By agreement with the publisher, this book is accessible by the search feature, but cannot be browsed.
NCBI Bookshelf. A service of the National Library of Medicine, National Institutes of Health.
Alberts B, Johnson A, Lewis J, et al. Molecular Biology of the Cell. 4th edition. New York: Garland Science; 2002.
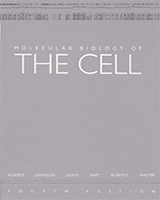
Molecular Biology of the Cell. 4th edition.
Show detailsMatter is made of combinations of elements—substances such as hydrogen or carbon that cannot be broken down or converted into other substances by chemical means. The smallest particle of an element that still retains its distinctive chemical properties is an atom. However, the characteristics of substances other than pure elements—including the materials from which living cells are made—depend on the way their atoms are linked together in groups to form molecules. In order to understand how living organisms are built from inanimate matter, therefore, it is crucial to know how all of the chemical bonds that hold atoms together in molecules are formed.
Cells Are Made From a Few Types of Atoms
Each atom has at its center a positively charged nucleus, which is surrounded at some distance by a cloud of negatively charged electrons, held in a series of orbitals by electrostatic attraction to the nucleus. The nucleus in turn consists of two kinds of subatomic particles: protons, which are positively charged, and neutrons, which are electrically neutral. The number of protons in the atomic nucleus gives the atomic number. An atom of hydrogen has a nucleus composed of a single proton; so hydrogen, with an atomic number of 1, is the lightest element. An atom of carbon has six protons in its nucleus and an atomic number of 6 (Figure 2-1). The electric charge carried by each proton is exactly equal and opposite to the charge carried by a single electron. Since an atom as a whole is electrically neutral, the number of negatively charged electrons surrounding the nucleus is equal to the number of positively charged protons that the nucleus contains; thus the number of electrons in an atom also equals the atomic number. It is these electrons that determine the chemical behavior of an atom, and all of the atoms of a given element have the same atomic number.

Figure 2-1
Highly schematic representations of an atom of carbon and an atom of hydrogen. Although the electrons are shown here as individual particles, in reality their behavior is governed by the laws of quantum mechanics, and there is no way of predicting exactly (more...)
Neutrons are uncharged subatomic particles of essentially the same mass as protons. They contribute to the structural stability of the nucleus—if there are too many or too few, the nucleus may disintegrate by radioactive decay—but they do not alter the chemical properties of the atom. Thus an element can exist in several physically distinguishable but chemically identical forms, called isotopes, each isotope having a different number of neutrons but the same number of protons. Multiple isotopes of almost all the elements occur naturally, including some that are unstable. For example, while most carbon on Earth exists as the stable isotope carbon 12, with six protons and six neutrons, there are also small amounts of an unstable isotope, the radioactive carbon 14, whose atoms have six protons and eight neutrons. Carbon 14 undergoes radioactive decay at a slow but steady rate. This forms the basis for a technique known as carbon 14 dating, which is used in archaeology to determine the time of origin of organic materials.
The atomic weight of an atom, or the molecular weight of a molecule, is its mass relative to that of a hydrogen atom. This is essentially equal to the number of protons plus neutrons that the atom or molecule contains, since the electrons are much lighter and contribute almost nothing to the total. Thus the major isotope of carbon has an atomic weight of 12 and is symbolized as 12C, whereas the unstable isotope just discussed has an atomic weight of 14 and is written as 14C. The mass of an atom or a molecule is often specified in daltons, one dalton being an atomic mass unit approximately equal to the mass of a hydrogen atom.
Atoms are so small that it is hard to imagine their size. An individual carbon atom is roughly 0.2 nm in diameter, so that it would take about 5 million of them, laid out in a straight line, to span a millimeter. One proton or neutron weighs approximately 1/(6 × 1023) gram, so one gram of hydrogen contains 6 × 1023 atoms. This huge number (6 × 1023, called Avogadro's number) is the key scale factor describing the relationship between everyday quantities and quantities measured in terms of individual atoms or molecules. If a substance has a molecular weight of X, 6 × 1023 molecules of it will have a mass of X grams. This quantity is called one mole of the substance (Figure 2-2).

Figure 2-2
Moles and molar solutions.
There are 92 naturally occurring elements, each differing from the others in the number of protons and electrons in its atoms. Living organisms, however, are made of only a small selection of these elements, four of which—carbon (C), hydrogen (H), nitrogen (N), and oxygen (O)—make up 96.5% of an organism's weight. This composition differs markedly from that of the nonliving inorganic environment (Figure 2-3) and is evidence of a distinctive type of chemistry. The most common elements in living organisms are listed in Table 2-1 with some of their atomic characteristics.

Figure 2-3
The abundances of some chemical elements in the nonliving world (the Earth's crust) compared with their abundances in the tissues of an animal. The abundance of each element is expressed as a percentage of the total number of atoms present in the sample. (more...)
Table 2-1
Atomic Characteristics of the Most Abundant Elements in Living Tissues.
The Outermost Electrons Determine How Atoms Interact
To understand how atoms bond together to form the molecules that make up living organisms, we have to pay special attention to their electrons. Protons and neutrons are welded tightly to one another in the nucleus and change partners only under extreme conditions—during radioactive decay, for example, or in the interior of the sun or of a nuclear reactor. In living tissues, it is only the electrons of an atom that undergo rearrangements. They form the exterior of an atom and specify the rules of chemistry by which atoms combine to form molecules.
Electrons are in continuous motion around the nucleus, but motions on this submicroscopic scale obey different laws from those we are familiar with in everyday life. These laws dictate that electrons in an atom can exist only in certain discrete states, called orbitals, and that there is a strict limit to the number of electrons that can be accommodated in an orbital of a given type—a so-called electron shell. The electrons closest on average to the positive nucleus are attracted most strongly to it and occupy the innermost, most tightly bound shell. This shell can hold a maximum of two electrons. The second shell is farther away from the nucleus, and its electrons are less tightly bound. This second shell can hold up to eight electrons. The third shell contains electrons that are even less tightly bound; it can also hold up to eight electrons. The fourth and fifth shells can hold 18 electrons each. Atoms with more than four shells are very rare in biological molecules.
The electron arrangement of an atom is most stable when all the electrons are in the most tightly bound states that are possible for them—that is, when they occupy the innermost shells. Therefore, with certain exceptions in the larger atoms, the electrons of an atom fill the orbitals in order—the first shell before the second, the second before the third, and so on. An atom whose outermost shell is entirely filled with electrons is especially stable and therefore chemically unreactive. Examples are helium with 2 electrons, neon with 2 + 8, and argon with 2 + 8 + 8; these are all inert gases. Hydrogen, by contrast, with only one electron and therefore only a half-filled shell, is highly reactive. Likewise, the other atoms found in living tissues all have incomplete outer electron shells and are therefore able to donate, accept, or share electrons with each other to form both molecules and ions (Figure 2-4).

Figure 2-4
Filled and unfilled electron shells in some common elements. All the elements commonly found in living organisms have unfilled outermost shells (red) and can thus participate in chemical reactions with other atoms. For comparison, some elements that have (more...)
Because an unfilled electron shell is less stable than a filled one, atoms with incomplete outer shells have a strong tendency to interact with other atoms in a way that causes them to either gain or lose enough electrons to achieve a completed outermost shell. This electron exchange can be achieved either by transferring electrons from one atom to another or by sharing electrons between two atoms. These two strategies generate two types of chemical bonds between atoms: an ionic bond is formed when electrons are donated by one atom to another, whereas a covalent bond is formed when two atoms share a pair of electrons (Figure 2-5). Often, the pair of electrons is shared unequally, with a partial transfer between the atoms; this intermediate strategy results in a polar covalent bond, as we shall discuss later.

Figure 2-5
Comparison of covalent and ionic bonds. Atoms can attain a more stable arrangement of electrons in their outermost shell by interacting with one another. An ionic bond is formed when electrons are transferred from one atom to the other. A covalent bond (more...)
An H atom, which needs only one more electron to fill its shell, generally acquires it by electron sharing, forming one covalent bond with another atom; in many cases this bond is polar. The other most common elements in living cells—C, N, and O, with an incomplete second shell, and P and S, with an incomplete third shell (see Figure 2-4)—generally share electrons and achieve a filled outer shell of eight electrons by forming several covalent bonds. The number of electrons that an atom must acquire or lose (either by sharing or by transfer) to attain a filled outer shell is known as its valence.
The crucial role of the outer electron shell in determining the chemical properties of an element means that, when the elements are listed in order of their atomic number, there is a periodic recurrence of elements with similar properties: an element with, say, an incomplete second shell containing one electron will behave in much the same way as an element that has filled its second shell and has an incomplete third shell containing one electron. The metals, for example, have incomplete outer shells with just one or a few electrons, whereas, as we have just seen, the inert gases have full outer shells.
Ionic Bonds Form by the Gain and Loss of Electrons
Ionic bonds are most likely to be formed by atoms that have just one or two electrons in addition to a filled outer shell or are just one or two electrons short of acquiring a filled outer shell. They can often attain a completely filled outer electron shell more easily by transferring electrons to or from another atom than by sharing electrons. For example, from Figure 2-4 we see that a sodium (Na) atom, with atomic number 11, can strip itself down to a filled shell by giving up the single electron external to its second shell. By contrast, a chlorine (Cl) atom, with atomic number 17, can complete its outer shell by gaining just one electron. Consequently, if a Na atom encounters a Cl atom, an electron can jump from the Na to the Cl, leaving both atoms with filled outer shells. The offspring of this marriage between sodium, a soft and intensely reactive metal, and chlorine, a toxic green gas, is table salt (NaCl).
When an electron jumps from Na to Cl, both atoms become electrically charged ions. The Na atom that lost an electron now has one less electron than it has protons in its nucleus; it therefore has a single positive charge (Na+). The Cl atom that gained an electron now has one more electron than it has protons and has a single negative charge (Cl-). Positive ions are called cations, and negative ions, anions. Ions can be further classified according to how many electrons are lost or gained. Thus sodium and potassium (K) have one electron to lose and form cations with a single positive charge (Na+ and K+), whereas magnesium and calcium have two electrons to lose and form cations with two positive charges (Mg2+ and Ca2+).
Because of their opposite charges, Na+ and Cl- are attracted to each other and are thereby held together in an ionic bond. A salt crystal contains astronomical numbers of Na+ and Cl- (about 2 × 1019 ions of each type in a crystal 1 mm across) packed together in a precise three-dimensional array with their opposite charges exactly balanced (Figure 2-6). Substances such as NaCl, which are held together solely by ionic bonds, are generally called salts rather than molecules. Ionic bonds are just one of several types of noncovalent bonds that can exist between atoms, and we shall meet other examples.

Figure 2-6
Sodium chloride: an example of ionic bond formation. (A) An atom of sodium (Na) reacts with an atom of chlorine (Cl). Electrons of each atom are shown schematically in their different energy levels; electrons in the chemically reactive (incompletely filled) (more...)
Because of a favorable interaction between water molecules and ions, ionic bonds are greatly weakened by water; thus many salts (including NaCl) are highly soluble in water—dissociating into individual ions (such as Na+ and Cl-), each surrounded by a group of water molecules. In contrast, covalent bond strengths are not affected in this way.
Covalent Bonds Form by the Sharing of Electrons
All the characteristics of a cell depend on the molecules it contains. A molecule is defined as a cluster of atoms held together by covalent bonds; here electrons are shared between atoms to complete the outer shells, rather than being transferred between them. In the simplest possible molecule—a molecule of hydrogen (H2)—two H atoms, each with a single electron, share two electrons, which is the number required to fill the first shell. These shared electrons form a cloud of negative charge that is densest between the two positively charged nuclei and helps to hold them together, in opposition to the mutual repulsion between like charges that would otherwise force them apart. The attractive and repulsive forces are in balance when the nuclei are separated by a characteristic distance, called the bond length.
A further crucial property of any bond—covalent or noncovalent—is its strength. Bond strength is measured by the amount of energy that must be supplied to break that bond. This is often expressed in units of kilocalories per mole (kcal/mole), where a kilocalorie is the amount of energy needed to raise the temperature of one liter of water by one degree centigrade. Thus if 1 kilocalorie must be supplied to break 6 × 1023 bonds of a specific type (that is, 1 mole of these bonds), then the strength of that bond is 1 kcal/mole. An equivalent, widely used measure of energy is the kilojoule, which is equal to 0.239 kilocalories.
To get an idea of what bond strengths mean, it is helpful to compare them with the average energies of the impacts that molecules are constantly undergoing from collisions with other molecules in their environment (their thermal, or heat, energy), as well as with other sources of biological energy such as light and glucose oxidation (Figure 2-7). Typical covalent bonds are stronger than the thermal energies by a factor of 100, so they are resistant to being pulled apart by thermal motions and are normally broken only during specific chemical reactions with other atoms and molecules. The making and breaking of covalent bonds are violent events, and in living cells they are carefully controlled by highly specific catalysts, called enzymes. Noncovalent bonds as a rule are much weaker; we shall see later that they are important in the cell in the many situations where molecules have to associate and dissociate readily to carry out their functions.

Figure 2-7
Some energies important for cells. Note that these energies are compared on a logarithmic scale.
Whereas an H atom can form only a single covalent bond, the other common atoms that form covalent bonds in cells—O, N, S, and P, as well as the all-important C atom—can form more than one. The outermost shell of these atoms, as we have seen, can accommodate up to eight electrons, and they form covalent bonds with as many other atoms as necessary to reach this number. Oxygen, with six electrons in its outer shell, is most stable when it acquires an extra two electrons by sharing with other atoms and therefore forms up to two covalent bonds. Nitrogen, with five outer electrons, forms a maximum of three covalent bonds, while carbon, with four outer electrons, forms up to four covalent bonds—thus sharing four pairs of electrons (see Figure 2-4).
When one atom forms covalent bonds with several others, these multiple bonds have definite orientations in space relative to one another, reflecting the orientations of the orbits of the shared electrons. Covalent bonds between multiple atoms are therefore characterized by specific bond angles as well as bond lengths and bond energies (Figure 2-8). The four covalent bonds that can form around a carbon atom, for example, are arranged as if pointing to the four corners of a regular tetrahedron. The precise orientation of covalent bonds forms the basis for the three-dimensional geometry of organic molecules.

Figure 2-8
The geometry of covalent bonds. (A) The spatial arrangement of the covalent bonds that can be formed by oxygen, nitrogen, and carbon. (B) Molecules formed from these atoms have a precise three-dimensional structure, as shown here by ball and stick models (more...)
There Are Different Types of Covalent Bonds
Most covalent bonds involve the sharing of two electrons, one donated by each participating atom; these are called single bonds. Some covalent bonds, however, involve the sharing of more than one pair of electrons. Four electrons can be shared, for example, two coming from each participating atom; such a bond is called a double bond. Double bonds are shorter and stronger than single bonds and have a characteristic effect on the three-dimensional geometry of molecules containing them. A single covalent bond between two atoms generally allows the rotation of one part of a molecule relative to the other around the bond axis. A double bond prevents such rotation, producing a more rigid and less flexible arrangement of atoms (Figure 2-9 and Panel 2-1, pp. 111–112).

Figure 2-9
Carbon-carbon double bonds and single bonds compared. (A) The ethane molecule, with a single covalent bond between the two carbon atoms, illustrates the tetrahedral arrangement of single covalent bonds formed by carbon. One of the CH3 groups joined by (more...)
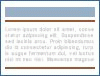
Panel 2-1
Chemical Bonds and Groups Commonly Encountered in Biological Molecules.
Some molecules share electrons between three or more atoms, producing bonds that have a hybrid character intermediate between single and double bonds. The highly stable benzene molecule, for example, comprises a ring of six carbon atoms in which the bonding electrons are evenly distributed (although usually depicted as an alternating sequence of single and double bonds, as shown in Panel 2-1).
When the atoms joined by a single covalent bond belong to different elements, the two atoms usually attract the shared electrons to different degrees. Compared with a C atom, for example, O and N atoms attract electrons relatively strongly, whereas an H atom attracts electrons more weakly. By definition, a polar structure (in the electrical sense) is one with positive charge concentrated toward one end (the positive pole) and negative charge concentrated toward the other (the negative pole). Covalent bonds in which the electrons are shared unequally in this way are therefore known as polar covalent bonds (Figure 2-10). For example, the covalent bond between oxygen and hydrogen, -O-H, or between nitrogen and hydrogen, -N-H, is polar, whereas that between carbon and hydrogen, -C-H, has the electrons attracted much more equally by both atoms and is relatively nonpolar.

Figure 2-10
Polar and nonpolar covalent bonds. The electron distributions in the polar water molecule (H2O) and the nonpolar oxygen molecule (O2) are compared (δ+, partial positive charge; δ-, partial negative charge).
Polar covalent bonds are extremely important in biology because they create permanent dipoles that allow molecules to interact through electrical forces. Any large molecule with many polar groups will have a pattern of partial positive and negative charges on its surface. When such a molecule encounters a second molecule with a complementary set of charges, the two molecules will be attracted to each other by permanent dipole interactions that resemble (but are weaker than) the ionic bonds discussed previously for NaCl.
An Atom Often Behaves as if It Has a Fixed Radius
When a covalent bond forms between two atoms, the sharing of electrons brings the nuclei of these atoms unusually close together. But most of the atoms that are rapidly jostling each other in cells are located in separate molecules. What happens when two such atoms touch?
For simplicity and clarity, atoms and molecules are usually represented in a highly schematic way—either as a line drawing of the structural formula or as a ball and stick model. However, a more accurate representation can be obtained through the use of so-called space-filling models. Here a solid envelope is used to represent the radius of the electron cloud at which strong repulsive forces prevent a closer approach of any second, non-bonded atom—the so-called van der Waals radius for an atom. This is possible because the amount of repulsion increases very steeply as two such atoms approach each other closely. At slightly greater distances, any two atoms will experience a weak attractive force, known as a van der Waals attraction. As a result, there is a distance at which repulsive and attractive forces precisely balance to produce an energy minimum in each atom's interaction with an atom of a second, non-bonded element (Figure 2-11).

Figure 2-11
The balance of van der Waals forces between two atoms. As the nuclei of two atoms approach each other, they initially show a weak bonding interaction due to their fluctuating electric charges. However, the same atoms will strongly repel each other if (more...)
Depending on the intended purpose, we shall represent small molecules either as line drawings, ball and stick models, or space filling models throughout this book. For comparison, the water molecule is represented in all three ways in Figure 2-12. When dealing with very large molecules, such as proteins, we shall often need to further simplify the representation used (see, for example, Panel 3-2, pp. 138–139).

Figure 2-12
Three representations of a water molecule. (A) The usual line drawing of the structural formula, in which each atom is indicated by its standard symbol, and each line represents a covalent bond joining two atoms. (B) A ball and stick model, in which atoms (more...)
Water Is the Most Abundant Substance in Cells
Water accounts for about 70% of a cell's weight, and most intracellular reactions occur in an aqueous environment. Life on Earth began in the ocean, and the conditions in that primeval environment put a permanent stamp on the chemistry of living things. Life therefore hinges on the properties of water.
In each water molecule (H2O) the two H atoms are linked to the O atom by covalent bonds (see Figure 2-12). The two bonds are highly polar because the O is strongly attractive for electrons, whereas the H is only weakly attractive. Consequently, there is an unequal distribution of electrons in a water molecule, with a preponderance of positive charge on the two H atoms and of negative charge on the O (see Figure 2-10). When a positively charged region of one water molecule (that is, one of its H atoms) comes close to a negatively charged region (that is, the O) of a second water molecule, the electrical attraction between them can result in a weak bond called a hydrogen bond. These bonds are much weaker than covalent bonds and are easily broken by the random thermal motions due to the heat energy of the molecules, so each bond lasts only an exceedingly short time. But the combined effect of many weak bonds is far from trivial. Each water molecule can form hydrogen bonds through its two H atoms to two other water molecules, producing a network in which hydrogen bonds are being continually broken and formed (Panel 2-2, pp. 112–113). It is only because of the hydrogen bonds that link water molecules together that water is a liquid at room temperature, with a high boiling point and high surface tension—rather than a gas.
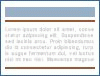
Panel 2-2
Water and Its Influence on the Behavior of Biological Molecules.
Molecules, such as alcohols, that contain polar bonds and that can form hydrogen bonds with water dissolve readily in water. As mentioned previously, molecules carrying plus or minus charges (ions) likewise interact favorably with water. Such molecules are termed hydrophilic, meaning that they are water-loving. A large proportion of the molecules in the aqueous environment of a cell necessarily fall into this category, including sugars, DNA, RNA, and a majority of proteins. Hydrophobic (water-hating) molecules, by contrast, are uncharged and form few or no hydrogen bonds, and so do not dissolve in water. Hydrocarbons are an important example (see Panel 2-1, pp. 110–111). In these molecules the H atoms are covalently linked to C atoms by a largely nonpolar bond. Because the H atoms have almost no net positive charge, they cannot form effective hydrogen bonds to other molecules. This makes the hydrocarbon as a whole hydrophobic—a property that is exploited in cells, whose membranes are constructed from molecules that have long hydrocarbon tails, as we shall see in Chapter 10.
Some Polar Molecules Form Acids and Bases in Water
One of the simplest kinds of chemical reaction, and one that has profound significance in cells, takes place when a molecule possessing a highly polar covalent bond between a hydrogen and a second atom dissolves in water. The hydrogen atom in such a molecule has largely given up its electron to the companion atom and so exists as an almost naked positively charged hydrogen nucleus—in other words, a proton (H + ) . When the polar molecule becomes surrounded by water molecules, the proton is attracted to the partial negative charge on the O atom of an adjacent water molecule and can dissociate from its original partner to associate instead with the oxygen atoms of the water molecule to generate a hydronium ion (H 3 O + ) (Figure 2-13A). The reverse reaction also takes place very readily, so one has to imagine an equilibrium state in which billions of protons are constantly flitting to and fro from one molecule in the solution to another.

Figure 2-13
Acids in water. (A) The reaction that takes place when a molecule of acetic acid dissolves in water. (B) Water molecules are continuously exchanging protons with each other to form hydronium and hydroxyl ions. These ions in turn rapidly recombine to form (more...)
Substances that release protons to form H3O+ when they dissolve in water are termed acids. The higher the concentration of H3O+, the more acidic the solution. H3O+ is present even in pure water, at a concentration of 10-7 M, as a result of the movement of protons from one water molecule to another (Figure 2-13B). By tradition, the H3O+ concentration is usually referred to as the H+ concentration, even though most H+ in an aqueous solution is present as H3O+. To avoid the use of unwieldy numbers, the concentration of H+ is expressed using a logarithmic scale called the pH scale, as illustrated in Panel 2-2 (pp. 112–113). Pure water has a pH of 7.0.
Because the proton of a hydronium ion can be passed readily to many types of molecules in cells, altering their character, the concentration of H3O+ inside a cell (the acidity) must be closely regulated. Molecules that can give up protons will do so more readily if the concentration of H3O+ in solution is low and will tend to receive them back if the concentration in solution is high.
The opposite of an acid is a base. Just as the defining property of an acid is that it donates protons to a water molecule so as to raise the concentration of H3O+ ions, the defining property of a base is that it raises the concentration of hydroxyl (OH-) ions—which are formed by removal of a proton from a water molecule. Thus sodium hydroxide (NaOH) is basic (the term alkaline is also used) because it dissociates in aqueous solution to form Na+ ions and OH- ions. Another class of bases, especially important in living cells, are those that contain NH2 groups. These groups can generate OH- by taking a proton from water: -NH2 + H2O → -NH3 + + OH-.
Because an OH- ion combines with a H3O+ ion to form two water molecules, an increase in the OH- concentration forces a decrease in the concentration of H3O+, and vice versa. A pure solution of water contains an equally low concentration (10-7 M) of both ions; it is neither acidic nor basic and is therefore said to be neutral with a pH of 7.0. The inside of cells is kept close to neutrality.
Four Types of Noncovalent Interactions Help Bring Molecules Together in Cells
In aqueous solutions, covalent bonds are 10 to 100 times stronger than the other attractive forces between atoms, allowing their connections to define the boundaries of one molecule from another. But much of biology depends on the specific binding of different molecules to each other. This binding is mediated by a group of noncovalent attractions that are individually quite weak, but whose bond energies can sum to create an effective force between two separate molecules. We have already introduced three of these noncovalent forces: ionic bonds, hydrogen bonds and van der Waals attractions. In Table 2-2, the strengths of these three types of bonds are compared to that of a typical covalent bond, both in the presence and the absence of water. Because of their fundamental importance in all biological systems, we shall summarize their properties here.
Table 2-2
Covalent and Noncovalent Chemical Bonds.
- Ionic bonds. These are purely electrostatic attractions between oppositely charged atoms. As we saw for NaCl, these forces are quite strong in the absence of water. However, the polar water molecules cluster around both fully charged ions and polar molecules that contain permanent dipoles (Figure 2-14). This greatly reduces the potential attractiveness of these charged species for each other (see Table 2-2).
- Hydrogen bonds. The structure of a typical hydrogen bond is illustrated in Figure 2-15. This bond represents a special form of polar interaction in which an electropositive hydrogen atom is partially shared by two electronegative atoms. Its hydrogen can be viewed as a proton that has partially dissociated from a donor atom, allowing it to be shared by a second acceptor atom. Unlike a typical electrostatic interaction, this bond is highly directional—being strongest when a straight line can be drawn between all three of the involved atoms. As already discussed, water weakens these bonds by forming competing hydrogen-bond interactions with the involved molecules (see Table 2-2).
- van der Waals attractions. The electron cloud around any nonpolar atom will fluctuate, producing a flickering dipole. Such dipoles will transiently induce an oppositely polarized flickering dipole in a nearby atom. This interaction generates an attraction between atoms that is very weak. But since many atoms can be simultaneously in contact when two surfaces fit closely, the net result is often significant. These so-called van der Waals attractions are not weakened by water (see Table 2-2).

Figure 2-14
How the dipoles on water molecules orient to reduce the affinity of oppositely charged ions or polar groups for each other.

Figure 2-15
Hydrogen bonds. (A) Ball- and-stick model of a typical hydrogen bond. The distance between the hydrogen and the oxygen atom here is less than the sum of their van der Waals radii, indicating a partial sharing of electrons. (B) The most common hydrogen (more...)
The fourth effect that can play an important part in bringing molecules together in water is a hydrophobic force. This force is caused by a pushing of nonpolar surfaces out of the hydrogen-bonded water network, where they would physically interfere with the highly favorable interactions between water molecules. Because bringing two nonpolar surfaces together reduces their contact with water, the force is a rather nonspecific one. Nevertheless, we shall see in Chapter 3 that hydrophobic forces are central to the proper folding of protein molecules.
Panel 2-3 provides an overview of the four types of interactions just described. And Figure 2-16 illustrates, in a schematic way, how many such interactions can sum to hold together the matching surfaces of two macromolecules, even though each interaction by itself would be much too weak to be effective.
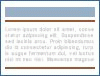
Panel 2-3
The Principal Types of Weak Noncovalent Bonds that Hold Macromolecules Together.

Figure 2-16
How two macro-molecules with complementary surfaces can bind tightly to one another through noncovalent interactions. In this schematic illustration, plus and minus are used to mark chemical groups that can form attractive interactions when paired.
A Cell Is Formed from Carbon Compounds
Having looked at the ways atoms combine into small molecules and how these molecules behave in an aqueous environment, we now examine the main classes of small molecules found in cells and their biological roles. We shall see that a few basic categories of molecules, formed from a handful of different elements, give rise to all the extraordinary richness of form and behavior shown by living things.
If we disregard water, nearly all the molecules in a cell are based on carbon. Carbon is outstanding among all the elements in its ability to form large molecules; silicon is a poor second. Because it is small and has four electrons and four vacancies in its outermost shell, a carbon atom can form four covalent bonds with other atoms. Most important, one carbon atom can join to other carbon atoms through highly stable covalent C-C bonds to form chains and rings and hence generate large and complex molecules with no obvious upper limit to their size (see Panel 2-1, pp. 110–111). The small and large carbon compounds made by cells are called organic molecules.
Certain combinations of atoms, such as the methyl (-CH3), hydroxyl (-OH), carboxyl (-COOH), carbonyl (-C=O), phosphate (-PO3 2-), and amino (-NH2) groups, occur repeatedly in organic molecules. Each such chemical group has distinct chemical and physical properties that influence the behavior of the molecule in which the group occurs. The most common chemical groups and some of their properties are summarized in Panel 2-1, pp. 110–111.
Cells Contain Four Major Families of Small Organic Molecules
The small organic molecules of the cell are carbon-based compounds that have molecular weights in the range 100 to 1000 and contain up to 30 or so carbon atoms. They are usually found free in solution and have many different fates. Some are used as monomer subunits to construct the giant polymeric macromolecules—the proteins, nucleic acids, and large polysaccharides—of the cell. Others act as energy sources and are broken down and transformed into other small molecules in a maze of intracellular metabolic pathways. Many small molecules have more than one role in the cell—for example, acting both as a potential subunit for a macromolecule and as an energy source. Small organic molecules are much less abundant than the organic macromolecules, accounting for only about one-tenth of the total mass of organic matter in a cell (Table 2-3). As a rough guess, there may be a thousand different kinds of these small molecules in a typical cell.
Table 2-3
The Approximate Chemical Composition of a Bacterial Cell.
All organic molecules are synthesized from and are broken down into the same set of simple compounds. Both their synthesis and their breakdown occur through sequences of chemical changes that are limited in scope and follow definite rules. As a consequence, the compounds in a cell are chemically related and most can be classified into a small number of distinct families. Broadly speaking, cells contain four major families of small organic molecules: the sugars, the fatty acids, the amino acids, and the nucleotides (Figure 2-17). Although many compounds present in cells do not fit into these categories, these four families of small organic molecules, together with the macromolecules made by linking them into long chains, account for a large fraction of cell mass (see Table 2-3).

Figure 2-17
The four main families of small organic molecules in cells. These small molecules form the monomeric building blocks, or subunits, for most of the macromolecules and other assemblies of the cell. Some, like the sugars and the fatty acids, are also energy (more...)
Sugars Provide an Energy Source for Cells and Are the Subunits of Polysaccharides
The simplest sugars—the monosaccharides—are compounds with the general formula (CH2O)n, where n is usually 3, 4, 5, 6, 7, or 8. Sugars, and the molecules made from them, are also called carbohydrates because of this simple formula. Glucose, for example, has the formula C6H12O6 (Figure 2-18). The formula, however, does not fully define the molecule: the same set of carbons, hydrogens, and oxygens can be joined together by covalent bonds in a variety of ways, creating structures with different shapes. As shown in Panel 2-4 (pp. 116–117), for example, glucose can be converted into a different sugar—mannose or galactose—simply by switching the orientations of specific OH groups relative to the rest of the molecule. Each of these sugars, moreover, can exist in either of two forms, called the d-form and the l-form, which are mirror images of each other. Sets of molecules with the same chemical formula but different structures are called isomers, and the subset of such molecules that are mirror-image pairs are called optical isomers. Isomers are widespread among organic molecules in general, and they play a major part in generating the enormous variety of sugars.

Figure 2-18
The structure of glucose, a simple sugar. As illustrated previously for water (see Figure 2-12), any molecule can be represented in several ways. In the structural formulas shown in (A), (B) and (E), the atoms are shown as chemical symbols linked together (more...)
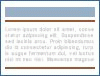
Panel 2-4
An Outline of Some of the Types of Sugars Commonly Found in Cells.
An outline of sugar structures and chemistry is given in Panel 2-4. Sugars can exist in either a ring or an open-chain form. In their open-chain form, sugars contain a number of hydroxyl groups and either one aldehyde (H>C=O) or one ketone ( C=O) group. The aldehyde or ketone group plays a special role. First, it can react with a hydroxyl group in the same molecule to convert the molecule into a ring; in the ring form the carbon of the original aldehyde or ketone group can be recognized as the only one that is bonded to two oxygens. Second, once the ring is formed, this same carbon can become further linked to one of the carbons bearing a hydroxyl group on another sugar molecule, creating a disaccharide; such as sucrose, which is composed of a glucose and a fructose unit. Larger sugar polymers range from the oligosaccharides (trisaccharides, tetrasaccharides, and so on) up to giant polysaccharides, which can contain thousands of monosaccharide units.
The way that sugars are linked together to form polymers illustrates some common features of biochemical bond formation. A bond is formed between an -OH group on one sugar and an -OH group on another by a condensation reaction, in which a molecule of water is expelled as the bond is formed (Figure 2-19). Subunits in other biological polymers, such as nucleic acids and proteins, are also linked by condensation reactions in which water is expelled. The bonds created by all of these condensation reactions can be broken by the reverse process of hydrolysis, in which a molecule of water is consumed (see Figure 2-19).

Figure 2-19
The reaction of two monosaccharides to form a disaccharide. This reaction belongs to a general category of reactions termed condensation reactions, in which two molecules join together as a result of the loss of a water molecule. The reverse reaction (more...)
Because each monosaccharide has several free hydroxyl groups that can form a link to another monosaccharide (or to some other compound), sugar polymers can be branched, and the number of possible polysaccharide structures is extremely large. Even a simple disaccharide consisting of two glucose residues can exist in eleven different varieties (Figure 2-20), while three different hexoses (C6H12O6) can join together to make several thousand trisaccharides. For this reason it is a much more complex task to determine the arrangement of sugars in a polysaccharide than to determine the nucleotide sequence of a DNA molecule, where each unit is joined to the next in exactly the same way.

Figure 2-20
Eleven disaccharides consisting of two D-glucose units. Although these differ only in the type of linkage between the two glucose units, they are chemically distinct. Since the oligosaccharides associated with proteins and lipids may have six or more (more...)
The monosaccharide glucose has a central role as an energy source for cells. In a series of reactions, it is broken down to smaller molecules, releasing energy that the cell can harness to do useful work, as we shall explain later. Cells use simple polysaccharides composed only of glucose units—principally glycogen in animals and starch in plants—as long-term stores of energy.
Sugars do not function only in the production and storage of energy. They also can be used, for example, to make mechanical supports. Thus, the most abundant organic chemical on Earth—the cellulose of plant cell walls—is a polysaccharide of glucose. Another extraordinarily abundant organic substance, the chitin of insect exoskeletons and fungal cell walls, is also a polysaccharide—in this case a linear polymer of a sugar derivative called N-acetylglucosamine. Polysaccharides of various other sorts are the main components of slime, mucus, and gristle.
Smaller oligosaccharides can be covalently linked to proteins to form glycoproteins and to lipids to form glycolipids, which are found in cell membranes. As described in Chapter 10, the surfaces of most cells are clothed and decorated with sugar polymers belonging to glycoproteins and glycolipids in the cell membrane. These sugar side chains are often recognized selectively by other cells. And differences between people in the details of their cell-surface sugars are the molecular basis for the major different human blood groups.
Fatty Acids Are Components of Cell Membranes
A fatty acid molecule, such as palmitic acid, has two chemically distinct regions (Figure 2-21). One is a long hydrocarbon chain, which is hydrophobic and not very reactive chemically. The other is a carboxyl (-COOH) group, which behaves as an acid (carboxylic acid): it is ionized in solution (-COO-), extremely hydrophilic, and chemically reactive. Almost all the fatty acid molecules in a cell are covalently linked to other molecules by their carboxylic acid group.

Figure 2-21
A fatty acid. A fatty acid is composed of a hydrophobic hydrocarbon chain to which is attached a hydrophilic carboxylic acid group. Palmitic acid is shown here. Different fatty acids have different hydrocarbon tails. (A) Structural formula. The carboxylic (more...)
The hydrocarbon tail of palmitic acid is saturated: it has no double bonds between carbon atoms and contains the maximum possible number of hydrogens. Stearic acid, another one of the common fatty acids in animal fat, is also saturated. Some other fatty acids, such as oleic acid, have unsaturated tails, with one or more double bonds along their length. The double bonds create kinks in the molecules, interfering with their ability to pack together in a solid mass. It is this that accounts for the difference between hard (saturated) and soft (polyunsaturated) margarine. The many different fatty acids found in cells differ only in the length of their hydrocarbon chains and the number and position of the carbon-carbon double bonds (see Panel 2-5, pp. 118–119).
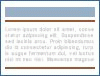
Panel 2-5
Fatty Acids and Other Lipids.
Fatty acids serve as a concentrated food reserve in cells, because they can be broken down to produce about six times as much usable energy, weight for weight, as glucose. They are stored in the cytoplasm of many cells in the form of droplets of triacylglycerol molecules, which consist of three fatty acid chains joined to a glycerol molecule (see Panel 2-5); these molecules are the animal fats found in meat, butter, and cream, and the plant oils like corn oil and olive oil. When required to provide energy, the fatty acid chains are released from triacylglycerols and broken down into two-carbon units. These two-carbon units are identical to those derived from the breakdown of glucose and they enter the same energy-yielding reaction pathways, as will be described later in this chapter.
Fatty acids and their derivatives such as triacylglycerols are examples of lipids. Lipids comprise a loosely defined collection of biological molecules with the common feature that they are insoluble in water, while being soluble in fat and organic solvents such as benzene. They typically contain either long hydrocarbon chains, as in the fatty acids and isoprenes, or multiple linked aromatic rings, as in the steroids.
The most important function of fatty acids in cells is in the construction of cell membranes. These thin sheets enclose all cells and surround their internal organelles. They are composed largely of phospholipids, which are small molecules that, like triacylglycerols, are constructed mainly from fatty acids and glycerol. In phospholipids the glycerol is joined to two fatty acid chains, however, rather than to three as in triacylglycerols. The “third” site on the glycerol is linked to a hydrophilic phosphate group, which is in turn attached to a small hydrophilic compound such as choline (see Panel 2-5). Each phospholipid molecule, therefore, has a hydrophobic tail composed of the two fatty acid chains and a hydrophilic head, where the phosphate is located. This gives them different physical and chemical properties from triacylglycerols, which are predominantly hydrophobic. Molecules like phospholipids, with both hydrophobic and hydrophilic regions, are termed amphipathic.
The membrane-forming property of phospholipids results from their amphipathic nature. Phospholipids will spread over the surface of water to form a monolayer of phospholipid molecules, with the hydrophobic tails facing the air and the hydrophilic heads in contact with the water. Two such molecular layers can readily combine tail-to-tail in water to make a phospholipid sandwich, or lipid bilayer. This bilayer is the structural basis of all cell membranes (Figure 2-22).

Figure 2-22
Phospholipid structure and the orientation of phospholipids in membranes. In an aqueous environment, the hydrophobic tails of phospholipids pack together to exclude water. Here they have formed a bilayer with the hydrophilic head of each phospholipid (more...)
Amino Acids Are the Subunits of Proteins
Amino acids are a varied class of molecules with one defining property: they all possess a carboxylic acid group and an amino group, both linked to a single carbon atom called the α-carbon (Figure 2-23). Their chemical variety comes from the side chain that is also attached to the α-carbon. The importance of amino acids to the cell comes from their role in making proteins, which are polymers of amino acids joined head-to-tail in a long chain that is then folded into a three-dimensional structure unique to each type of protein. The covalent linkage between two adjacent amino acids in a protein chain is called a peptide bond; the chain of amino acids is also known as a polypeptide (Figure 2-24). Regardless of the specific amino acids from which it is made, the polypeptide has an amino (NH2) group at one end (its N-terminus) and a carboxyl (COOH) group at its other end (its C-terminus). This gives it a definite directionality—a structural (as opposed to an electrical) polarity.

Figure 2-23
The amino acid alanine. (A) In the cell, where the pH is close to 7, the free amino acid exists in its ionized form; but when it is incorporated into a polypeptide chain, the charges on the amino and carboxyl groups disappear. (B) A ball-and-stick model (more...)

Figure 2-24
A small part of a protein molecule. The four amino acids shown are linked together by three peptide bonds, one of which is highlighted in yellow. One of the amino acids is shaded in gray. The amino acid side chains are shown in red. The two ends of a (more...)
Twenty types of amino acids are found commonly in proteins, each with a different side chain attached to the α-carbon atom (see Panel 3-1, pp. 132–133). The same 20 amino acids occur over and over again in all proteins, whether from bacteria, plants, or animals. How this precise set of 20 amino acids came to be chosen is one of the mysteries surrounding the evolution of life; there is no obvious chemical reason why other amino acids could not have served just as well. But once the choice was established, it could not be changed; too much depended on it.
Like sugars, all amino acids, except glycine, exist as optical isomers in d- and l-forms (see Panel 3-1). But only l-forms are ever found in proteins (although d-amino acids occur as part of bacterial cell walls and in some antibiotics). The origin of this exclusive use of l-amino acids to make proteins is another evolutionary mystery.
The chemical versatility that the 20 standard amino acids provide is vitally important to the function of proteins. Five of the 20 amino acids have side chains that can form ions in solution and thereby can carry a charge (Figure 2-25). The others are uncharged; some are polar and hydrophilic, and some are nonpolar and hydrophobic. As we shall discuss in Chapter 3, the collective properties of the amino acid side chains underlie all the diverse and sophisticated functions of proteins.

Figure 2-25
The charge on amino acid side chains depends on the pH. The five different side chains that can carry a charge are shown. Carboxylic acids can readily lose H+ in aqueous solution to form a negatively charged ion, which is denoted by the suffix “-ate,” (more...)
Nucleotides Are the Subunits of DNA and RNA
A nucleotide is a molecule made up of a nitrogen-containing ring compound linked to a five-carbon sugar. This sugar can be either ribose or deoxyribose, and it carries one or more phosphate groups (Panel 2-6, pp. 120–121). Nucleotides containing ribose are known as ribonucleotides, and those containing deoxyribose as deoxyribonucleotides. The nitrogen-containing rings are generally referred to as bases for historical reasons: under acidic conditions they can each bind an H+ (proton) and thereby increase the concentration of OH- ions in aqueous solution. There is a strong family resemblance between the different bases. Cytosine (C), thymine (T), and uracil (U) are called pyrimidines because they all derive from a six-membered pyrimidine ring; guanine (G) and adenine (A) are purine compounds, and they have a second, five-membered ring fused to the six-membered ring. Each nucleotide is named from the base it contains (see Panel 2-6).
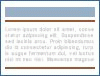
Panel 2-6
A Survey of the Nucleotides.
Nucleotides can act as short-term carriers of chemical energy. Above all others, the ribonucleotide adenosine triphosphate, or ATP (Figure 2-26), is used to transfer energy in hundreds of different cellular reactions. ATP is formed through reactions that are driven by the energy released by the oxidative breakdown of foodstuffs. Its three phosphates are linked in series by two phosphoanhydride bonds, whose rupture releases large amounts of useful energy. The terminal phosphate group in particular is frequently split off by hydrolysis, often transferring a phosphate to other molecules and releasing energy that drives energy-requiring biosynthetic reactions (Figure 2-27). Other nucleotide derivatives serve as carriers for the transfer of other chemical groups, as will be described later.

Figure 2-26
Chemical structure of adenosine triphosphate (ATP). (A) Structural formula. (B) Space-filling model. In (B) the colors of the atoms are C, black; N, blue; H, white; O, red; and P, yellow.

Figure 2-27
The ATP molecule serves as an energy carrier in cells. The energy-requiring formation of ATP from ADP and inorganic phosphate is coupled to the energy-yielding oxidation of foodstuffs (in animal cells, fungi, and some bacteria) or to the capture of light (more...)
The most fundamental role of nucleotides in the cell, however, is in the storage and retrieval of biological information. Nucleotides serve as building blocks for the construction of nucleic acids—long polymers in which nucleotide subunits are covalently linked by the formation of a phosphodiester bond between the phosphate group attached to the sugar of one nucleotide and a hydroxyl group on the sugar of the next nucleotide. Nucleic acid chains are synthesized from energy-rich nucleoside triphosphates by a condensation reaction that releases inorganic pyrophosphate during phosphodiester bond formation.
There are two main types of nucleic acids, differing in the type of sugar in their sugar-phosphate backbone. Those based on the sugar ribose are known as ribonucleic acids, or RNA, and contain the bases A, G, C, and U. Those based on deoxyribose (in which the hydroxyl at the 2′ position of the ribose carbon ring is replaced by a hydrogen (see Panel 2-6) are known as deoxyribonucleic acids, or DNA, and contain the bases A, G, C, and T (T is chemically similar to the U in RNA, merely adding the methyl group on the pyrimidine ring) (Figure 2-28). RNA usually occurs in cells in the form of a single polynucleotide chain, but DNA is virtually always in the form of a double-stranded molecule—a DNA double helix composed of two polynucleotide chains running antiparallel to each other and held together by hydrogen-bonding between the bases of the two chains.

Figure 2-28
A small part of one chain of a deoxyribonucleic acid (DNA) molecule. Four nucleotides are shown. One of the phosphodiester bonds that links adjacent nucleotide residues is highlighted in yellow, and one of the nucleotides is shaded in gray. Nucleotides (more...)
The linear sequence of nucleotides in a DNA or an RNA encodes the genetic information of the cell. The ability of the bases in different nucleic acid molecules to recognize and pair with each other by hydrogen-bonding (called base-pairing)—G with C, and A with either T or U—underlies all of heredity and evolution, as explained in Chapter 4.
The Chemistry of Cells is Dominated by Macromolecules with Remarkable Properties
On a weight basis, macromolecules are by far the most abundant of the carbon-containing molecules in a living cell (Figure 2-29 and Table 2-4). They are the principal building blocks from which a cell is constructed and also the components that confer the most distinctive properties of living things. The macromolecules in cells are polymers that are constructed simply by covalently linking small organic molecules (called monomers, or subunits) into long chains (Figure 2-30). Yet they have many remarkable properties that could not have been predicted from their simple constituents.

Figure 2-29
Macromolecules are abundant in cells. The approximate composition of a bacterial cell is shown by weight. The composition of an animal cell is similar (see Table 2-4).
Table 2-4
Approximate Chemical Compositions of a Typical Bacterium and a Typical Mammalian Cell.

Figure 2-30
Three families of macromolecules. Each is a polymer formed from small molecules (called monomers, or subunits) linked together by covalent bonds.
Proteins are especially abundant and versatile. They perform thousands of distinct functions in cells. Many proteins serve as enzymes, the catalysts that direct the large number of covalent bond-making and bond-breaking reactions that the cell needs. All of the reactions whereby cells extract energy from food molecules are catalyzed by proteins serving as enzymes, for example, and an enzyme called ribulose bisphosphate carboxylase converts CO2 to sugars in photosynthetic organisms, producing most of the organic matter needed for life on Earth. Other proteins are used to build structural components, such as tubulin, a protein that self-assembles to make the cell's long microtubules—or histones, proteins that compact the DNA in chromosomes. Yet other proteins act as molecular motors to produce force and movement, as in the case of myosin in muscle. Proteins can also have a wide variety of other functions, and we shall examine the molecular basis for many of them later in this book. Here we merely mention some general principles of macromolecular chemistry that make such functions possible.
Although the chemical reactions for adding subunits to each polymer are different in detail for proteins, nucleic acids, and polysaccharides, they share important features. Each polymer grows by the addition of a monomer onto the end of a growing polymer chain in a condensation reaction, in which a molecule of water is lost with each subunit added (see Figure 2-19). The stepwise polymerization of monomers into a long chain is a simple way to manufacture a large, complex molecule, since the subunits are added by the same reaction performed over and over again by the same set of enzymes. In a sense, the process resembles the repetitive operation of a machine in a factory—except in one crucial respect. Apart from some of the polysaccharides, most macromolecules are made from a set of monomers that are slightly different from one another—for example, the 20 different amino acids from which proteins are made. It is critical to life that the polymer chain is not assembled at random from these subunits; instead the subunits are added in a particular order, or sequence. The elaborate mechanisms that allow this to be accomplished by enzymes are described in detail in Chapters 5 and 6.
Noncovalent Bonds Specify Both the Precise Shape of a Macromolecule and its Binding to Other Molecules
Most of the covalent bonds in a macromolecule allow rotation of the atoms they join, so that the polymer chain has great flexibility. In principle, this allows a macromolecule to adopt an almost unlimited number of shapes, or conformations, as the polymer chain writhes and rotates under the influence of random thermal energy. However, the shapes of most biological macromolecules are highly constrained because of the many weak noncovalent bonds that form between different parts of the molecule. If these noncovalent bonds are formed in sufficient numbers, the polymer chain can strongly prefer one particular conformation, determined by the linear sequence of monomers in its chain. Virtually all protein molecules and many of the small RNA molecules found in cells fold tightly into one highly preferred conformation in this way (Figure 2-31).

Figure 2-31
Most proteins and many RNA molecules fold into only one stable conformation. If the noncovalent bonds maintaining this stable conformation are disrupted, the molecule becomes a flexible chain that usually has no biological value.
The four types of noncovalent interactions important in biological molecules have been previously described in this chapter, and they are reviewed in Panel 2-3 (pp. 114–115). Although individually very weak, these interactions not only cooperate to fold biological macromolecules into unique shapes: they can also add up to create a strong attraction between two different molecules when these molecules fit together very closely, like a hand in a glove. This form of molecular interaction provides for great specificity, inasmuch as the multipoint contacts required for strong binding make it possible for a macromolecule to select out—through binding—just one of the many thousands of other types of molecules present inside a cell. Moreover, because the strength of the binding depends on the number of noncovalent bonds that are formed, interactions of almost any affinity are possible—allowing rapid dissociation when necessary.
Binding of this type underlies all biological catalysis, making it possible for proteins to function as enzymes. Noncovalent interactions also allow macromolecules to be used as building blocks for the formation of larger structures. In cells, macromolecules often bind together into large complexes, thereby forming intricate machines with multiple moving parts that perform such complex tasks as DNA replication and protein synthesis (Figure 2-32).

Figure 2-32
Small molecules, proteins, and a ribosome drawn approximately to scale. Ribosomes are a central part of the machinery that the cell uses to make proteins: each ribosome is formed as a complex of about 90 macromolecules (protein and RNA molecules).
Summary
Living organisms are autonomous, self-propagating chemical systems. They are made from a distinctive and restricted set of small carbon-based molecules that are essentially the same for every living species. Each of these molecules is composed of a small set of atoms linked to each other in a precise configuration through covalent bonds. The main categories are sugars, fatty acids, amino acids, and nucleotides. Sugars are a primary source of chemical energy for cells and can be incorporated into polysaccharides for energy storage. Fatty acids are also important for energy storage, but their most critical function is in the formation of cell membranes. Polymers consisting of amino acids constitute the remarkably diverse and versatile macromolecules known as proteins. Nucleotides play a central part in energy transfer. They are also the subunits from which the informational macromolecules, RNA and DNA, are made.
Most of the dry mass of a cell consists of macromolecules that have been produced as linear polymers of amino acids (proteins) or nucleotides (DNA and RNA), covalently linked to each other in an exact order. The protein molecules and many of the RNAs fold into a unique conformation that depends on their sequence of subunits. This folding process creates unique surfaces, and it depends on a large set of weak interactions produced by noncovalent forces between atoms. These forces are of four types: ionic bonds, hydrogen bonds, van der Waals attractions, and an interaction between nonpolar groups caused by their hydrophobic expulsion from water. The same set of weak forces governs the specific binding of other molecules to macromolecules, making possible the myriad associations between biological molecules that produce the structure and the chemistry of a cell.
- Cells Are Made From a Few Types of Atoms
- The Outermost Electrons Determine How Atoms Interact
- Ionic Bonds Form by the Gain and Loss of Electrons
- Covalent Bonds Form by the Sharing of Electrons
- There Are Different Types of Covalent Bonds
- An Atom Often Behaves as if It Has a Fixed Radius
- Water Is the Most Abundant Substance in Cells
- Some Polar Molecules Form Acids and Bases in Water
- Four Types of Noncovalent Interactions Help Bring Molecules Together in Cells
- A Cell Is Formed from Carbon Compounds
- Cells Contain Four Major Families of Small Organic Molecules
- Sugars Provide an Energy Source for Cells and Are the Subunits of Polysaccharides
- Fatty Acids Are Components of Cell Membranes
- Amino Acids Are the Subunits of Proteins
- Nucleotides Are the Subunits of DNA and RNA
- The Chemistry of Cells is Dominated by Macromolecules with Remarkable Properties
- Noncovalent Bonds Specify Both the Precise Shape of a Macromolecule and its Binding to Other Molecules
- Summary
- The Chemical Components of a Cell - Molecular Biology of the CellThe Chemical Components of a Cell - Molecular Biology of the Cell
Your browsing activity is empty.
Activity recording is turned off.
See more...