By agreement with the publisher, this book is accessible by the search feature, but cannot be browsed.
NCBI Bookshelf. A service of the National Library of Medicine, National Institutes of Health.
Gilbert SF. Developmental Biology. 6th edition. Sunderland (MA): Sinauer Associates; 2000.
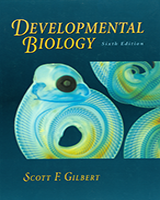
Developmental Biology. 6th edition.
Show detailsRegeneration—the reactivation of development in later life to restore missing tissues—is so “unhuman” that it has been a source of fascination to humans since the beginnings of biological science. It is difficult to behold the phenomenon of limb regeneration in newts or starfish without wondering why we cannot grow back our own arms and legs. What gives salamanders this ability we so sorely lack? Experimental biology was born in the efforts of eighteenth-century naturalists to document regeneration and to answer this question. The regeneration experiments of Tremblay (hydras), Réaumur (crustaceans), and Spallanzani (salamanders) set the standard for experimental research and for the intelligent discussion of one's data (see Dinsmore 1991). Réaumur, for instance, noted that crayfish had the ability to regenerate their limbs because their limbs broke easily at the joints. Human limbs, he wrote, were not so vulnerable, so Nature provided us not with regenerable limbs, but with “a beautiful opportunity to admire her foresight.” Tremblay's advice to researchers who would enter this new field is pertinent to read even today. He tells us to go directly to nature and to avoid the prejudices that our education has given us.* Moreover, “one should not become disheartened by want of success, but should try anew whatever has failed. It is even good to repeat successful experiments a number of times. All that is possible to see is not discovered, and often cannot be discovered, the first time.”
More than two centuries later, we are beginning to find answers to the great problem of regeneration, and we may soon be able to alter the human body so as to permit our own limbs, nerves, and organs to regenerate. This would mean that severed limbs could be restored, that diseased organs could be removed and regrown, and that nerve cells altered by age, disease, or trauma could once again function normally. To bring these benefits to humanity, we first have to understand how regeneration occurs in those species that have this ability. Our new knowledge of the roles of paracrine factors in organ formation, and our ability to clone the genes that produce those factors, has propelled what Susan Bryant (1999) has called “a regeneration renaissance.” Since “renaissance” literally means “rebirth,” and since regeneration can be seen as a return to the embryonic state, the term is apt in many ways.
There are three major ways by which regeneration can occur. The first mechanism involves the dedifferentiation of adult structures to form an undifferentiated mass of cells that then becomes respecified. This type of regeneration is called epimorphosis and is characteristic of regenerating limbs. The second mechanism is called morphallaxis. Here, regeneration occurs through the repatterning of existing tissues, and there is little new growth. Such regeneration is seen in hydras. A third type of regeneration is an intermediate type, and can be thought of as compensatory regeneration. Here, the cells divide, but maintain their differentiated functions. They produce cells similar to themselves and do not form a mass of undifferentiated tissue. This type of regeneration is characteristic of the mammalian liver. We discussed regeneration of flatworms (Chapter 3) and of the amphibian eye (Chapter 4) earlier in the book. Here we will concentrate on salamander limb, hydras, and mammalian liver regeneration.
Epimorphic Regeneration of Salamander Limbs
When an adult salamander limb is amputated, the remaining cells are able to reconstruct a complete limb, with all its differentiated cells arranged in the proper order. In other words, the new cells construct only the missing structures and no more. For example, when a wrist is amputated, the salamander forms a new wrist and not a new elbow (Figure 18.25). In some way, the salamander limb “knows” where the proximal-distal axis has been severed and is able to regenerate from that point on.

Figure 18.25
Regeneration of a salamander forelimb. The amputation shown on the left was made below the elbow; the amputation shown on the right cut through the humerus. In both cases, the correct positional information is respecified. (From Goss 1969; photograph (more...)
Formation of the apical ectodermal cap and regeneration blastema
Salamanders accomplish this feat by dedifferentiation and respecification. Upon limb amputation, a plasma clot forms, and within 6 to 12 hours, epidermal cells from the remaining stump migrate to cover the wound surface, forming the wound epidermis. This single-layered structure is required for the regeneration of the limb, and it proliferates to form the apical ectodermal cap. Thus, in contrast to wound healing in mammals, no scar forms, and the dermis does not move with the epidermis to cover the site of amputation. The nerves innervating the limb degenerate for a short distance proximal to the plane of amputation (see Chernoff and Stocum 1995). During the next 4 days, the cells beneath the developing cap undergo a dramatic dedifferentiation: bone cells, cartilage cells, fibroblasts, myocytes, and neural cells lose their differentiated characteristics and become detached from one another. Genes that are expressed in differentiated tissues (such as the MRF4 and myf5 genes expressed in the muscle cells) are downregulated, while there is a dramatic increase in the expression of genes, such as msx1, that are associated with the proliferating progress zone mesenchyme of the embryonic limb (Simon et al. 1995). The formerly well-structured limb region at the cut edge of the stump thus forms a proliferating mass of indistinguishable, dedifferentiated cells just beneath the apical ectodermal cap. This dedifferentiated cell mass is called the regeneration blastema. These cells will continue to proliferate, and will eventually redifferentiate to form the new structures of the limb (Figure 18.26; Butler 1935).

Figure 18.26
Regeneration in the larval forelimb of the spotted salamander Ambystoma maculatum. (A) Longitudinal section of the upper arm, 2 days after amputation. The skin and muscle have retracted from the tip of the humerus. (B) At 5 days after amputation, a thin (more...)
The creation of the blastema depends upon the formation of single, mononucleated cells. It is probable that the macrophages that are released into the wound site secrete metalloproteinases that digest the extracellular matrices holding epithelial cells together (Yang et al. 1999). But many of these cells are differentiated and have left the cell cycle. How do they regain the ability to divide? Microscopy and tracer dye studies have shown that when multinucleated myotubes (whose nuclei are removed from the cell cycle: see Chapter 14) are introduced into a blastema, they give rise to labeled mononucleated cells that proliferate and can differentiate into many tissues of the regenerated limb (Hay 1959; Lo et al. 1993). It appears that myotube nuclei are forced to enter the cell cycle by a serum factor created by thrombin, the same protease that is involved in forming clots. Thrombin is released when the amputation is made, and when serum is exposed to thrombin, it forms a factor capable of inducing cultured newt myotubes to enter the cell cycle. Mouse myotubes, however, do not respond to this chemical. This difference in responsiveness may relate directly to the difference in regenerative ability between salamanders and mammals† (Tanaka et al. 1999).
Proliferation of the blastema cells: the requirement for nerves
The proliferation of the salamander limb regeneration blastema is dependent on the presence of nerves. Singer (1954) demonstrated that a minimum number of nerve fibers must be present for regeneration to take place. It is thought that the neurons release mitosis-stimulating factors that increase the proliferation of the blastema cells (Singer and Caston 1972; Mescher and Tassava 1975). There are several candidates for these neural-derived mitotic factors, and each may be important. Glial growth factor (GGF) is known to be produced by newt neural cells, is present in the blastema, and is lost upon denervation. When this peptide is added to a denervated blastema, the mitotically arrested cells are able to divide again (Brockes and Kinter 1986). A fibroblast growth factor may also be involved. FGFs infused into denervated blastemas are able to restore mitosis (Mescher and Gospodarowicz 1979). Another important neural agent necessary for cell cycling is transferrin, an iron-transport protein that is necessary for mitosis in all dividing cells (since ribonucleotide reductase, the rate-limiting enzyme of DNA synthesis, requires a ferric ion in its active site). When a hindlimb is severed, the sciatic nerve transports transferrin along the axon and releases large quantities of this protein into the blastema (Munaim and Mescher 1986; Mescher 1992). Neural extracts and transferrin are both able to stimulate cell division in denervated limbs, and chelation of ferric ions from a neural extract abolishes its mitotic activity (Munaim and Mescher 1986; Albert and Boilly 1988).
Pattern formation in the regeneration blastema
The regeneration blastema resembles in many ways the progress zone of the developing limb. The dorsal-ventral and anterior-posterior axes between the stump and the regenerating tissue are conserved, and cellular and molecular studies have confirmed that the patterning mechanisms of developing and regenerating limbs are very similar. By transplanting regenerating limb blastemas onto developing limb buds, Muneoka and Bryant (1982) showed that the blastema cells could respond to limb bud signals and contribute to the developing limb. At the molecular level, just as Sonic hedgehog is seen in the posterior region of the developing limb progress zone mesenchyme, it is seen in the early posterior regeneration blastema (Imokawa and Yoshizato 1997; Torok et al. 1999). The initial pattern of Hox gene expression in regenerating limbs is not the same as that in developing limbs. However, the nested pattern of Hoxa and Hoxd gene expression characteristic of limb development is established as the limb regenerates (Torok et al. 1998).
Retinoic acid appears to play an important role both in the dedifferentiation of the cells to form the regeneration blastema and in the respecification processes as the cells redifferentiate. If regenerating animals are treated with sufficient concentrations of retinoic acid (or other retinoids), their regenerated limbs will have duplications along the proximal-distal axis (Figure 18.27; Niazi and Saxena 1978; Maden 1982). This response is dose-dependent and at maximal dosage can result in a complete new limb regenerating (starting at the most proximal bone), regardless of the original level of amputation. Dosages higher than this result in inhibition of regeneration. It appears that the retinoic acid causes the cells to be respecified to a more proximal position (Figure 18.28; Crawford and Stocum 1988b; Pecorino et al. 1996).

Figure 18.27
Effects of vitamin A (a retinoid) on regenerating salamander limbs. (A) Normal regenerated axolotl limb (9×) with humerus, paired radius and ulna, carpals, and digits. Dotted line shows plane of amputation through the carpal area. (B) Regeneration (more...)

Figure 18.28
Proximalization of blastema respecification by retinoic acid. (A) When a wrist blastema from a recently cut axolotl forelimb is placed onto a host hindlimb cut at the mid-thigh level, it will generate only the wrist. The host (whose own leg was removed) (more...)
Retinoic acid is synthesized in the regenerating limb wound epidermis and is seen to form a gradient along the proximal-distal axis of the blastema (Brockes 1992; Scadding and Maden 1994; Viviano et al. 1995). This gradient of retinoic acid is thought to activate genes differentially across the blastema, resulting in the specification of pattern in the regenerating limb. One of these retinoic acid-responsive genes is the msx1 gene that is associated with mesenchyme proliferation (Shen et al. 1994). Another set of genes that may be respecified by retinoic acid is the Hoxa genes. Gardiner and colleagues (1995) have shown that the expression pattern of certain Hoxa genes in the distal cells of the regeneration blastema is changed by exogenous retinoic acid into an expression pattern characteristic of more proximal cells. It is probable that during normal regeneration, the wound epidermis/apical ectodermal cap secretes retinoic acid, which activates the genes needed for cell proliferation, downregulates the genes that are specific for differentiated cells, and activates a set of Hox genes that tells the cells where they are in the limb and how much they need to grow. The mechanism by which the Hox genes do this is not known, but changes in cell-cell adhesion and other surface qualities of the cells have been observed (Nardi and Stocum 1983; Stocum and Crawford 1987; Bryant and Gardiner 1992). Thus, in salamander limb regeneration, adult cells can go “back to the future,” returning to an “embryonic” condition to begin the formation of the limb anew.
WEBSITE
18.5 The polar coordinate model. The phenomena of epimorphic regeneration can be seen formally as events that reestablish continuity among tissues that the amputation has severed. The polar coordinate model attempts to explain the numerous phenomena of limb regeneration. http://www.devbio.com/chap18/link1805.shtml
WEBSITE
18.6 Regeneration in annelid worms. An easy laboratory exercise can discover the rules by which worms regenerate their segments. This website details some of those experiments. http://www.devbio.com/chap18/link1806.shtml
Compensatory Regeneration in the Mammalian Liver
The liver's ability to regenerate seems to have been known since ancient times. According to Greek mythology, Prometheus's punishment (for giving civilization to humans) was to be chained to a rock and to have an eagle eat a portion of his liver each day. His liver recovered from this partial hepatectomy each night, thereby continually supplying food for the eagle and eternal punishment for Prometheus. The standard assay for liver regeneration is to remove (after anesthesia) specific lobes of the liver, leaving the others intact. The removed lobe does not grow back, but the remaining lobes enlarge to compensate for the loss of the missing liver tissue (Higgins and Anderson 1931). Even in humans, the amount of liver regenerated is equivalent to the amount of liver removed.
The liver regenerates by the proliferation of the existing tissues. Surprisingly, the regenerating liver cells do not fully dedifferentiate when they reenter the cell cycle. No blastema is formed. Rather, the five types of liver cells—hepatocytes, duct cells, fat-storing (Ito) cells, endothelial cells, and Kupffer macrophages—each begin dividing to produce more of themselves (Figure 18.29). Each type of cell retains its cellular identity, and the liver retains its ability to synthesize the liver-specific enzymes necessary for glucose regulation, toxin degradation, bile synthesis, albumin production, and other hepatic functions (Michalopoulos and DeFrances 1997).

Figure 18.29
Kinetics of DNA synthesis in the four major cell types of the mammalian liver. It is possible that, since the hepatocytes respond fastest, they are secreting paracrine factors that induce DNA replication in the other cells. (After Michalopoulos and DeFrances (more...)
As in the regenerating salamander limb, there is a return to an embryonic condition in the regenerating liver. Fetal transcription factors and products are made, as are the cyclins that control cell division. But the return to the embryonic state is not as complete as in the amphibian limb. Although other paracrine and endocrine factors are necessary for liver regeneration, one of the most important proteins for returning liver cells to the cell cycle is hepatocyte growth factor (HGF). This protein, also known and mentioned earlier in the book as scatter factor, induces many of the embryonic proteins. Within an hour after partial hepatectomy, the blood level of HGF has risen 20-fold (Lindroos et al. 1991). However, hepatocytes that are still connected to one another in an epithelium cannot respond to HGF. The trauma of partial hepatectomy may activate metalloproteinases that digest the extracellular matrix and permit the hepatocytes to separate and proliferate. These enzymes also may cleave HGF to its active form (Mars et al. 1995). The mechanisms by which the factors interact and by which the liver is told to stop regenerating after the appropriate size is reached remain to be discovered.
Morphallactic Regeneration in Hydras
Hydra‡ is a genus of freshwater cnidarians. Most hydras are about 0.5 cm long. A hydra has a tubular body, with a “head” at its distal end and a “foot” at its proximal end. The “foot,” or basal disc, enables the hydra to stick to rocks. The “head” consists of a conical hypostome region (containing the mouth) surrounded by a ring of tentacles (which catch its food). Hydras have only two epithelial cell layers, lacking a true mesoderm. They can reproduce sexually, but do so only under adverse conditions, such as severe crowding. They usually multiply by budding off a new individual (Martin 1997; Figure 18.30). The buds form about two-thirds of the way down the body axis.

Figure 18.30
Budding in Hydra. A new individual buds from the right side of an adult Hydra. (Photograph © Biophoto/Photo Researchers Inc.)
When a hydra is cut in half, the half containing the head will regenerate a new basal disc, and the half containing the basal disc will regenerate a new head. Moreover, if a hydra is cut into several portions, the middle portions will regenerate both heads and basal discs at their appropriate ends. No cell division is required for this to happen, and the result is a small hydra. This regeneration is morphallactic.
The head activator gradient
The above experiments show that every portion of the hydra along the apical-basal axis is potentially able to form a basal disc, a head, or even an entire hydra. However, the polarity of the hydra is coordinated by a series of morphogenetic gradients that permit the head to form only at one place and the disc to form only at another. Evidence for such gradients in hydras was first obtained by grafting experiments begun by Ethel Browne in the early 1900s. When hypostome tissue from one hydra is transplanted into the middle of another hydra, it forms a new apical-basal axis, with the hypostome extending outward (Figure 18.31A). When a basal disc is grafted to the middle of a host hydra, a new axis also forms, but with the opposite polarity, extending a basal disc (Figure 18.31B). When cells from both ends are transplanted simultaneously into the middle of a host, no new axis is formed, or the new axis has little polarity (Figure 18.31C; Browne 1909; Newman 1974). These experiments have been interpreted to indicate the existence of a head activator gradient (highest at the hypostome) and a basal activator gradient (highest at the basal disc).

Figure 18.31
Grafting experiments showing different morphogenetic capabilities in different regions of the Hydra axis. (A) Hypostome tissue grafted into host trunk induces a secondary axis with an extended hypostome. (B) Basal disc tissue grafted into host trunk induces (more...)
The head activator gradient can be measured by implanting rings of tissue from various levels of a donor hydra into a particular region of the host trunk (MacWilliams 1983b). The higher the level of head activator in the donor tissue, the greater the percentage of implants that will induce the formation of new hypostomes. The head activating factor is found to be concentrated in the head and to decrease linearly toward the basal disc.
The head inhibitor gradient
In 1926, Rand showed that the normal regeneration of the hypostome is inhibited when an intact hypostome is grafted adjacent to the amputation site. This finding suggested that one hypostome can inhibit the formation of another. Extra heads do not form in the hydra because the presence of the hypostome prevents the formation of any other hypostome. The head inhibitor gradient can be measured by inserting a subhypostomal region (a region just below the hypostome, having a relatively high concentration of head activator) into various regions along the trunks of host hydras. This region will not produce a head when implanted into the apical area of an intact host hydra (Figure 18.32). However, it will form a head if the host's head has been removed. Moreover, this subhypostomal region will induce a head if placed lower on the host. Thus, there appears to be a gradient of head inhibitor as well as head activator (Wilby and Webster 1970; MacWilliams 1983a).

Figure 18.32
Grafting experiments providing evidence for a gradient of head inhibitor. (A) Subhypostomal tissue does not generate a new head when placed close to an existing head. (B) Subhypostomal tissue forms a head if the existing one is removed. A head will also (more...)
Basal disc activator and inhibitor gradients
The basal disc also has properties suggesting that it is the source of a foot inhibitor gradient and a foot activator gradient (Hicklin and Wolpert 1973; Schmidt and Schaller 1976; Meinhardt 1993; Grens et al. 1999). The inhibitor gradients for the head and the foot may be important for determining where and when a bud can form. In young adult hydras, the gradients of head and foot inhibitors appear to block bud formation. However, as the hydra grows, the sources of these labile substances grow farther apart, creating a region of tissue, about two-thirds down the trunk, where both inhibitor gradients are minimal. Here is where the bud forms (Figure 18.33; Shostak 1974; Bode and Bode 1984; Schiliro et al. 1999). Certain mutants of Hydra have defects in their ability to form buds, and these defects can be explained by alterations of the morphogen gradients. The L4 mutant of Hydra magnipapillata, for instance, forms buds very slowly, and only after reaching a size about twice as long as wild-type individuals. The amount of head inhibitory substance in these mutants was found to be much greater than in wild-type Hydra (Takano and Sugiyama 1983).

Figure 18.33
Head inhibitor (blue line) and foot inhibitor (red line) gradients in newly dropped hydra buds, young adults, and budding adults. (After Bode and Bode 1984.)
The inhibitor and activator gradients also inform the hydra “which end is up” and specify positional values along the apical-basal axis. When the head is removed, the head inhibitor no longer is made, and this causes the head activator to induce a new head. The region with the most head activator will form the head. Once the head is made, it makes the head inhibitor, and the equilibrium is restored.
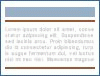
Box
Medical Advances in Regeneration.
Footnotes
- *
The tradition of leaving one's books and classrooms and going directly to nature is very strong in developmental biology. There is a sign at Woods Hole Marine Biological Laboratory, the scene of some of the most important embryological research in America. The sign, attributed to Louis Agassiz, reads, “Study Nature, Not Books.” It hangs at the entrance to the library.
- †
This thrombin-produced factor has not yet been isolated. But it is not the only difference between urodele and mammalian limbs. Another difference is that salamanders retain Hox gene expression in their appendages even when they are adults. It should be noted that, although most of the regeneration blastema comes from the dedifferentiation of the tissue at the edge of the stump, another source of cells is also possible. While multinucleated skeletal muscle tissue will dedifferentiate and supply uncommitted mononucleated cells to the blastema, the limb also contains muscle satellite cells—mononucleated cells committed to the muscle lineage—that may be used during regeneration. Thus, the regenerated limb's muscles may come from both the blastema and from these reserve cells.
- ‡
The Hydra is another character from Greek mythology. Whenever one of this serpent's many heads was chopped off, it regenerated two new ones. Hercules finally defeated the Hydra by cauterizing the stumps of its heads with fire. Hercules had a longstanding interest in regeneration, for he was also the hero who finally freed the bound Prometheus, thus stopping his daily hepatectomies.
- Regeneration - Developmental BiologyRegeneration - Developmental Biology
Your browsing activity is empty.
Activity recording is turned off.
See more...