By agreement with the publisher, this book is accessible by the search feature, but cannot be browsed.
NCBI Bookshelf. A service of the National Library of Medicine, National Institutes of Health.
Gilbert SF. Developmental Biology. 6th edition. Sunderland (MA): Sinauer Associates; 2000.
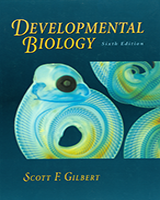
Developmental Biology. 6th edition.
Show detailsCleavage in Sea Urchins
Sea urchins exhibit radial holoblastic cleavage. The first and second cleavages are both meridional and are perpendicular to each other. That is to say, the cleavage furrows pass through the animal and vegetal poles. The third cleavage is equatorial, perpendicular to the first two cleavage planes, and separates the animal and vegetal hemispheres from one another (Figures 8.8 and 8.9). The fourth cleavage, however, is very different from the first three. The four cells of the animal tier divide meridionally into eight blastomeres, each with the same volume. These cells are called mesomeres. The vegetal tier, however, undergoes an unequal equatorial cleavage to produce four large cells, the macromeres, and four smaller micromeres at the vegetal pole (8.10;Summers et al. 1993). As the 16-cell embryo cleaves, the eight mesomeres divide to produce two “animal” tiers, an1 and an2, one staggered above the other. The macromeres divide meridionally, forming a tier of eight cells below an2. The micromeres also divide, albeit somewhat later, producing a small cluster beneath the larger tier. All the cleavage furrows of the sixth division are equatorial, and the seventh division is meridional, producing a 128-cell blastula.
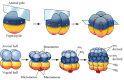
Figure 8.8
Cleavage in the sea urchin. Planes of cleavage in the first three divisions and the formation of tiers of cells in divisions 3–6.
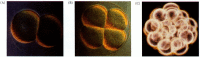
Figure 8.9
Cleavage in the sea urchin. (A-C) Photomicrographs of live embryos of the sea urchin Lytechinus pictus, looking down upon the animal pole. (A) The 2-cell stage. (B) The 4-cell stage. (C) The 32-cell stage, shown without the fertilization membrane to reveal (more...)
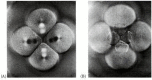
Figure 8.10
Formation of the micromeres during the fourth division of sea urchin embryos. The vegetal poles of the embryos are viewed from below. (A) The location and orientation of the mitotic spindle at the bottom portion of the vegetal cells is shown by viewing (more...)
Blastula formation
The blastula stage of sea urchin development begins at the 128-cell stage. Here the cells form a hollow sphere surrounding a central cavity, or blastocoel (Figure 8.11A). By this time, all the cells are the same size, the micromeres having slowed down their cell division. Every cell is in contact with the proteinaceous fluid of the blastocoel on the inside and with the hyaline layer on the outside. At this time, tight junctions unite the once loosely connected blastomeres into a seamless epithelial sheet that completely encircles the blastocoel (Figure 8.11B; Dan-Sohkawa and Fujisawa 1980). As the cells continue to divide, the blastula remains one cell layer thick, thinning out as it expands. This is accomplished by the adhesion of the blastomeres to the hyaline layer and by an influx of water that expands the blastocoel (Dan 1960; Wolpert and Gustafson 1961; Ettensohn and Ingersoll 1992).
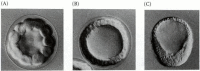
Figure 8.11
Sea urchin blastulae. (A) Formation of a blastocoel as cell division continues. (B) Soon after the rapid divisions of cleavage end, the previously rounded cells unite to form a true epithelium. The fertilization envelope can still be seen. As cilia develop, (more...)
These rapid and invariant cell cleavages last through the ninth or tenth cell division, depending upon the species. After that time, there is a mid-blastula transition, when the synchrony of cell division ends, new genes become expressed, and many of the nondividing cells develop cilia on their outer surfaces; Masuda and Sato 1984). The ciliated blastula begins to rotate within the fertilization envelope. Soon afterward, differences are seen in the cells. The cells at the vegetal pole of the blastula begin to thicken, forming a vegetal plate. The cells of the animal half synthesize and secrete a hatching enzyme that digests the fertilization envelope (Lepage et al. 1992; Figure 8.11C). The embryo is now a free-swimming hatched blastula.
Fate maps and the determination of sea urchin blastomeres
Cell fate determination
The fate map of the sea urchin embryo was originally created by observing each of the cell layers and what its descendants became. More recent investigations have refined these maps by following the fates of individual cells injected with fluorescent dyes such as diI (see Chapter 1). These studies have shown that by the 60-cell stage, most of the embryonic cell fates are specified, but that the cells are not irreversibly committed. In other words, particular blastomeres consistently produce the same cell types in each embryo, but these cells remain pluripotent and can give rise to other cell types if experimentally placed in a different part of the embryo.
A fate map of the 60-cell sea urchin embryo is shown in Figure 8.12 (Logan and McClay 1999; Wray 1999). The animal half of the embryo consistently gives rise to the ectoderm—the larval skin and its neurons. The veg1 layer produces cells that can enter into either the ectodermal or endodermal organs. The veg2 layer gives rise to cells that can populate three different structures—the endoderm, the coelom (body wall), and secondary mesenchyme (pigment cells, immunocytes, and muscle cells). The first tier of micromeres produces the primary mesenchyme cells that form the larval skeleton, while the second tier of micromeres contributes cells to the coelom (Logan and McClay 1997, 1999).
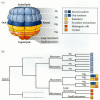
Figure 8.12
Fate map and cell lineage of the sea urchin Strongylocentrotus purpuratus. (A) The 60-cell embryo is shown, with the left side facing the viewer. Blastomere fates are segregated along the animal-vegetal axis of the egg. (B) Cell lineage map of the embryo. (more...)
Although the early blastomeres have consistent fates in the larva, most of these fates are achieved by conditional specification. The only cells whose fates are determined autonomously are the skeletogenic micromeres. If these micromeres are isolated from the embryo and placed in test tubes, they will still form skeletal spicules. Moreover, if these micromeres are transplanted into the animal region of the blastula, not only will their descendants form skeletal spicules, but the transplanted micromeres will alter the fates of nearby cells by inducing a secondary site for gastrulation. Cells that would normally have produced ectodermal skin cells will be respecified as endoderm and will produce a secondary gut (Figure 8.13; Hörstadius 1973; Ransick and Davidson 1993). The micromeres appear to produce a signal that tells the cells adjacent to them to become endoderm and induces them to invaginate into the embryo. Their ability to reorganize the embryonic cells is so pronounced that if the isolated micromeres are recombined with an isolated animal cap (the top two animal tiers), the animal cap cells will generate endoderm, and a more or less normal larva will develop (Figure 8.14; Hörstadius 1939).
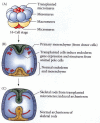
Figure 8.13
Ability of the micromeres to induce a secondary axis in sea urchin embryos. (A) Micromeres are transplanted from the vegetal pole of a 16-cell embryo into the animal pole of a host 16-cell embryo. (B) The transplanted micromeres invaginate into the blastocoel (more...)
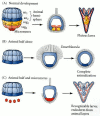
Figure 8.14
Ability of the micromeres to induce presumptive ectodermal cells to acquire other fates. (A) Normal development of the 64-cell sea urchin embryo, showing the fates of the different layers. (B) An isolated animal hemisphere becomes a ciliated ball of ectodermal (more...)
In a normal embryo, the veg2 cells become specified by the micromeres, and they, in turn, help specify the veg1 layer. Without the veg2 layer, the veg1 cells are able to produce endoderm, but the endoderm is not specified as foregut, midgut, or hindgut. Thus, there appears to be a cascade wherein the vegetal pole micromeres induce the cells above them to become the veg2 cells, and the veg2 cells induce the cells above them to assume the veg1 fates.Thus, the micromeres undergo autonomous specification to become skeletogenic mesenchyme, and these micromeres produce the initial signals that specify the other tiers of cells.
The identities of the signaling molecules involved in this process are just now becoming known (Sherwood and McClay 1997). The molecule responsible for specifying the micromeres (and their ability to induce the neighboring cells) appears to be β-catenin. As we saw in Chapter 6, β-catenin is a transcription factor that is often activated by the Wnt pathway, and several pieces of evidence suggest it for this role. First, during normal sea urchin development, β-catenin accumulates in the nuclei of those cells fated to become endoderm and mesoderm (Figure 8.15A). This accumulation is autonomous and can occur even if the micromere precursors are separated from the rest of the embryo. Second, this accumulation appears to be responsible for specifying the vegetal half of the embryo. Treating sea urchin embryos with lithium chloride causes the accumulation of β-catenin in all their cells, and this treatment transforms the presumptive ectoderm into endoderm. Conversely, experimental procedures that inhibit β-catenin accumulation in the vegetal cell nuclei prevent the formation of endoderm and mesoderm (Figure 8.15B,C; Logan et al. 1998; Wikramanayake et al. 1998). It is possible that the levels of nuclear β-catenin accumulation help to determine the mesodermal and endodermal fates of the vegetal cells. Third, β-catenin is essential for giving the micromeres their inductive ability.* Experiments described above demonstrated that the micromeres were able to induce a second embryonic axis when transplanted to the animal hemisphere. However, micromeres from embryos in which β-catenin was prevented from entering the nucleus were unable to induce the animal hemisphere cells to form endoderm, and a second axis was not formed (Logan et al. 1998).
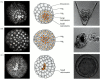
Figure 8.15
The role of β-catenin in specifying the vegetal cells of the sea urchin embryo. β-catenin is stained by a fluorescently labeled antibody. (A) During normal development, β-catenin accumulates predominantly in the micromeres and (more...)
It appears that the specification of cell fates in sea urchins involves a cascade initiated by the micromeres. It has been hypothesized (Davidson 1989) that the signal from the micromeres induces a post-translational modification in some factor in the veg2 layer. Similarly, the signal from the veg2 layer probably modifies some protein (possibly a transcription factor) in the veg1 layer. In this way, the different tiers are assigned different fates.
Axis specification
In the sea urchin blastula, the cell fates line up along the animal-vegetal axis established in the egg cytoplasm prior to fertilization. The animal-vegetal axis also appears to structure the future anterior-posterior axis, with the vegetal region sequestering those maternal components necessary for posterior development (Boveri 1901; Maruyama et al. 1985).
In most sea urchins, the dorsal-ventral and left-right axes are specified after fertilization, but the manner of their specification is not well understood. Since the first cleavage plane can be either parallel, perpendicular, or oblique with respect to the eventual dorsal-ventral axis, it is probable that the dorsal-ventral axis is not specified until the 8-cell stage, when there are cell boundaries that correspond to these positions (Kominami 1983; Henry et al. 1992). Interestingly, in those sea urchins that bypass the larval stage to develop directly into juveniles, the dorsal-ventral axis is specified maternally in the egg cytoplasm (Henry and Raff 1990).
WEBSITE
8.2 Sea urchin cell specification.The specification of sea urchin cells was one of the first major research projects in experimental embryology and remains a fascinating area. This site looks at how new studies amplify some of the earliest investigations into cell differentiation. http://www.devbio.com/chap08/link0802.shtml
Sea Urchin Gastrulation
The late sea urchin blastula consists of a single layer of about 1000 cells that form a hollow ball, somewhat flattened at the vegetal end. The blastomeres, derived from different regions of the zygote, have different sizes and properties. Figures 8.16 and 8.17 show the fates of the various regions of the blastula as it develops through gastrulation to the pluteus larva stage characteristic of sea urchins. The fate of each cell layer can be seen through its movements during gastrulation.
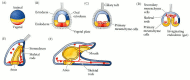
Figure 8.16
Normal sea urchin development, following the fate of the cellular layers of the blastula. (A) Fate map of the zygote. (B) Late blastula with ciliary tuft and flattened vegetal plate. (C) Blastula with primary mesenchyme. (D) Gastrula with secondary mesenchyme. (more...)
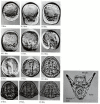
Figure 8.17
Entire sequence of gastrulation in Lytechinus variegatus. The time shows the length of development at 25°C. (Photographs courtesy of J. Morrill; pluteus larva courtesy of G. Watchmaker.)
Ingression of primary mesenchyme
Function of primary mesenchyme cells
Shortly after the blastula hatches from its fertilization envelope, the vegetal side of the spherical blastula begins to thicken and flatten (Figure 8.17, 9 hours). At the center of this flat vegetal plate, a cluster of small cells begins to change. These cells begin extending and contracting long, thin (30 × 5 μm) processes called filopodia from their inner surfaces. The cells then dissociate from the epithelial monolayer and ingress into the blastocoel (Figure 8.17, 9–10 hours). These cells, derived from the micromeres, are called the primary mesenchyme. They will form the larval skeleton, so they are sometimes called the skeletogenic mesenchyme. At first the cells appear to move randomly along the inner blastocoel surface, actively making and breaking filopodial connections to the wall of the blastocoel. Eventually, however, they become localized within the prospective ventrolateral region of the blastocoel. Here they fuse into syncytial cables, which will form the axis of the calcium carbonate spicules of the larval skeleton (Figure 8.18).
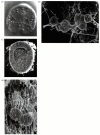
Figure 8.18
Formation of syncytial cables by mesenchyme cells of the sea urchin. (A) Primary mesenchyme cells in the early gastrula align and fuse to lay down the matrix of the calcium carbonate spicule (arrows). (B) Scanning electron micrograph (SEM) of spicules (more...)
Importance of extracellular lamina inside the blastocoel
The ingression of the micromere descendants into the blastocoel is caused by these cells losing their affinity for their neighbors and for the hyaline membrane and acquiring a strong affinity for a group of proteins that line the blastocoel. This model was first proposed by Gustafson and Wolpert (1967) and was confirmed in 1985, when Rachel Fink and David McClay measured the strengths of sea urchin blastomere adhesion to the hyaline layer, to the basal lamina lining the blastocoel, and to other blastomeres. Originally, all the cells of the blastula are connected on their outer surface to the hyaline layer and on their inner surface to a basal lamina secreted by the cells. On their lateral sides, each cell has another cell for a neighbor. Fink and McClay found that the prospective ectoderm and endoderm cells (descendants of the mesomeres and macromeres, respectively) bind tightly to one another and to the hyaline layer, but adhere only loosely to the basal lamina (Table 8.2). The micromeres originally display a similar pattern of binding. However, the micromere pattern changes at gastrulation. Whereas the other cells retain their tight binding to the hyaline layer and to their neighbors, the primary mesenchyme precursors lose their affinity for these structures (which drops to about 2% of its original value) while their affinity for components of the basal lamina and extracellular matrix (such as fibronectin) increases a hundredfold. This change in affinity causes the micromeres to release their attachments to the external hyaline layer and their neighboring cells and, drawn in by the basal lamina, migrate up into the blastocoel (Figures 8.18, 8.19). These changes in affinity have been correlated with changes in cell surface molecules that occur during this time (Wessel and McClay 1985).
Table 8.2
Affinities of mesenchymal and nonmesenchymal cells to cellular and extracellular componentsa.
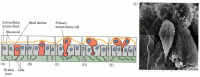
Figure 8.19
Ingression of primary mesenchyme cells. (A-E) Interpretative diagrams depicting changes in adhesive interactions in the presumptive primary mesenchyme cells (color). These cells lose their affinities for hyaline and for their neighboring blastomeres while (more...)
As shown in Figure 8.18, there is a heavy concentration of extracellular material around the ingressing primary mesenchyme cells (Galileo and Morrill 1985; Cherr et al. 1992). Once inside the blastocoel, the primary mesenchyme cells appear to migrate along the extracellular matrix of the blastocoel wall, extending their filopodia in front of them (Galileo and Morrill 1985; Karp and Solursh 1985). Several proteins (including fibronectin and a particular sulfated glycoprotein) are necessary to initiate and maintain this migration (Wessel et al. 1984; Sugiyama 1972; Lane and Solursh 1991; Berg et al. 1996).
But these guidance cues cannot be sufficient, since the migrating cells “know” when to stop their movement and form spicules near the equator of the blastocoel. The primary mesenchyme cells arrange themselves in a ring at a specific position along the animal-vegetal axis. At two sites near the future ventral side of the larva, many of these primary mesenchyme cells cluster together, fuse with each other, and initiate spicule formation (Figure 8.18A; Hodor and Ettensohn 1998). If a labeled micromere from another embryo is injected into the blastocoel of a gastrulating sea urchin embryo, it migrates to the correct location and contributes to the formation of the embryonic spicules (Figure 8.20; Ettensohn 1990). It is thought that this positional information is provided by the prospective ectodermal cells and their basal laminae (von Übisch 1939; Harkey and Whiteley 1980). Only the primary mesenchyme cells (and not other cell types or latex beads) are capable of responding to these patterning cues (Ettensohn and McClay 1986). Miller and colleagues (1995) have reported the existence of extremely fine (0.3-μm diameter) filopodia on the skeleton-forming mesenchyme. These filopodia are not thought to function in locomotion; rather, they appear to explore and sense the blastocoel wall and may be responsible for picking up dorsal-ventral and animal-vegetal patterning cues from the ectoderm (Figure 8.20; Malinda et al. 1995).
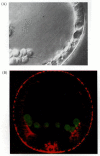
Figure 8.20
Placement of the primary mesenchyme cells. (A) Nomarski videomicrograph showing a long, thin filopodium from a primary mesenchyme cell extending to the ectodermal wall of the gastrula, as well as a shorter filopodium extending inward from the ectoderm. (more...)
First stage of archenteron invagination
As the ring of primary mesenchyme cells leaves the vegetal region of the blastocoel, important changes are occurring in the cells that remain at the vegetal plate. These cells remain bound to one another and to the hyaline layer of the egg, and they move to fill the gaps caused by the ingression of the primary mesenchyme. Moreover, the vegetal plate bends inward and invaginates about one-fourth to one-half the way into the blastocoel (Figure 8.21A; also see Figure 8.17, 10.5–11.5 hours). Then invagination suddenly ceases. The invaginated region is called the archenteron (primitive gut), and the opening of the archenteron at the vegetal region is called the blastopore.
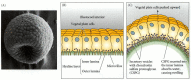
Figure 8.21
Invagination of the vegetal plate. (A) Vegetal plate invagination in Lytechinus variegatus, seen by scanning electron micrography of the external surface of the early gastrula. The blastopore is clearly visible. (B) The hyaline layer consists of inner (more...)
Lane and co-workers (1993) have provided evidence that the mechansim of this invagination is similar to that of the buckling produced by heating a bimetallic strip. The hyaline layer is actually made up of two layers, an outer lamina made primarily of hyalin protein and an inner lamina composed of fibropellin proteins (Hall and Vacquier 1982; Bisgrove et al. 1991). Fibropellins are stored in secretory granules within the oocyte, and are secreted from those granules after cortical granule exocytosis releases the hyalin protein. By the blastula stage, the fibropellins have formed a meshlike network over the embryo surface. At the time of invagination, the vegetal plate cells (and only those cells) secrete a chondroitin sulfate proteoglycan into the inner lamina of the hyaline layer directly beneath them. This hygroscopic (water-absorbing) molecule swells the inner lamina, but not the outer lamina. This causes the vegetal region of the hyaline layer to buckle (Figure 8.21B,C). Slightly later, a second force arising from the movements of epithelial cells adjacent to the vegetal plate may facilitate this invagination by drawing the buckled layer inward (Burke et al. 1991).
At the stage when the skeletogenic mesenchyme cells begin ingressing into the blastocoel, the fates of the vegetal plate cells have already been specified (Figure 8.22; Ruffins and Ettensohn 1996). The endodermal cells adjacent to the micromere-derived mesenchyme become foregut, migrating the farthest distance into the blastocoel. The next layer of endodermal cells becomes midgut, and the last circumferential row to invaginate forms the hindgut and anus.
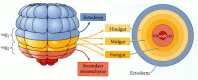
Figure 8.22
Fate map of the vegetal plate of the sea urchin embryo, looking “upward” at the vegetal surface. The central portion becomes the secondary mesenchyme cells, while the concentric layers around it become the foregut, midgut, and hindgut, (more...)
Second and third stages of archenteron invagination
The invagination of the vegetal cells occurs in three discrete stages. After a brief pause, the second phase of archenteron formation begins. During this time, the archenteron extends dramatically, sometimes tripling its length. In this process of extension, the wide, short gut rudiment is transformed into a long, thin tube (see Figure 8.17, 12 hours; Figure 8.23). To accomplish this extension, the cells of the archenteron rearrange themselves by migrating over one another and by flattening themselves (Ettensohn 1985; Hardin and Cheng 1986). This phenomenon, wherein cells intercalate to narrow the tissue and at the same time move it forward, is called convergent extension. Moreover, cell division continues, producing more endodermal and secondary mesenchyme cells as the archenteron extends (Figure 8.24; Martins et al. 1998).
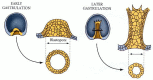
Figure 8.23
Cell rearrangement during the extension of the archenteron in sea urchin embryos. In this species, the early archenteron has 20 to 30 cells around its circumference. Later in gastrulation, the archenteron has a circumference made by only 6 to 8 cells. (more...)
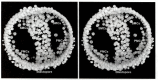
Figure 8.24
Stereo pair showing Lytechinus gastrula during the dispersal of the secondary mesenchyme cells. Convergent extension has occured, and a few mitotic spindles (amall arrows) can be seen. (PMC, primary mesenchyme cell; SMC, secondary mesenchyme cell.) If (more...)
In at least some species of sea urchins, a third stage of archenteron elongation occurs. This last phase is initiated by the tension provided by secondary mesenchyme cells, which form at the tip of the archenteron and remain there (see Figure 8.17, 13 hours; Figure 8.25). Filopodia are extended from these cells through the blastocoel fluid to contact the inner surface of the blastocoel wall (Dan and Okazaki 1956; Schroeder 1981). The filopodia attach to the wall at the junctions between the blastoderm cells and then shorten, pulling up the archenteron. Hardin (1988) ablated the secondary mesenchyme cells with a laser, with the result that the archenteron could elongate to only about two-thirds of the normal length. If a few secondary mesenchyme cells were left, elongation continued, although at a slower rate. The secondary mesenchyme cells, then, play an essential role in pulling the archenteron up to the blastocoel wall during the last phase of invagination.
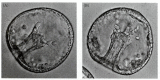
Figure 8.25
Mid-gastrula stage of the sea urchin Lytechinus pictus, showing filopodial extensions of secondary mesenchyme extending from the archenteron tip to the blastocoel wall. (A) Mesenchyme cells extending filopodia from the tip of the archenteron. (B) Filopodial (more...)
But can the secondary mesenchyme filopodia attach to any part of the blastocoel wall, or is there a specific target in the animal hemisphere that must be present for attachment to occur? Is there a region of the blastocoel wall that is already committed to becoming the ventral side of the larva? Studies by Hardin and McClay (1990) show that there is a specific “target” site for the filopodia that differs from other regions of the animal hemisphere. The filopodia extend, touch the blastocoel wall at random sites, and then retract. However, when the filopodia contact a particular region of the wall, they remain attached there, flatten out against this region, and pull the archenteron toward it. When Hardin and McClay poked in the other side of the blastocoel wall so that the contacts were made most readily with that region, the filopodia continued to extend and retract after touching it. Only when the filopodia found their “target” did they cease these movements. If the gastrula was constricted so that filopodia never reached the target area, the secondary mesenchyme cells continued to explore until they eventually moved off the archenteron and found the target tissue as freely migrating cells. There appears, then, to be a target region on what is to become the ventral side of the larva that is recognized by the secondary mesenchyme cells, and which positions the archenteron in the region where the mouth will form.
As the top of the archenteron meets the blastocoel wall in the target region, the secondary mesenchyme cells disperse into the blastocoel, where they proliferate to form the mesodermal organs (see Figure 8.17, 13.5 hours). Where the archenteron contacts the wall, a mouth is eventually formed. The mouth fuses with the archenteron to create a continuous digestive tube. Thus, as is characteristic of deuterostomes, the blastopore marks the position of the anus.
VADE MECUM
Sea urchin development. The CD-ROM provides an excellent review of sea urchin development as well as containing questions on the fundamentals of echinoderm cleavage and gastrulation. [Click on Sea Urchin]
Footnotes
- *
They probably activate the genes necessary for producing the inducing signal, and a Notch ligand (such as Delta) may be one of the signal's components.
- The Early Development of Sea Urchins - Developmental BiologyThe Early Development of Sea Urchins - Developmental Biology
Your browsing activity is empty.
Activity recording is turned off.
See more...