetic- secretory and endocytic pathways. When the same coats function in (more...)
The Assembly of a Clathrin Coat Drives Vesicle Formation
Clathrin-coated vesicles were the first coated vesicles discovered and have been the most thoroughly studied. They provide a good example of how vesicles form.
The major protein component of clathrin-coated vesicles is clathrin itself. Each clathrin subunit consists of three large and three small polypeptide chains that together form a three-legged structure called a triskelion. Clathrin triskelions assemble into a basketlike convex framework of hexagons and pentagons to form coated pits on the cytosolic surface of membranes (Figure 13-6). Under appropriate conditions, isolated triskelions spontaneously self-assemble into typical polyhedral cages in a test tube, even in the absence of the membrane vesicles that these baskets normally enclose (Figure 13-7). Thus, the geometry of the clathrin cage is determined by the clathrin triskelion alone.

Figure 13-6
Clathrin-coated pits and vesicles. This rapid-freeze, deep-etch electron micrograph shows numerous clathrin-coated pits and vesicles on the inner surface of the plasma membrane of cultured fibroblasts. The cells were rapidly frozen in liquid helium, fractured, (more...)
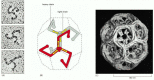
Figure 13-7
The structure of a clathrin coat. (A) Electron micrographs of clathrin triskelions shadowed with platinum. Although this feature cannot be seen in these micrographs, each triskelion is composed of 3 clathrin heavy chains and 3 clathrin light chains. (B) (more...)
A second major coat protein in clathrin-coated vesicles is a multisubunit complex called adaptin. It is required both to bind the clathrin coat to the membrane and to trap various transmembrane proteins, including transmembrane receptors that capture soluble cargo molecules inside the vesicle—so-called cargo receptors. In this way, a selected set of membrane proteins and the soluble proteins that interact with them are packaged into each newly formed clathrin-coated transport vesicle (Figure 13-8).

Figure 13-8
The assembly and disassembly of a clathrin coat. The assembly of the coat is thought to introduce curvature into the membrane, which leads in turn to the formation of uniformly sized coated buds. The adaptins bind both clathrin triskelions and membrane-bound (more...)
There are at least four types of adaptins, each specific for a different set of cargo receptors. Clathrin-coated vesicles budding from different membranes use different adaptins and thus package different receptors and cargo molecules. The formation of a clathrin-coated pit is driven by forces generated by the successive assembly of adaptins and the clathrin coat on the cytosolic surface of the membrane. The lateral interactions between adaptins and between clathrin molecules then aid in bud formation.
Both The Pinching-off and Uncoating of Coated Vesicles Are Regulated Processes
As a clathrin-coated bud grows, soluble cytoplasmic proteins, including dynamin, assemble as a ring around the neck of each bud. Dynamin is a GTPase, which regulates the rate with which vesicles pinch off from the membrane. In the pinching-off process, the two noncytosolic leaflets of the membrane are brought into close proximity and fuse, sealing off the forming vesicle (Figure 13-9). To perform this task, dynamin recruits other proteins to the neck of the budding vesicle, which together with dynamin help to bend the membrane, either by directly distorting the bilayer structure locally or by changing the lipid composition, or both. A local change in lipid composition may result from the action of lipid-modifying enzymes that are recruited into the dynamin complex.

Figure 13-9
The role of dynamin in pinching off clathrin-coated vesicles from the membrane. (A) The dynamin binds to a forming bud on the membrane and assembles into a ring around the neck of the bud. The dynamin ring is thought to be a template that recruits other (more...)
Once the vesicle is released from the membrane, the clathrin coat is rapidly lost. A chaperone protein of the hsp70 family functions as an uncoating ATPase, using the energy of ATP hydrolysis to peel off the coat. Another protein called auxillin, which is attached to the vesicle, is believed to activate the ATPase. Because the coated bud persists much longer than the coat on the vesicle, additional control mechanisms must somehow prevent the coat from being removed before it has formed a vesicle (discussed below).
Although there are many similarities in vesicle budding at various locations in the cell, each cell membrane poses its own special challenges. The plasma membrane, for example, is comparatively flat and stiff, owing to its cholesterol-rich lipid composition and underlying cortical cytoskeleton. Thus, clathrin coats have to produce considerable force to introduce curvature, especially at the neck of the bud where dynamin and its associated proteins facilitate the sharp bends required for the pinching-off of the vesicle. In contrast, vesicle budding from many intracellular membranes occurs preferentially at regions where the membranes are already curved, such as the rims of Golgi cisternae or membrane tubules.
COPI-coated vesicles and COPII-coated vesicles transport material early in the secretory pathway: COPII-coated packages bud from the ER, and COPI-coated packages bud from pre-Golgi compartments and Golgi cisternae (see Figure 13-5). The coats of COPI and COPII vesicles consist, in part, of large protein complexes that are composed of seven individual coat-protein subunits for COPI and four for COPII coats. Some COPI coat-protein subunits show sequence similarity to adaptins, suggesting a common evolutionary origin.
Not All Transport Vesicles are Spherical
Transport vesicles occur in various sizes and shapes. When living cells that have been genetically engineered to express fluorescent membrane components are observed under the microscope, endosomes and the trans Golgi network are seen to continually send out long tubules. Coat proteins assemble onto the tubules and help recruit specific cargo. The tubules then either withdraw, or they pinch off with the help of dynamin-like proteins and thus can serve as transport vesicles. Depending on the relative efficiencies of membrane tubulation and severing, differently sized portions of a donor organelle can pinch off.
Tubules have a much higher surface-to-volume ratio than the organelles from which they form. They are therefore relatively enriched in membrane proteins compared with soluble cargo proteins. As we discuss later, this property of tubules is used for sorting proteins in endosomes. Thus, vesicular transport does not necessarily occur only through uniformly sized spherical vesicles, but can involve larger portions of a donor organelle.
Monomeric GTPases Control Coat Assembly
The vesicular transport performed by both clathrin-coated and COP-coated vesicles depends on a variety of GTP-binding proteins that control both the spatial and the temporal aspects of membrane exchange. As discussed in Chapter 3, large families of GTP-binding proteins regulate diverse processes within cells. These proteins act as molecular switches that flip between an active state with GTP bound and an inactive state with GDP bound. Two classes of proteins regulate the flipping: guanine-nucleotide-exchange factors (GEFs) activate the proteins by catalyzing the exchange of GDP for GTP, and GTPase-activating proteins (GAPs) inactivate the proteins by triggering the hydrolysis of the bound GTP to GDP (see Figure 3-72). Although both monomeric GTP-binding proteins (monomeric GTPases) and trimeric GTP-binding proteins (G proteins) have essential roles in vesicular transport, the roles of the monomeric GTPases are better understood, and we focus our discussion on them.
To ensure that membrane traffic to and from an organelle is balanced, coat proteins must assemble only when and where they are needed. Coat-recruitment GTPases, which are members of a family of monomeric GTPases, usually serve this function. They include the ARF proteins, which are responsible for both COPI coat assembly and clathrin coat assembly at Golgi membranes, and the Sar1 protein, which is responsible for COPII coat assembly at the ER membrane. Clathrin coat assembly at the plasma membrane is also thought to involve a GTPase, but its identity is unknown.
Coat-recruitment GTPases are usually found in high concentration in the cytosol in an inactive, GDP-bound state. When a COPII-coated vesicle is to bud from the ER membrane, a specific GEF embedded in the ER membrane binds to cytosolic Sar1, causing the Sar1 to release its GDP and bind GTP in its place (recall that GTP is present in much higher concentration in the cytosol than GDP and therefore will spontaneously bind after GDP is released). In its GTP-bound state, Sar1 exposes a hydrophobic tail, which inserts into the lipid bilayer of the ER membrane. The tightly bound Sar1 now recruits coat protein subunits to the ER membrane to initiate budding (Figure 13-10). Other GEFs and coat-recruitment GTPases operate in a similar way on other membranes.
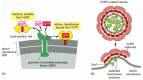
Figure 13-10
A current model of COPII-coated vesicle formation. (A) The Sar1 protein is a coat recruitment GTPase. Inactive, soluble Sar1-GDP binds to a GEF (called Sec12) in the ER membrane, causing the Sar1 to release its GDP and bind GTP. A GTP-triggered conformational (more...)
Some coat protein subunits also interact, albeit more weakly, with the head groups of certain lipid molecules, in particular phosphatidic acid and phosphoinositides, as well as with the cytoplasmic tails of some of the membrane proteins they recruit into the bud. Activated coat-recruitment GTPases at sites of bud formation can locally activate phospholipase D, which converts some phospholipids to phosphatidic acid, thereby enhancing the binding of coat proteins. Together, these protein-protein and protein-lipid interactions tightly bind the coat to the membrane, causing the membrane to deform into a bud, which then pinches off as a coated vesicle.
The coat-recruitment GTPases also have a role in coat disassembly. The hydrolysis of bound GTP to GDP causes the GTPase to change its conformation so that its hydrophobic tail pops out of the membrane, causing the vesicle's coat to disassemble. Although it is not known what triggers the GTP hydrolysis process, it has been proposed that the GTPases work like timers, which hydrolyze GTP at a slow but predictable rate. COPII coats, for example, accelerate GTP hydrolysis by Sar1, thereby triggering coat disassembly at a certain time after coat assembly has begun. Thus, a fully formed vesicle will be produced only when bud formation occurs faster than the timed disassembly process; otherwise, disassembly will be triggered before a vesicle pinches off, and the process will have to start again at a more appropriate time and place. Completion of coating or contact with the target membrane may also trigger coat disassembly.
SNARE Proteins and Targeting GTPases Guide Membrane Transport
To ensure that membrane traffic proceeds in an orderly way, transport vesicles must be highly selective in recognizing the correct target membrane with which to fuse. Because of the diversity of membrane systems, a vesicle is likely to encounter many potential target membranes before it finds the correct one. Specificity in targeting is ensured because all transport vesicles display surface markers that identify them according to their origin and type of cargo, while target membranes display complementary receptors that recognize the appropriate markers. This crucial recognition step is thought to be controlled mainly by two classes of proteins: SNAREs and targeting GTPases called Rabs. SNARE proteins seem to have a central role both in providing specificity and in catalyzing the fusion of vesicles with the target membrane. Rabs seem to work together with other proteins to regulate the initial docking and tethering of the vesicle to the target membrane.
There are at least 20 different SNAREs in an animal cell, each associated with a particular membrane-enclosed organelle involved in the biosynthetic-secretory or endocytic pathway. These transmembrane proteins exist as complementary sets—vesicle membrane SNAREs, called v-SNAREs, and target membrane SNAREs, called t-SNAREs, (Figures 13-11 and 13-12). v-SNARESs and t-SNAREs have characteristic helical domains. When a v-SNARE interacts with a t-SNARE, the helical domains of one wrap around the helical domains of the other to form stable trans-SNARE complexes, which lock the two membranes together. We discuss later how the trans-SNARE complex is thought to contribute to membrane fusion. The specificity with which SNAREs interact determines the specificity of vesicle docking and fusion. In this way SNAREs specify compartment identity and govern the orderly transfer of material during vesicular transport.
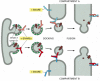
Figure 13-11
The postulated role of SNAREs in guiding vesicular transport. Complementary sets of vesicle SNAREs (v-SNAREs) and target membrane SNAREs (t-SNAREs) contribute to the selectivity of transport-vesicle docking and fusion. The v-SNAREs are packaged together (more...)

Figure 13-12
The structure of paired SNAREs. The SNAREs responsible for docking synaptic vesicles at the plasma membrane of nerve terminals consist of three proteins. The v-SNARE synaptobrevin, and the t-SNARE syntaxin are both transmembrane proteins and each contributes (more...)
SNAREs have been best characterized in nerve cells, where they mediate the docking and fusion of synaptic vesicles at the nerve terminal plasma membrane (see Figure 13-12). The SNARE complexes at neuron terminals are the targets of powerful neurotoxins that are secreted by the bacteria that cause tetanus and botulism. These toxins are highly specific proteases that enter specific neurons, cleave SNARE proteins in the nerve terminals and thereby block synaptic transmissions, often fatally.
Interacting SNAREs Need To Be Pried Apart Before They Can Function Again
Most SNARE proteins in cells have already participated in multiple rounds of membrane targeting and are sometimes present in a membrane as stable complexes with one or two partner SNAREs (see Figure 13-11). The complexes have to be disassembled before the SNAREs can mediate new rounds of transport. A crucial protein called NSF cycles between membranes and the cytosol and cata-lyzes the disassembly process. It is an ATPase that structurally resembles a minor class of cytosolic chaperone proteins that use the energy of ATP hydrolysis to solubilize and help refold denatured proteins. Similarly, NSF uses ATP to unravel the coiled-coil interaction between the helical domains of SNARE proteins, using several adaptor proteins to bind to the SNAREs (Figure 13-13).

Figure 13-13
Dissociation of SNARE pairs by NSF after a membrane fusion cycle is completed. After the v-SNAREs and t-SNAREs have mediated the fusion of a vesicle on a target membrane, the NSF binds to the SNARE complex via adaptor proteins and hydrolyzes ATP to pry (more...)
The requirement for SNARE complex disassembly may help explain why membranes do not fuse indiscriminately in cells. If the t-SNAREs in a target membrane were always active, then any membrane containing an appropriate v-SNARE would fuse whenever the two membranes made contact. The requirement for NSF-mediated reactivation of SNAREs allows the cell to control when and where membranes fuse. In addition, t-SNAREs in target membranes are often associated with inhibitory proteins that must be released before the t-SNARE can function. This release step may be controlled by the targeting GTPases, as we discuss next.
Rab Proteins Help Ensure the Specificity of Vesicle Docking
Rab proteins make an important contribution to the specificity of vesicular transport. They are monomeric GTPases, and with over 30 known members, they are the largest subfamily of these GTPases. Like the SNAREs, each Rab protein has a characteristic distribution on cell membranes and every organelle has at least one Rab protein on its cytosolic surface (Table 13-1). Rab proteins are thought to facilitate and regulate the rate of vesicle docking and the matching of v-SNAREs and t-SNAREs, as required for membrane fusion.
Table 13-1
Subcellular Locations of Some Rab Proteins.
Like the coat-recruitment GTPases discussed earlier (see Figure 13-10), Rab proteins cycle between a membrane and the cytosol. In their GDP-bound state they are inactive and in the cytosol, and in their GTP-bound state they are active and associated with the membrane of an organelle or transport vesicle (Figure 13-14). Many transport vesicles only form if a proper complement of SNARE and Rab proteins are included in the membrane, so as to allow the vesicle to dock and fuse appropriately.
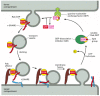
Figure 13-14
A postulated role of Rab proteins in facilitating the docking of transport vesicles. A GEF in the donor membrane recognizes a specific Rab protein and induces it to exchange GDP for GTP. GTP binding alters the conformation of the Rab protein, exposing (more...)
The amino acid sequences of Rab proteins are most dissimilar near their C-terminal tails. Tail-swapping experiments indicate that the tail determines the intracellular location of each family member, presumably by enabling the protein to bind to complementary proteins, including GEFs, on the surface of the appropriate organelle. Once in its GTP-bound state and membrane-bound through a lipid anchor, a Rab protein is thought to bind to other proteins (called Rab effectors) that facilitate the docking process.
In contrast to the highly conserved structure of Rab proteins, the structures of Rab effectors vary greatly from one Rab protein to the next. One Rab effector, for example, is a large protein complex that serves to direct vesicles to specific sites on the plasma membrane for exocytosis. Vesicle fusion is limited to the region where this complex resides, even though the required t-SNAREs are uniformly distributed in the membrane. Some Rab effectors are long, filamentous, tethering proteins, which may restrict the movement of vesicles between adjacent Golgi cisternae. Others bind to Rab proteins in their active GTP-bound state and prevent premature GTP hydrolysis. Yet others are motor proteins that propel vesicles along actin filaments or microtubules to their proper target.
Although the Rab proteins and their effectors use widely different molecular mechanisms to influence vesicular transport, they have a common function. They help concentrate and tether vesicles near their target site and trigger the release of SNARE control proteins. In this way Rab proteins speed up the process by which appropriate SNARE proteins in two membranes find each other. Some Rab proteins function on the vesicle, whereas others function on the target membrane. The pairing of v-SNAREs and t-SNAREs then locks the docked vesicle onto the target membrane, readying it for fusion, which we discuss next. After fusion, the Rab protein hydrolyzes its bound GTP and the inactive GDP-bound protein returns to the cytosol to participate in another cycle of transport.
SNAREs May Mediate Membrane Fusion
Once a transport vesicle has recognized its target membrane and docked there, it unloads its cargo by membrane fusion. Fusion does not always follow immediately, however. As we discuss later, in the process of regulated exocytosis, fusion is delayed until it is triggered by a specific extracellular signal.
Thus, docking and fusion are two distinct and separable processes. Docking requires only that the two membranes come close enough for proteins protruding from the lipid bilayers to interact and adhere. Fusion requires a much closer approach, bringing the lipid bilayers to within 1.5 nm of each other so that they can join. When the membranes are in such close apposition, lipids can flow from one bilayer to the other. For this close approach, water must be displaced from the hydrophilic surface of the membrane—a process that is energetically highly unfavorable. It seems likely that all membrane fusions in cells are catalyzed by specialized fusion proteins that provide a way to overcome this energy barrier. We have already discussed the role of dynamin in a related task during clathrin-coated vesicle budding (see Figure 13-9).
SNAREs are thought to have a central role in membrane fusion. The formation of the SNARE complex may work like a winch, using the energy that is freed when the interacting helices wrap around each other to pull the membrane faces together, while simultaneously squeezing out water molecules from the interface (Figure 13-15). When liposomes containing purified v-SNAREs are mixed with liposomes containing matching t-SNAREs, their membranes fuse, albeit slowly. In the cell, other proteins recruited to the fusion site presumably cooperate with SNAREs to initiate fusion. Moreover, inhibitory proteins may have to be released to allow the complete zipping-up of SNARE pairs. In some cases, such as in regulated exocytosis (discussed later), a localized influx of Ca2+ triggers the fusion process.

Figure 13-15
A model for how SNARE proteins may concentrate in membrane fusion. Bilayer fusion is proposed to occur in multiple steps. A tight SNARE pairing forces lipid bilayers into close apposition so that water molecules are expelled from the interface. Lipids (more...)
Viral Fusion Proteins and SNAREs May Use Similar Strategies
Membrane fusion is important in other processes beside vesicular transport. Examples are the fusion of the plasma membranes of sperm and egg that occurs at fertilization (discussed in Chapter 20) and the fusion of myoblasts during muscle cell development (discussed in Chapter 21). All cell membrane fusions require special proteins and are subject to tight controls, which ensure that only appropriate membranes fuse. The controls are crucial for maintaining both the identity of cells and the individuality of each type of intracellular compartment.
The membrane fusions catalyzed by viral fusion proteins are the best understood. These proteins have a crucial role in permitting the entry of enveloped viruses (which have a lipid-bilayer-based membrane coat) into the cells that they infect (discussed in Chapters 5 and 25, CD). For example, viruses—such as human immunodeficiency virus (HIV), which causes AIDS—bind to cell-surface receptors, and then the viral and plasma membranes fuse (Figure 13-16). This fusion event allows the viral nucleic acid to enter the cytosol, where it replicates. Other viruses, such as influenza virus, first enter the cell by receptor-mediated endocytosis (discussed later) and are delivered to endosomes. In this case, the low pH in endosomes activates a fusion protein in the viral envelope that catalyzes the fusion of the viral and endosomal membranes. This likewise releases the viral nucleic acid into the cytosol.

Figure 13-16
The entry of enveloped viruses into cells. (A) Electron micrographs showing how HIV enters a cell by fusing its membrane with the plasma membrane of the cell. (B) A model for the HIV membrane-fusion process. HIV binds first to the CD4 protein on the surface (more...)
The three-dimensional structures of the fusion proteins of influenza virus and HIV provide valuable insights into the molecular mechanism of the membrane fusion catalyzed by these proteins. An exposure of the influenza fusion protein to low pH, or an exposure of HIV fusion protein to receptors on the target cell membrane, uncovers previously buried hydrophobic regions. These regions, called fusion peptides, are observed to then insert directly into the hydrophobic core of lipid bilayer of the target membrane. Thus, the viral fusion proteins are, for a moment, integral membrane proteins in two separate lipid bilayers. Structural rearrangements in the fusion proteins then bring the two lipid bilayers into very close apposition and destabilize them so that the bilayers fuse (see Figure 13-16). For viral fusion, the fusion proteins are the only components required, supporting the possibility that SNAREs are also the central players in the process of bilayer fusion in cells.
Summary
The differences between the many different membrane-enclosed compartments in a eucaryotic cell are maintained by directed, selective transport of particular membrane components from one compartment to another. Transport vesicles, which can be spherical or tubular, bud from specialized coated regions of the donor membrane. The assembly of the coat helps to collect specific membrane and soluble cargo molecules for transport and to drive the formation of the vesicle.
Of the various types of coated vesicles, the best characterized are clathrin-coated vesicles, which mediate transport from the plasma membrane and the trans Golgi network, and COPI- and COPII-coated vesicles, which mediate transport between the ER and the Golgi apparatus and between Golgi cisternae. In clathrin-coated vesicles, adaptins link the clathrin to the vesicle membrane and also trap specific cargo molecules for packaging into the vesicle. The coat is shed rapidly after budding, which is necessary for a vesicle to fuse with its appropriate target membrane.
Monomeric GTPases help regulate various steps in vesicular transport, including both vesicle budding and docking. The coat-recruitment GTPases, including Sar1 and the ARF proteins, regulate coat assembly and disassembly. A family of Rab proteins functions as vesicle targeting GTPases. Being incorporated with v-SNAREs into budding transport vesicles, the Rab proteins help ensure that the vesicles deliver their contents only to the appropriate membrane-enclosed compartment: the one that displays complementary t-SNARE proteins. Complementary v-SNARE and t-SNARE proteins form stable trans-SNARE complexes, thereby bringing their membrane bilayers into close apposition for fusion.
- There Are Various Types of Coated Vesicles
- The Assembly of a Clathrin Coat Drives Vesicle Formation
- Both The Pinching-off and Uncoating of Coated Vesicles Are Regulated Processes
- Not All Transport Vesicles are Spherical
- Monomeric GTPases Control Coat Assembly
- SNARE Proteins and Targeting GTPases Guide Membrane Transport
- Interacting SNAREs Need To Be Pried Apart Before They Can Function Again
- Rab Proteins Help Ensure the Specificity of Vesicle Docking
- SNAREs May Mediate Membrane Fusion
- Viral Fusion Proteins and SNAREs May Use Similar Strategies
- Summary
- The Molecular Mechanisms of Membrane Transport and the Maintenance of Compartmen...The Molecular Mechanisms of Membrane Transport and the Maintenance of Compartmental Diversity - Molecular Biology of the Cell
- protein Wnt-5b [Rattus norvegicus]protein Wnt-5b [Rattus norvegicus]gi|197304681|ref|NP_001093959.1|Protein
- Arthrobacter sp. 1152G49 16S ribosomal RNA gene, partial sequenceArthrobacter sp. 1152G49 16S ribosomal RNA gene, partial sequencegi|930625464|gb|KT803299.1|Nucleotide
Your browsing activity is empty.
Activity recording is turned off.
See more...