By agreement with the publisher, this book is accessible by the search feature, but cannot be browsed.
NCBI Bookshelf. A service of the National Library of Medicine, National Institutes of Health.
Gilbert SF. Developmental Biology. 6th edition. Sunderland (MA): Sinauer Associates; 2000.
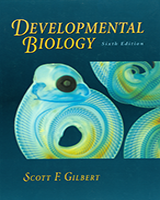
Developmental Biology. 6th edition.
Show detailsThe first known study of comparative developmental anatomy was undertaken by Aristotle in the fourth century b.c.e. He noted the different ways that animals are born: from eggs (oviparity, as in birds, frogs, and most invertebrates), by live birth (viviparity, as in eutherian mammals), or by producing an egg that hatches inside the body (ovoviviparity, as in certain reptiles and sharks). Aristotle also identified the two major cell division patterns by which embryos are formed: the holoblastic pattern of cleavage (in which the entire egg is divided into smaller cells, as it is in frogs and mammals) and the meroblastic pattern of cleavage (as in chicks, wherein only part of the egg is destined to become the embryo, while the other portion—the yolk—serves as nutrition). And should anyone want to know who first figured out the functions of the placenta and the umbilical cord, it was Aristotle.
After Aristotle, there was remarkably little progress in embryology for the next two thousand years. It was only in 1651 that William Harvey concluded that all animals—even mammals—originate from eggs. Ex ovo omnia (“All from the egg”) was the motto on the frontispiece of his On the Generation of Living Creatures, and this precluded the spontaneous generation of animals from mud or excrement. This statement was not made lightly, for Harvey knew that it went against the views of Aristotle, whom Harvey still venerated. (Aristotle had thought that menstrual fluid formed the material of the embryo, while the semen acted to give it form and animation.) Harvey also was the first to see the blastoderm of the chick embryo—that small region of the egg that contains the yolk-free cytoplasm that gives rise to the embryo—and he was the first to notice that “islands” of blood cells form before the heart does. Harvey also suggested that the amnionic fluid might function as a shock absorber for the embryo.
As might be expected, embryology remained little but speculation until the invention of the microscope allowed detailed observations. In 1672, Marcello Malpighi published the first microscopic account of chick development. Here, for the first time, the neural groove (precursor of the neural tube), the muscle-forming somites, and the first circulation of the arteries and veins—to and from the yolk—were identified (Figure 1.2).

Figure 1.2
Depictions of chick developmental anatomy. (A) Dorsal view (looking “down” at what will become the back) of a 2-day chick embryo, as depicted by Marcello Malpighi in 1672. (B) Ventral view (looking “up” at the prospective (more...)
Epigenesis and preformation
With Malpighi begins one of the great debates in embryology—the controversy over whether the organs of the embryo are formed de novo (“from scratch”) at each generation, or whether the organs are already present, but in miniature form, within the egg (or sperm). The first view is called epigenesis, and it was supported by Aristotle and Harvey. The second view is called preformation, and it was reinvigorated with support from Malpighi. Malpighi showed that the unincubated* chick egg already had a great deal of structure. This observation provided him with reasons to question epigenesis. According to the preformationist view, all the organs of the adult were prefigured in miniature within the sperm or (more usually) the egg. Organisms were not seen to be “developed,” but rather “unrolled.”
The preformationist hypothesis had the backing of eighteenth-century science, religion, and philosophy (Gould 1977; Roe 1981, Pinto-Correia 1997). First, because all organs were prefigured, embryonic development merely required the growth of existing structures, not the formation of new ones. No extra mysterious force was needed for embryonic development. Second, just as the adult organism was prefigured in the germ cells, another generation already existed in a prefigured state within the germ cells of the first prefigured generation. This corollary, called embôitment (encapsulation), ensured that the species would always remain constant. Although certain microscopists claimed to see fully formed human miniatures within the sperm or egg, the major proponents of this hypothesis—Albrecht von Haller and Charles Bonnet—knew that organ systems develop at different rates and that embryonic structures need not be in the same place as those in the newborn.
The preformationists had no cell theory to provide a lower limit to the size of their preformed organisms (the cell theory arose in the mid-1800s), nor did they view humankind's tenure on Earth as potentially infinite. Rather, said Bonnet (1764), “Nature works as small as it wishes,” and the human species existed in that finite time between Creation and Resurrection. This view was in accord with the best science of its time, conforming to the French mathematician-philosopher René Descartes's principle of the infinite divisibility of a mechanical nature initiated, but not interfered with, by God. It also conformed to Enlightenment views of the Deity. The scientist-priest Nicolas Malebranche saw in preformationism the fusion of the rule-giving God of Christianity with Cartesian science (Churchill 1991; Pinto-Correia 1997).†
The embryological case for epigenesis was revived at the same time by Kaspar Friedrich Wolff, a German embryologist working in St. Petersburg. By carefully observing the development of chick embryos, Wolff demonstrated that the embryonic parts develop from tissues that have no counterpart in the adult organism. The heart and blood vessels (which, according to preformationism, had to be present from the beginning to ensure embryonic growth) could be seen to develop anew in each embryo. Similarly, the intestinal tube was seen to arise by the folding of an originally flat tissue. This latter observation was explicitly detailed by Wolff, who proclaimed (1767), “When the formation of the intestine in this manner has been duly weighed, almost no doubt can remain, I believe, of the truth of epigenesis.” However, to explain how an organism is created anew each generation, Wolff had to postulate an unknown force, the vis essentialis (“essential force”), which, acting like gravity or magnetism, would organize embryonic development.
A reconciliation of sorts was attempted by the German philosopher Immanuel Kant (1724–1804) and his colleague, biologist Johann Friedrich Blumenbach (1752–1840). Attempting to construct a scientific theory of racial descent, Blumenbach postulated a mechanical, goal-directed force called the Bildungstrieb (“development force”). Such a force, he said, was not theoretical, but could be shown to exist by experimentation. A hydra, when cut, regenerates its amputated parts by rearranging existing elements (see Chapter 18). Some purposive organizing force could be observed in operation, and this force was a property of the organism itself. This Bildungstrieb was thought to be inherited through the germ cells. Thus, development could proceed through a predetermined force inherent in the matter of the embryo (Cassirer 1950; Lenoir 1980). Moreover, this force was believed to be susceptible to change, as demonstrated by the left-handed variant of snail coiling (where left-coiled snails can produce right-coiled progeny). In this hypothesis, wherein epigenetic development is directed by preformed instructions, we are not far from the view held by modern biologists that most of the instructions for forming the organism are already present in the egg.
Naming the parts: The primary germ layers and early organs
The end of preformationism did not come until the 1820s, when a combination of new staining techniques, improved microscopes, and institutional reforms in German universities created a revolution in descriptive embryology. The new techniques enabled microscopists to document the epigenesis of anatomical structures, and the institutional reforms provided audiences for these reports and students to carry on the work of their teachers. Among the most talented of this new group of microscopically inclined investigators were three friends (born within a year of each other) who came from the Baltic region and who studied in northern Germany. The work of Christian Pander, Karl Ernst von Baer, and Heinrich Rathke transformed embryology into a specialized branch of science (and allowed the term embryology to be used to describe their work).
Pander studied the chick embryo for less than two years (before becoming a paleontologist), but in those 15 months, he discovered the three germ layers,‡ the specific regions of the embryo that give rise to the specific organ systems (see Figure 1.1).
- The ectoderm generates the outer layer of the embryo. It produces the surface layer (epidermis) of the skin and forms the nerves.
- The endoderm becomes the innermost layer of the embryo and produces the digestive tube and its associated organs (including the lungs).
- The mesoderm becomes sandwiched between the ectoderm and endoderm. It generates the blood, heart, kidney, gonads, bones, and connective tissues.
These three layers are found in the embryos of all triploblastic (three-layer) organisms. Some phyla, such as the porifera (sponges), cnidarians (sea anemones, hydra, jellyfish), and ctenophores (comb jellies) lack a true mesoderm and are considered diploblastic animals.
Pander (1817) also made observations that weighted the balance in favor of epigenesis. The germ layers, he noted, did not form their organs independently. Rather, each germ layer “is not yet independent enough to indicate what it truly is; it still needs the help of its sister travelers, and therefore, although already designated for different ends, all three influence each other collectively until each has reached an appropriate level.” Pander had discovered the tissue interactions that we now call induction. No tissue is able to construct organs by itself; it must interact with other tissues. (We will discuss the principles of induction more thoroughly in Chapter 6.) Thus, Pander felt that preformation could not be true, since the organs come into being through interactions between simpler structures.
Interestingly, the glory of Pander's book is its engravings; the artist, Eduard d’Alton, drew details for which the vocabulary had not yet been invented. Today we can look at these drawings and see the four regions of the embryonic chick brain, even though these regions had not yet been separately defined or given names (Figure 1.2C; see Churchill 1991). The ability to make precise observations has been among the greatest skills of embryologists, and even today modern developmental biologists looking at gene expression patterns are “rediscovering” regions of the embryo that were observed by embryologists a century ago.
Rathke looked at the development of frogs, salamanders, fish, birds, and mammals, and emphasized the similarities in the development of all these vertebrate groups. During his 40 years of embryological research, he described for the first time the vertebrate pharyngeal arches (Figure 1.3), which become the gill apparatus of fish but become the mammalian jaws and ears, (among other things, as we will see in Figure 1.14), the formation of the vertebrate skull, and the origin of the reproductive, excretory, and respiratory systems. He also studied the development of invertebrates, especially the crayfish. He is memorialized today in the name Rathke's pouch, the embryonic rudiment of the glandular portion of the pituitary. That he could see such a structure using the techniques available at that time is testimony to his remarkable powers of observation and his steady hand.

Figure 1.3
Pharyngeal arches (also called branchial arches and gill arches) in the embryo of the salamander Ambystoma mexicanum. The surface ectoderm has been removed to permit the easy visualization of these arches (highlighted) as they form. (Photograph courtesy (more...)

Figure 1.14
Jaw structure in the fish, reptile, and mammal. (A) Homologies of the jaws and gill arches as seen in the skull of the Paleozoic shark Cobeledus aculentes. (B) Lateral view of an alligator skull. The articular portion of the lower jaw articulates with (more...)
Karl Ernst von Baer extended Pander's studies of the chick embryo. He discovered the notochord, the rod of dorsalmost mesoderm that separates the embryo into right and left halves and which instructs the ectoderm above it to become the nervous system (Figure 1.4). He also discovered the mammalian egg, that long-sought cell that everyone believed existed but no one had yet seen.§

Figure 1.4
The notochord in the chick embryo. (A) Dorsal view of the 24-hour chick embryo. (B) A cross-section through the trunk shows the notochord and developing neural tube. By comparing Figures 1.2 and 1.4, you should see the remarkable changes between days (more...)
The four principles of Karl Ernst von Baer
In 1828, von Baer reported, “I have two small embryos preserved in alcohol, that I forgot to label. At present I am unable to determine the genus to which they belong. They may be lizards, small birds, or even mammals.” Figure 1.5 allows us to appreciate his quandary. All vertebrate embryos (fish, reptiles, amphibians, birds, and mammals) begin with a basically similar structure. From his detailed study of chick development and his comparison of chick embryos with the embryos of other vertebrates, von Baer derived four generalizations (now often referred to as “von Baer's laws”), stated here with some vertebrate examples:

Figure 1.5
The similarities and differences between different vertebrate embryos as they proceed through development. They each begin with a basically similar structure, although they acquire this structure at different ages and sizes. As they develop, they become (more...)
- 1.
The general features of a large group of animals appear earlier in development than do the specialized features of a smaller group. All developing vertebrates appear very similar shortly after gastrulation. It is only later in development that the special features of class, order, and finally species emerge. All vertebrate embryos have gill arches, notochords, spinal cords, and primitive kidneys.
- 2.
Less general characters are developed from the more general, until finally the most specialized appear. All vertebrates initially have the same type of skin. Only later does the skin develop fish scales, reptilian scales, bird feathers, or the hair, claws, and nails of mammals. Similarly, the early development of the limb is essentially the same in all vertebrates. Only later do the differences between legs, wings, and arms become apparent.
- 3.
The embryo of a given species, instead of passing through the adult stages of lower animals, departs more and more from them.¶ The visceral clefts of embryonic birds and mammals do not resemble the gill slits of adult fish in detail. Rather, they resemble the visceral clefts of embryonic fish and other embryonic vertebrates. Whereas fish preserve and elaborate these clefts into true gill slits, mammals convert them into structures such as the eustachian tubes (between the ear and mouth).
- 4.
Therefore, the early embryo of a higher animal is never like a lower animal, but only like its early embryo. Human embryos never pass through a stage equivalent to an adult fish or bird. Rather, human embryos initially share characteristics in common with fish and avian embryos. Later, the mammalian and other embryos diverge, none of them passing through the stages of the others.
Von Baer also recognized that there is a common pattern to all vertebrate development: the three germ layers give rise to different organs, and this derivation of the organs is constant whether the organism is a fish, a frog, or a chick.
WEBSITE
1.1 The reception of von Baer's principles. The acceptance of von Baer's principles and their interpretation over the past hundred years has varied enormously. Recent evidence suggests that one important researcher in the 1800s even fabricated data when his own theory went against these postulates. http://www.devbio.com/chap01/link0101.shtml
Fate mapping the embryo
By the late 1800s, the cell had been conclusively demonstrated to be the basis for anatomy and physiology. Embryologists, too, began to base their field on the cell. One of the most important programs of descriptive embryology became the tracing of cell lineages: following individual cells to see what they become. In many organisms, this fine a resolution is not possible, but one can label groups of cells to see what that area of the embryo will become. By bringing such studies together, one can construct a fate map. These diagrams “map” the larval or adult structure onto the region of the embryo from which it arose. Fate maps are the bases for experimental embryology, since they provide researchers with information on which portions of the embryo normally become which larval or adult structures. Fate maps of some embryos at the early gastrula stage are shown in Figure 1.6. Fate maps have been generated in several ways.

Figure 1.6
Fate maps of different vertebrate classes at the early gastrula stage. All views are dorsal surface views (looking “down” on the embryo at what will be its back). Despite the different appearances of these adult animals, their fate maps (more...)
Observing living embryos
In certain invertebrates, the embryos are transparent, have relatively few cells, and the daughter cells remain close to one another. In such cases, it is actually possible to look through the microscope and trace the descendants of a particular cell into the organs they generate. This type of study was performed about a century ago by Edwin G. Conklin. In one of these studies, he took eggs of the tunicate Styela partita, a sea squirt that resides in the waters off the coast of Massachusetts, and he patiently followed the fates of each cell in the embryo until they differentiated into particular structures (Figure 1.7; Conklin 1905). He was helped in this endeavor by the peculiarity of the Styela egg, wherein the different cells contain different pigments. For example, the muscle-forming cells always had a yellow color. Conklin's fate map was confirmed by cell removal experiments. Removal of the B4.1 cell (which should produce all the tail musculature), for example, resulted in the absence of tail muscles (Reverberi and Minganti 1946).

Figure 1.7
Fate map of the tunicate embryo. (A) The 1-cell embryo (left), shown shortly before the first cell division, with the fate of the cytoplasmic regions indicated. The 8-cell embryo on the right shows these regions after three cell divisions. (B) A linear (more...)
WEBSITE
1.2 Conklin's art and science. The plates from Conklin's remarkable 1905 paper are online. Looking at them, one can see the precision of his observations and how he constructed his fate map of the tunicate embryo. http://www.devbio.com/chap01/link0102.shtml
VADE MECUM
The compound microscope. The compound microscope has been the critical tool of developmental anatomists. Mastery of microscopic techniques allows one to enter an entire world of form and pattern. [Click on Microscope]
Vital dye marking
Most embryos are not so accommodating as to have cells of different colors. Nor do all embryos have as few cells as tunicates. In the early years of the twentieth century, Vogt (1929) traced the fates of different areas of amphibian eggs by applying vital dyes to the region of interest. Vital dyes will stain cells but not kill them. He mixed the dye with agar and spread the agar on a microscope slide to dry. The ends of the dyed agar would be very thin. He cut chips from these ends and placed them onto a frog embryo. After the dye stained the cells, the agar chip was removed, and cell movements within the embryo could be followed (Figure 1.8).

Figure 1.8
Vital dye staining of amphibian embryos. (A) Vogt's method for marking specific cells of the embryonic surface with vital dyes. (B–D) Dorsal surface views of stain on successively later embryos. (E) Newt embryo dissected in a medial sagittal section (more...)
Radioactive labeling and fluorescent dyes
A variant of the dye marking technique is to make one area of the embryo radioactive. To do this, a donor embryo is usually grown in a solution containing radioactive thymidine. This base will be incorporated into the DNA of the dividing embryo. A second embryo (the host embryo) is grown under normal conditions. The region of interest is cut out from the host embryo and replaced by a radioactive graft from the donor embryo. After some time, the host embryo is sectioned for microscopy. The cells that are radioactive will be the descendants of the cells of the graft, and can be distinguished by autoradiography. Fixed microscope slides containing the sectioned tissues are dipped into photographic emulsion. The high-energy electrons from the radioactive thymidine will reduce the silver ions in the emulsion (just as light would). The result is a cluster of dark silver grains directly above the radioactive region. In this manner, the fates of different regions of the chick embryo have been determined (Rosenquist 1966).
One of the problems with vital dyes and radioactive labels is that they become diluted at each cell division. One way around this problem was the creation of fluorescent dyes that were extremely powerful and could be injected into individual cells. Fluorescein-conjugated dextran, for example, could be injected into a single cell of an early embryo. The descendants of that cell could then be seen by examining the embryo under ultraviolet light (Figure 1.9). More recently, diI, a powerfully fluorescent molecule that becomes incorporated into lipid membranes, has also been used to follow the fates of cells and their progeny.

Figure 1.9
Fate mapping using a fluorescent dye. (A) Specific cells of a zebrafish embryo were injected with a fluorescent dye that will not diffuse from the cells. The dye was then activated by laser in a small region (about five cells) of the late cleavage stage (more...)
Genetic marking
The problems with radioactive and vital dye marking include their dilution over many cell divisions and the laborious preparation of the slides. One permanent way of marking cells is to create mosaic embryos having different genetic constitutions. One of the best examples of this technique is the construction of chimeric embryos, consisting, for example, of a graft of quail cells inside a chick embryo. Chick and quail develop in a very similar manner (especially during early embryonic development), and a graft of quail cells will become integrated into a chick embryo and participate in the construction of the various organs. The substitution of quail cells for chick cells can be performed on an embryo while it is still inside the egg, and the chick that hatches will have quail cells in particular sites, depending upon where the graft was placed. The quail cells differ from the chick's in two important ways. First, the quail heterochromatin in the nucleus is concentrated around the nucleoli, making the quail nucleus easily distinguishable from chick nuclei. Second, there are cell-specific antigens that are quail-specific and can be used to find individual quail cells, even if they are in a large population of chick cells. In this way, fine-structure maps of the chick brain and skeletal system can be made (Figure 1.10; Le Douarin 1969; Le Douarin and Teillet 1973).

Figure 1.10
Genetic markers as cell lineage tracers. (A) Grafting experiment wherein the cells from a particular region of a 1-day quail embryo have been placed into a similar region of a 1-day chick embryo. (B) After several days, the quail cells can be seen by (more...)
VADE MECUM
Histotechniques. Most cells must be stained in order to see them; different dyes stain different types of molecules. Instructions on staining cells to observe particular structures (such as the nucleus) are given here. [Click on Histotechniques]
Cell migration
One of the most important contributions of fate maps has been their demonstration of extensive cell migration during development. Mary Rawles (1940) showed that the pigment cells (melanocytes) of the chick originate in the neural crest, a transient band of cells that joins the neural tube to the epidermis. When she transplanted small regions of neural crest-containing tissue from a pigmented strain of chickens into a similar position in an embryo from an unpigmented strain of chickens, the migrating pigment cells entered the epidermis and later entered the feathers (Figure 1.11A). Ris (1941) used similar techniques to show that while almost all of the external pigment of the chick embryo came from the migrating neural crest cells, the pigment of the retina formed in the retina itself and was not dependent on the migrating neural crest cells. By using radioactive marking techniques, Weston (1963) demonstrated that the migrating neural crest cells gave rise to the melanocytes, and also to the peripheral neurons and the epinephrine-secreting adrenal medulla (Figure 1.11B, C). This pattern was confirmed in chick-quail hybrids, in which the quail neural crest cells produced their own pigment and pattern in the chick feathers. More recently, fluorescent dye labeling has followed the movements of individual neural crest cells as they form their pigment, adrenal, and neuronal lineages (see Chapter 13).

Figure 1.11
Neural crest cell migration. (A) Chick resulting from the transplantation of a trunk neural crest region from an embryo of a pigmented strain of chickens into the same region of an embryo of an unpigmented strain. The neural crest cells that gave rise (more...)
In addition to the travels of pigment cells, other wide-scale migrations include those of the primordial germ cells (which migrate from the yolky cells to the gonads and form the sperm and eggs) and the blood cell precursors (which undergo several migrations to colonize the liver and bone marrow).
Footnotes
- *
As was pointed out by Maître-Jan in 1722, the egg examined by Malpighi may technically be called “unincubated,” but as it was left sitting in the Bolognese sun in August, it was not unheated.
- †
Preformation was a conservative theory, emphasizing the lack of change between generations. Its principal failure was its inability to account for the variations known by the limited genetic evidence of the time. It was known, for instance, that matings between white and black parents produced children of intermediate skin color, an impossibility if inheritance and development were solely through either the sperm or the egg. In more controlled experiments, the German botanist Joseph Kölreuter (1766) had produced hybrid tobacco plants having the characteristics of both species. Moreover, by mating the hybrid to either the male or female parent, Kölreuter was able to “revert” the hybrid back to one or the other parental type after several generations. Thus, inheritance seemed to arise from a mixture of parental components.
- ‡
From the same root as germination: the Latin germen, meaning “sprout” or “bud.” The names of the three germ layers are from the Greek: ectoderm from ektos (“outside”) plus derma (skin); mesoderm from mesos (“middle”), and endoderm from endon (“within”).
- §
von Baer could hardly believe that he had at last found it when so many others—Harvey, de Graaf, von Haller, Prevost, Dumas, and even Purkinje—had failed. “I recoiled as if struck by lightening … I had to try to relax a while before I could work up enough courage to look again, as I was afraid I had been deluded by a phantom. Is it not strange that a sight which is expected, and indeed hoped for, should be frightening when it eventually materializes?”
- ¶
von Baer formulated these generalizations prior to Darwin's theory of evolution. “Lower animals” would be those appearing earlier in life's history.
- Comparative Embryology - Developmental BiologyComparative Embryology - Developmental Biology
Your browsing activity is empty.
Activity recording is turned off.
See more...