Except where otherwise noted, this work is licensed under a Creative Commons Attribution-NonCommercial-NoDerivatives 4.0 International License (CC-BY-NC-ND 4.0). To view a copy of this license, visit http://creativecommons.org/licenses/by-nc-nd/4.0/)
NCBI Bookshelf. A service of the National Library of Medicine, National Institutes of Health.
Ferretti JJ, Stevens DL, Fischetti VA, editors. Streptococcus pyogenes: Basic Biology to Clinical Manifestations [Internet]. 2nd edition. Oklahoma City (OK): University of Oklahoma Health Sciences Center; 2022 Oct 8.

Streptococcus pyogenes: Basic Biology to Clinical Manifestations [Internet]. 2nd edition.
Show detailsAbstract
Control of virulence gene expression in S. pyogenes is under the charge of 13 two-component regulatory systems and at least 30 transcriptional regulators known thus far. These virulence-related regulators are tasked with integrating environmental host cues with the pathogen’s own metabolic state, as well as feedback signals from the expressed genome, into a coordinated response. This overview of the current understanding of S. pyogenes virulence-related regulators describes their roles in one or multiple of the following categories: Master regulators, such as CovR/S, RocA, LiaS/F/R, and RofA-like proteins (RALPs), that control the activity of multiple virulence-related regulators; Metabolite-responsive regulators, which sense and respond to changes in availability of sources of energy; carbohydrates (CcpA, Mga), amino acid/nitrogen supplies (Rsh, CodY), and other metabolites S. pyogenes must monitor in the host; Metabolic-control regulators, such as VicR/S, MtsR, CiaH/R, that influence expression of metabolism-related genes to maintain the necessary homeostasis to promote colonization; and Environmental/host immunity-responsive regulators, which control responses to a variety of cues, like oxidative stress (PerR, CiaH/R), quorum sensing (Rgg2/3, Sil), saliva (SalK/R, SptR/S), blood, and neutrophils (Ihk/Irr, CovR/S, LiaS/F/R). Emphasis is made on the interdependence among regulators and the ways in which variation in their connectivity depends on their genotypic background, likely due to coevolution with its human host.
Introduction
Under natural conditions, Streptococcus pyogenes (group A streptococci) is a strictly human-specific pathogen, implying that this fastidious species (i) lacks an independent environmental reservoir, and (ii) in order to succeed as a pathogen, needs to spread before extinguishing its host. S. pyogenes has demonstrated a high capacity to colonize multiple tissue sites and cause a wide variety of different diseases. These range from mild superficial infections of the skin (such as impetigo) and mucosal membranes (such as pharyngitis) to severe invasive infections (such as streptococcal toxic-shock-like syndrome or necrotizing fasciitis) to autoimmune sequelae (such as rheumatic fever). Infection of mammals other than humans requires experimental conditions that are characterized by unnaturally high bacterial titers and specific genotypes that are capable of breaking species barriers. Ideally, on the scale of population biology, the pathogen and its specific host should coevolve and exhibit an evolutionary equilibrium, with coevolution rates balancing each other (Rosenzweig, Brown, & Vincent, 1987), as suggested by the “Red Queen” argument (“It takes all the running you can do, to keep in the same place” (Van Valen, 1973)). In mammalian streptococcal pathogens, the well-explored example of host plasminogen activation mediated by streptokinase illustrates the workings of coevolution and renders streptokinase responsible for the host’s specificity of infection (Gladysheva, Turner, Sazonova, Liu, & Reed, 2003; Sun, et al., 2004). The ample and varied repertoire of virulence factors that S. pyogenes expresses in the course of infection (as detailed elsewhere) and their highly coordinated regulation results from the coevolution described by the Red Queen principle. Control of virulence factor expression in S. pyogenes is under the charge of 13 two-component regulatory systems and at least 30 transcriptional regulators known thus far (Kreikemeyer, McIver, & Podbielski, 2003). These virulence-related regulators are tasked with integrating environmental cues (such as nutrient availability, chemical stressors, host immune components, and temperature) with information on the pathogen’s own metabolic state, as well as feedback signals from the expressed genome, into a coordinated response. As a result, the virulence-related regulators of S. pyogenes constitute a highly interconnected network that is in constant flux, which makes it quite difficult to elucidate and represent. Most of what is known about virulence factor regulation in S. pyogenes has stemmed from the examination of the pathogen’s responses to different environmental and internal inputs. Moreover, emerging literature reveals that some of these regulators are capable of translating metabolic inputs unrelated to disease (like carbohydrate levels) into changes in the expression of factors for the evasion of host immunity. As a result, we have organized this overview of the current understanding of S. pyogenes virulence-related regulators by describing their roles in one, multiple or each (in some cases) of the following categories: metabolite sensing, metabolism control, coordination among regulators, and environmental/host immunity responsiveness (Figure 1). An emphasis is made on the interdependence among regulators and the ways in which variation in their connectivity depends on their serotypic/ genotypic background, most likely as a result from coevolution with their primary host niches. Several regulators will be described in more than one of these categories, given their activity, especially in the case of those involved in coordinating other regulators, which are referred to here as “master” regulators.
Mechanisms of regulation
In terms of mechanisms of action, the virulence-related regulators of S. pyogenes have been found to belong to three types: two-component signal transduction systems (TCSs) (Table 1, 2); so-called “stand alone” transcriptional activators/repressors; and non-coding RNAs (Table 3, 4). TCSs are a common mechanism among bacteria to integrate the detection of extracellular signals with intracellular adaptive responses. These consist of a transmembrane sensor histidine kinase component, which recognizes an extracellular signal; and an associated cytoplasmic response regulator, which transduces the input sensed by the kinase into activation/repression of virulence-related gene expression. Upon interaction with the signaling stimulus, two sensor kinase molecules often form a dimer, and are activated and auto-phosphorylated at one or both conserved histidine residues in the cytoplasmic transducer domain. The phosphorylated transducer signals to the cytoplasmic response regulator by transferring its phosphoryl group to an aspartyl residue of the regulator, which in turn acts as a transcription factor to control target gene expression. The genome sequences of S. pyogenes serotypes M1, M3 and M18 (Ferretti, et al., 2001; Beres, et al., 2002; Smoot, et al., 2002) have revealed the presence of an average of 13 TCSs, of which 11 are shared in serotypes M1 and M18 (Beres, et al., 2002). All systems have been annotated and assigned a putative function on the basis of sequence homology towards similar systems from other species (Graham, et al., 2002) or defined functions, as will be discussed later.
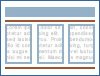
Table 2.
Activity of TCS regulators of virulence in S. pyogenes. The categories of downstream effectors influenced by TCS regulator activity in S. pyogenes (colored fields) are shown. Colors correspond to categories across all tables.
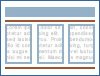
Table 4.
Activity of transcriptional regulators of virulence in S. pyogenes. The categories of downstream effectors influenced by transcriptional regulator activity (colored fields) are shown. Colors correspond to categories across all tables.
In contrast to TCSs, stand-alone regulators activate and/or repress the transcription of virulence genes without inputs from cognate TCS sensor kinases. This is not to say that all virulence regulators of this kind function without inputs from other cell signaling components; rather, that associated sensory kinases or other sensory elements have not been identified for them. The importance of stand-alone regulator contributions to S. pyogenes biology and virulence is underscored by their ubiquity. An in silico analysis of the molecular epidemiology of 35 stand-alone response regulators found that 97% of the 944 queried S. pyogenes genomes encoded 32 or more of the 35 examined response regulators (Buckley, Davies, & McMillan, 2020). Buckley et al determined strong associations between concatenated response regulator allele types, and M serotype, MLST-type, core genome phylogroup and location of strain sampling, leading them to propose that 11 response regulators may serve to refine S. pyogenes typing systems to better reflect core genome evolutionary associations (Buckley, Davies, & McMillan, 2020). Additionally, the analysis revealed the level to which certain regulators are highly conserved, while others show allelic diversity resulting from recombination events (e.g., mga, rofA/nra, msmR), though no substantial associations between individual loci and disease potential were uncovered. The best-characterized stand-alone virulence regulators are Mga and the RofA-like protein type regulators (RALPs); we will use existing knowledge of these regulators to illustrate what is understood about this type of virulence regulator. For the most part, these regulators have been identified as containing DNA-binding domains that can interact directly with sequences in the promoter regions of the genes they control, but for many specific binding sequences, either they have not been characterized or they are hypothesized to indirectly affect the expression of their targets (Kreikemeyer, McIver, & Podbielski, 2003).
Alternatively, small non-coding RNAs of S. pyogenes, like in other bacteria, regulate gene expression primarily at the translational level in response to environmental signals (Wassarman, Zhang, & Storz, 1999). Most non-coding RNAs participate in posttranscriptional regulation by base pairing with target mRNAs, which results in either inhibition/activation of translation or degradation of the mRNAs (Gottesman, 2004). A small group of non-coding RNAs function by interacting with RNA-binding proteins to modify their activities (Majdalani, Vanderpool, & Gottesman, 2005). Only a relatively small number of non-coding RNAs have been identified and characterized in S. pyogenes so far, as compared to those observed in other bacteria (Tesorero, et al., 2013).
Master regulators/Virulence regulator control
In the first category, we examine the role that some regulators play in the control of other virulence-related regulators, which causes them to be referred to as master regulators. This is the case for TCSs like CovR/S, as well as RofA and other RALPs.
CovR/S
The best-characterized TCS of S. pyogenes is control of virulence (CovR/S) or capsule synthesis regulator (CsrR/S). CovS senses external signals, including environmental Mg2+ and host antimicrobial peptides (Gryllos, Levin, & Wessels, 2003; Gryllos, et al., 2008a) and transduces them to CovR, which in turn regulates the expression of several virulence gene regulons (Table 2). Phosphorylation of CovR enhances oligomerization of the activated protein and subsequent DNA binding to long AT-rich sequences within the target promoter regions (Miller, Engleberg, & DiRita, 2001; Gusa, Gao, Stringer, Churchward, & Scott, 2006). CovR mechanisms of binding to DNA appear to vary across promoters and display a high affinity for single DNA binding sites (Churchward, Bates, Gusa, Stringer, & Scott, 2009) or cooperative binding along long stretches of promoter region DNA (Gao, Gusa, Scott, & Churchward, 2005). A consensus CovR-binding element (ATTARA) has been proposed on the basis of binding studies of the hasA promoter (Federle & Scott, 2002), but no single conserved binding box has been identified, even among the 18 most strongly CovR influenced genes (Graham, et al., 2002). For a thorough review of the molecular mechanisms of CovR transcriptional regulation, see (Churchward, 2007).
More recent research has further defined the molecular mechanisms that underlie CovRS-mediated changes in S. pyogenes global gene expression. CovS acts as both a kinase and phosphatase to modulate levels of CovR phosphorylation. Increased streptolysin O (Slo) and NADase (Nga/Spn) production from S. pyogenes exposure to subinhibitory concentrations of clindamycin was found to involve CovRS activity (Chiang-Ni, Tseng, Shi, & Chiu, 2019). Inactivation of CovS phosphatase activity, whether by mutation or growth medium supplementation with Mg2+, inhibited clindamycin-mediated upregulation of Slo production in a dose-dependent manner. Horstmann et al. established a key role for CovS phosphatase activity in S. pyogenes pathogenesis using iso-allelic M1 and M3 S. pyogenes strains that differed in mutations that abrogated either CovS kinase or phosphatase activity, as well as CovR phosphorylation, to define their respective contribution to S. pyogenes pathogenesis (Horstmann, et al., 2018). In both serotypes, mutants that lack CovS phosphatase activity (CovST284A) and that failed to respond to signaling by human cathelicidin (LL-37) were impaired in causing skin infection or colonizing the oropharynx in murine models and showed reduced survival in human blood. Impairment of CovS phosphatase activity also decreased transcription of multiple virulence genes, including emm and its regulator Mga (Horstmann, et al., 2018). Additionally, hypervirulent S. pyogenes generated by spontaneous CovRS-inactivating mutations emerged at a lower frequency in CovST284A strains than in the iso-allelic parents.
Alternately, CovS kinase activity is involved in repression of CovR-regulated genes (hasA, ska, slo) under acidic stress, as inactivation of CovS kinase activity or CovR protein phosphorylation derepresses transcription of these genes in an acidic environment (Chiang-Ni, Tseng, Hung, & Chiu, 2017). Under acidic conditions, activity of the CovRS promoter (Pcov) increased, and replacement of pPcov with a constitutively active promoter abrogated acid stress-mediated repression of CovR-regulated genes. A CovR phosphorylation site mutant strain (CovRD53E), generated in an M3 background, displayed CovR-dependent differential expression of only a subset of the CovR regulon (Horstmann, Sahasrabhojane, Yao, Su, & Shelburne, 2017). The mutant CovRD53E protein binds promoter DNA from both CovRD53E-regulated and -nonregulated genes, which suggests that more than the ability to bind DNA is involved in CovR regulon modulation. Promoter sequences belonging to CovRD53E-regulated and CovRD53E-nonregulated genes exhibit distinct promoter structures, differing in the location and number of putative CovR-binding sites. Furthermore, the CovRD53E protein cannot dimerize, a functional feature of OmpR/PhoB family regulators. Thus, differences in the CovR regulon associated with the CovRD53E mutation suggest that dimerization-dependent and dimerization-independent modes of CovR-mediated gene repression exist (Horstmann, Sahasrabhojane, Yao, Su, & Shelburne, 2017). Examination of CovRS activation in response to external signals using strains mutagenized in CovS kinase and phosphatase activity reveals that extracellular Mg+2 and the human antimicrobial peptide LL-37 have opposing effects on the phosphorylation of CovR (Finn, Ramsey, Dove, & Wessels, 2021). These mutants had similar but more pronounced effects on CovR phosphorylation relative to growth in magnesium or LL-37 and are correlated with repression or activation of CovR-regulated genes.
CovR/S TCS is considered to be a master regulator, given that microarray and quantitative RT–PCR-based analysis of S. pyogenes serotype M1 revealed that CovR influences the transcription of up to 15% (271 genes) of all chromosomal genes (Graham, et al., 2002), with its activity exerted during late exponential and stationary growth phases. CovR primarily acts as a transcriptional repressor, as it was observed that in a CovR mutant array analysis, only 26 and 4 of the 271 differentially expressed transcripts examined were down regulated during exponential and stationary phase growth, respectively (Graham, et al., 2002; Federle, McIver, & Scott, 1999). CovR both directly and indirectly regulates multiple virulence factors that repress the hyaluronic acid capsule operon (hasABC), and modulate the expression of streptolysin S (SagA), a cysteine protease (SpeB), an NAD glycohydrolase (Nga), streptolysin O (Slo), an IL-8 degrading protease (SpyCEP), streptokinase (Ska), streptodornase (Sda), an immunoglobulin modifying protein (EndoS), and a fibronectin binding protein (Fba) (Graham, et al., 2002; Heath, DiRita, Barg, & Engleberg, 1999; Darmstadt, Mentele, Podbielski, & Rubens, 2000; Levin & Wessels, 1998; Bernish & van de Rijn, 1999; Shelburne, et al., 2010). As a master regulator, CovR/S also controls the expression of other proven and putative transcriptional regulators, as observed in the up-regulation of 8 transcriptional regulators in the exponential growth phase, and that of 18 transcriptional regulators in the stationary phase of growth in a CovR-deficient mutant (Graham, et al., 2002). Chromatin immunoprecipitation and DNA sequencing (ChIPseq) has identified CovR binding sites at CovR/S-regulated promoters, as well as lower-affinity association at additional locations on the S. pyogenes chromosome (Finn, Ramsey, Dove, & Wessels, 2021). Intermediate transcriptional regulators were identified as directly affected by CovR that occupied a subset (22 out of 42) of CovR-regulated promoters with which no CovR was found to be associated by ChIPseq analysis (Finn, Ramsey, Dove, & Wessels, 2021).
One directly CovR-repressed regulator is RivR (Ralp4), a member of the RofA-like protein type (RALP) family of standalone virulence-related regulators that repress the expression of capsule (hasABC) and protein G-related α2-macroglobulin-binding proteins (Grab) involved in the inactivation of proteolytic blood plasma proteins (Roberts, Churchward, & Scott, 2007; Treviño, Liu, Cao, Ramirez-Peña, & Sumby, 2013). Significantly, CovR can regulate its target genes independently of CovS, as S. pyogenes strains with isogenic CovR or CovS mutations have different phenotypes (Treviño, et al., 2009) and the most studied invasive clinical isolate, the MGAS5005 (M1) strain, encodes a truncated and functionally inactive CovS protein (Treviño, et al., 2009; Sumby, Whitney, Graviss, DeLeo, & Musser, 2006; Shelburne, et al., 2008). The regulatory role of CovR/S in responding to the host environment and in metabolic control affecting virulence, whether directly or through the modulation of other virulence-related regulators (such as RALPs, Ihk/Irr, and LytR), is detailed in later sections.
RocA
The “Regulator of Cov” (RocA) was first identified by transposon mutagenesis of an M6 serotype strain, which isolated two independent insertions in the rocA open reading frame that resulted in enhanced CovR expression (Biswas & Scott, 2003). Disruption of rocA resulted in S. pyogenes mucoid colony appearance and increased transcription from the has promoter, both of which are CovR-related phenotypes. Biswas et al. also determined that while RocA negatively autoregulated its expression independently of CovR, RocA-associated phenotypes were dependent on the presence of a wild-type covR allele (Biswas & Scott, 2003).
The interaction of rocA with CovR/S has been validated in multiple S. pyogenes backgrounds by various experimental approaches. Levels of phosphorylated CovR increase in the presence of intact functional RocA, and RocA has no regulatory activity in the context of covR or covS mutation in an M3 S. pyogenes background (Miller, et al., 2015). Conversely, using isogenic mutant M89 S. pyogenes strains, deleting one or more nucleotide repeat units of a variable number tandem repeat upstream of the rocA gene abolished RocA production, reduced CovR phosphorylation, and altered levels of CovR-regulated transcripts (nga, slo, prtS, grab) (Zhu, et al., 2016). Reduction of RocA levels enhanced secretion of Spn NADase and streptolysin O (Slo) and enhanced virulence in a mouse model of necrotizing fasciitis. Further evidence of RocA and CovRS association was provided by the identification of RocA mutants isolated from S. pyogenes-infected mice exhibiting enhanced Sse expression, concomitant with normal levels of SpeB production (Feng, et al., 2016). These variants showed transcript levels of CovRS-controlled virulence genes comparable to those of a covS mutant, but had no covRS mutations.
These lines of evidence and sequence similarity with sensor protein kinases suggested that RocA acts in concert with CovRS phosphorylation to alter gene expression and influence virulence. Ectopic expression of a truncated RocA derivative, harboring the predicted membrane-spanning domains but not the dimerization or HATPase domain, was sufficient to complement an M1 serotype ∆rocA mutant strain (Jain, Miller, Danger, Pflughoeft, & Sumby, 2017). Complementation mutagenesis in an M3 background using intact M1 rocA, and naturally occurring truncated M3 and M18 rocA alleles indicated RocA is a pseudokinase that inhibits capsule expression and reduced M1 S. pyogenes resistance to killing in human blood (Jain, Miller, Danger, Pflughoeft, & Sumby, 2017). Varying the RocA concentration attenuates the regulatory activity of Mg2+ and the antimicrobial peptide LL-37, which positively and negatively regulate CovS function, respectively, which further suggests that RocA is an accessory protein to the CovRS system. Co-immunoprecipitation of CovS with tagged RocA transmembrane domain fragments demonstrated RocA interacts with CovS via its seven N-terminal transmembrane domains (Lynskey, Velarde, Finn, Dove, & Wessels, 2019). The effect of expressed RocA fragments on CovR phosphorylation in a rocA-null M18 S. pyogenes strain demonstrated that interaction of RocA with CovS is essential for RocA-mediated regulation of CovRS function. Lynskey et al. also showed that RocA forms homodimers via its cytoplasmic domain, and that RocA truncation observed in M3 isolates alters RocA homotypic interaction, resulting in protein aggregation and impairment of RocA-mediated regulation. Further research determined RocA is a membrane protein that directly interacts with itself (RocA) and CovS, but not CovR (Bernard, Duarte, Bogdanov, Musser, & Olsen, 2020). The seven transmembrane helices of RocA are oriented such that the C-terminus is cytoplasmic, whereas the N terminus is extracellular. Bernard et al. also showed that single amino acid polymorphisms along the entire length of RocA in an M28 strain background can disrupt homotypic and heterotypic (i.e. RocA-CovS) interactions (Bernard, Duarte, Bogdanov, Musser, & Olsen, 2020). This resulted in significant changes in S. pyogenes virulence phenotypes, including in vitro activity of secreted virulence factors (Spn, Slo, Ska), murine model tissue destruction and morbidity. These findings were confirmed in M1 and M3 S. pyogenes, with the exception that in these backgrounds the RocA regulon was found to include the secreted SpeB protease (Jain, Danger, Burgess, Uppal, & Sumby, 2020). Additional studies on the mechanism of RocA regulation determined that while CovR phosphorylation decreased in a rocA mutant during exponential growth, levels of phosphorylated of CovR increased during the stationary phase of growth and under acidic stress in a rocA mutant to levels similar to those in the wild-type strain (Chiang-Ni, Chiou, Tseng, Hsu, & Chiu, 2020). Similar CovR phosphorylation in a CovS phosphatase-inactivated mutant and a rocA mutant under acidic stress and exposure to Mg2+ suggest that CovS phosphatase activity is required for RocA to modulate CovR phosphorylation.
As is the case with other S. pyogenes virulence regulators, RocA heterogeneity correlates with variations in disease potential among and within S. pyogenes serotypes. Unlike M1 S. pyogenes that encode an intact RocA allele (Jain, Miller, Danger, Pflughoeft, & Sumby, 2017), naturally occurring truncation mutations of RocA conserved among serotype M18 (Lynskey, et al., 2013) and M3 serotype S. pyogenes (Lynskey, Turner, Heng, & Sriskandan, 2015; Miller, Pflughoeft, & Sumby, 2015) result in the increased production of hyaluronic acid capsule. In addition to capsule production, the premature stop codon in rocA, due to a single nucleotide polymorphism early in the open reading frame (T269A) in the M18 background enhances covR transcription as well as alters expression of genes involved in metabolism and virulence (spyCEP, cppA, salB) (Lynskey, et al., 2013). Allelic replacement with an intact rocA (from serotype M89) reverted the capsule production phenotype, reduced M18 S. pyogenes survival in human blood, and altered nasopharyngeal carriage longevity in a mouse model of infection. In contrast, the conserved truncated RocA allele in serotype M3 invasive disease isolates is missing 35 amino acids from its C-terminus. RocA truncation in the M3 background results in enhanced expression of several immunomodulatory virulence factor genes (nga/spn, slo, scpC, ska, sse, grab, spd), enhanced hemolysis and NAD(+) hydrolysis, as well as increased resistance to human phagocytic killing and virulence in a murine bacteremia model of infection (Miller, et al., 2015).
M1 RocA mutants exhibit intermediate skin invasion and virulence phenotypes relative to wild-type and CovS mutants in subcutaneous infection of mice (Feng, et al., 2016). RocA and CovS mutations enhanced hasA transcript levels more than those of spyCEP above wild-type M1 S. pyogenes levels, but mutation of RocA, unlike mutations of CovS, did not result in the downregulation of speB transcription at a stationary growth phase or in subcutaneous infection of mice. Additionally, an inverse correlation has been observed between the virulence of wild-type in M1 S. pyogenes, rocA mutant, covS mutant and covR mutant strains during invasive infection and their fitness in an ex vivo upper respiratory tract model (Jain, Danger, Burgess, Uppal, & Sumby, 2020).
M28 S. pyogenes strains collected from invasive infections in humans also exhibit missense and nonsense polymorphisms in rocA (Bernard, Kachroo et al., 2018). As in other serotypes, rocA-inactivating mutations enhanced M28 S. pyogenes virulence in a mouse model of necrotizing myositis. Genome-wide transcript analysis in M28 strains revealed thatRocA inactivation significantly alters transcript levels of 427 genes and 323 genes at mid-exponential and early stationary growth phases, respectively, including 21 virulence factors. Comparison to RocA transcriptomes in other S. pyogenes serotype backgrounds found them to differ in both size and the number of regulators affected (Bernard, et al., 2018). Further investigation of M28 rocA polymorphisms revealed each polymorphism had a unique effect on the global S. pyogenes transcriptome, including differential expression of virulence genes (nga, ifs, slo, spyCEP, sag operon, emm, enn, mrp, scpA, has operon), regulators (mga, spxA2) and metabolic genes (Bernard, et al., 2019). The varied rocA polymorphisms also distinctly altered production of Spn andSlo, as well as Ska and Sof activity. These variations in virulence factor production in turn were associated with an increase or decrease in virulence in mouse and nonhuman primate infection models. Conversely, unlike observations in M1, M3, and M18 S. pyogenes strains, rocA mutation reduced, rather than enhanced, survival of the M12 isolate in human blood ex vivo (Sarkar, et al., 2018).
LiaFSR/Spx
LiaFSR (formerly referred to as YvqFEC in S. pyogenes) is a three-component system (TCS), unique to Gram-positive bacteria, that consists of a predicted histidine kinase sensor (LiaS/YvqE), a response regulator (LiaR/YvqC), and an accessory membrane protein (LiaF/YvqF). In Firmicutes, the LiaFSR system is activated by exposure to antimicrobial peptides (AMPs) and antibiotics (e.g., bacitracin, daptomycin) that target the bacterial membrane, and is therefore critical to the cell envelope stress response (Mascher, 2006). Mutation of LiaFSR proteins or downstream effectors has been associated with changes in antimicrobial susceptibility in enterococci (Arias, et al., 2011; Khan, et al., 2019). The contribution of LiaFSR to S. pyogenes virulence was first described by the discovery of an arginine-to-glycine amino acid mutation in the LiaS histidine kinase (LiaSR135G) that contributed to pharyngeal asymptomatic carriage of an M3 strain (Flores, Jewell, Yelamanchili, Olsen, & Musser, 2015). Mutagenesis of wild-type liaS to liaSR135G and vice-versa altered adherence of M3 S. pyogenes to epithelial cells, S. pyogenes carriage in a mouse nasopharyngeal infection model, and invasiveness in a murine intramuscular infection model.
Recent research suggests that LiaFSR function connects the sensing of host antimicrobial peptide-mediated stress on the bacterial membrane with regulation of S. pyogenes virulence. Antimicrobial peptides (AMPs), which are produced in human skin and mucosal surfaces as well as immune cells, disrupt bacterial membranes through various mechanism of action to achieve killing and clearance of invading bacteria (Melo, Ferre, & Castanho, 2009; Cole & Nizet, Bacterial Evasion of Host Antimicrobial Peptide Defenses, 2016). One target of AMP activity are discrete bacterial membrane locations that carry out crucial cellular processes, which are referred to as functional membrane microdomains (FMM) (López & Kolter, 2010; García-Fernández, et al., 2017). In S. pyogenes, the “ExPortal” is a FMM that colocalizes protein secretion via the general secretory pathway and processing of virulence proteins (Rosch & Caparon, 2004), along with peptidoglycan precursor synthesis proteins (Vega, Port, & Caparon, 2013), in an anionic lipid-enriched microdomain. Polymyxin B and the alpha-defensin human neutrophil peptide 1 (hNP-1) produced in azurophilic granules of neutrophils (Ganz, et al., 1985) preferentially target and disrupt the ExPortal, which inhibits protein secretion and processing (Rosch & Caparon, 2005; Vega & Caparon, 2012). LiaF and LiaS have been found to colocalize to the ExPortal, which when disrupted following exposure to hNP-1, induces activation the LiaFSR system (Lin, Sanson et al., 2020). LiaS colocalization with the S. pyogenes ExPortal can also be disrupted by the elimination of membrane cardiolipin through deletion of cardiolipin synthase (cls), which leaves LiaF membrane localization unaffected. Conversely, production of LiaF appears to be required for maintaining ExPortal integrity (Lin, et al., 2020). Further characterization of LiaFSR-mediated gene regulation through RNAseq analysis of isogenic ∆liaF, ΔliaS and ΔliaR mutants in an M3 S. pyogenes background revealed a near perfect inverse correlation between ∆liaF and ∆liaR transcriptomes, suggesting LiaF acts as an inhibitor of LiaR-mediated transcriptional regulation (Sanson, Vega et al., 2021). The most highly differentially expressed gene in ∆liaF and ∆liaR mutants is that encoding the RNA polymerase-binding protein SpxA2, which has been shown to be directly regulated by activation of LiaR (Sanson, et al., 2021).
Spx regulatory proteins are highly conserved among Firmicutes, acting as anti-activators of expression via their interactions with RNA polymerase (Rojas-Tapias & Helmann, 2019). S. pyogenes encodes two highly similar SpxA paralogs (SpxA1 and SpxA2). An M14 serotype S. pyogenes mutant that lacks SpxA1 grows poorly under aerobic conditions, whereas an SpxA2-lacking strain is severely attenuated in its response to stressors, which can include thermal and oxidative stress (Port, Cusumano, Tumminello, & Caparon, 2017). Loss of SpxA1 reduced susceptibility to the cationic antimicrobial polymyxin B, whereas the absence of SpxA2 enhanced it. Virulence phenotypes in a murine model of soft tissue infection were also in direct opposition, as the ∆spxA1 strain showed highly attenuated virulence, while ∆spxA2 was hypervirulent and produced more extensive tissue damage and a greater bacterial burden than the wild-type isogenic parent (Port, Cusumano, Tumminello, & Caparon, 2017). SpxA1 loss correlated with reduced expression of several toxins (e.g., SpeB), in opposition to speB overexpression observed in the SpxA2-lacking background in which hypervirulence was reverted by deletion of speB.
Among the other genes differentially expressed as a result of LiaFSR mutagenesis were multiple virulence factors that are also regulated by CovRS, and high levels of spxA2 transcript resulting from LiaFSR activation correlate with increased CovR-regulated gene transcription (Sanson, et al., 2021). Moreover, changes in CovR phosphorylation inversely correlate with levels of SpxA2 expression that result from LiaFSR activity, which suggests that regulatory cross talk occurs between LiaFSR and CovRS systems.
Virulence phenotypes that result from LiaFSR mutagenesis suggest additional levels of regulatory complexity associated with the system, since a ΔliaF mutant exhibited decreased virulence in whole human blood and in a murine model of infection, despite the increased levels of CovR-regulated virulence gene expression associated with SpxA2 overexpression (Sanson, et al., 2021). Furthermore, higher than expected virulence was observed for a ∆liaR mutant, which was attributed to elevated SpxA2-associated speB transcription, which as mentioned previously is a phenotype observed in SpxA2 mutants in other S. pyogenes serotypes (Port, Cusumano, Tumminello, & Caparon, 2017). Other studies suggest LiaS function contributes to S. pyogenes biofilm formation (Isaka, et al., 2016) and environmental stress adaptation, including salt, acidic and oxidative stress (Roobthaisong, Aikawa, Nozawa, Maruyama, & Nakagawa, 2017), which potentially result from the substantial effect of LiaFSR activation on SpxA2 expression (Lin, et al., 2020; Sanson, et al., 2021) that in turn has been shown to contribute to S. pyogenes stress responses (Port, Cusumano, Tumminello, & Caparon, 2017). There is also evidence that LiaS mediates biofilm production and pilus expression in an environment acidified through glucose fermentation, as a ∆liaS isogenic mutant produced biofilms in acidified culture but not exposed to glucose (Isaka, et al., 2021).
RofA and the RofA-like protein type regulators (RALPs)
Four RALPs have been identified in S. pyogenes: RofA, Nra, Ralp3, and RivR (Ralp4). Among sequenced strains, they are 52% similar and 29% identical to each other on average (Granok, Parsonage, Ross, & Caparon, 2000), and are involved in the control of S. pyogenes–host-cell interactions, avoidance of host cell damage, and balanced virulence factor expression during stationary phase growth (Beckert, Kreikemeyer, & Podbielski, 2001; Podbielski, Woischnik, Leonard, & Schmidt, 1999; Molinari, et al., 2001). The most studied of the RALPs is the namesake of this family: RofA, a DNA-binding protein that was first identified in a serotype M6 strain as a transcriptional inducer under anaerobic growth conditions of prtF, the gene encoding fibronectin-binding protein F (sfbI) (Fogg & Caparon, 1997). RofA transcription occurs adjacent to and divergently from prtF and is positively autoregulated by RofA interaction with specific 17-bp sites within the intergenic region shared by the two genes (Granok, Parsonage, Ross, & Caparon, 2000; Fogg & Caparon, 1997). RofA directly modulates multiple virulence genes, up-regulating transcription of the FCT region genes (SfbI, the collagen binding protein Cpa, and T antigen) (Kreikemeyer, Beckert, Braun-Kiewnick, & Podbielski, 2002) and repressing transcription of the streptolysin S hemolysin (encoded by sagA), the SpeB protease, and the SpeA superantigen (Beckert, Kreikemeyer, & Podbielski, 2001). As a regulator of other virulence-related regulators, RofA down-regulates Mga in serotype M6 and thus can modulate Mga regulon virulence genes.
Nra is another well-studied RALP, which is only present in M serotypes that carry the FCT type 3 gene region (M3, 18 and 49), as all other M serotypes possess FCT gene regions that encode for RofA instead of Nra (Kratovac, Manoharan, Luo, Liziano, & Bessen, 2007). In the M49 strain, Nra was characterized as a repressor for the adjacent cpa, as well as for an unlinked gene that encodes a second fibronectin-binding protein, prtF2 (Podbielski, Woischnik, Leonard, & Schmidt, 1999). Transcriptome analysis of a serotype M49 strain and its isogenic nra mutant strain showed that Nra is active in the exponential, transition, and stationary growth phases (Kreikemeyer, et al., 2007). Maximum expression of Nra occurs in early stationary phase growth (Podbielski, Woischnik, Leonard, & Schmidt, 1999), and the highest number of differentially transcribed genes was observed in the transition phase of growth, with an Nra-knockout strain displaying 112 and 84 genes with increased and decreased transcript abundance, respectively (Kreikemeyer, et al., 2007). However, unlike some RofA-expressing strains, Nra does not appear to respond to changing atmospheric conditions (Podbielski, Woischnik, Leonard, & Schmidt, 1999). Nra has been shown to repress the transcription of FCT region genes, pilus protein-encoding genes, and the capsule biosynthesis operon (hasABC) (Kreikemeyer, et al., 2007). As a master regulator, Nra also represses virulence factors encoded by the Mga core regulon, and in the M49 serotype background, Nra has been observed to directly or indirectly repress expression of the global regulator Rgg, the Ihk/Irr TCS, additional RALPs (Ralp3 and RivR), and Mga itself (Podbielski, Woischnik, Leonard, & Schmidt, 1999; Kreikemeyer, et al., 2007). For other virulence genes, a negative regulatory effect of Nra has only been shown during one or two growth phases. These genes encode prtF2, a putative hemolysin (hylX), two hyaluronidases (hylP2 and hylP3), an extracellular matrix-binding protein (epf), the laminin-binding protein (lmb), speA, and ska.
Ralp3 is encoded in the so-called eno-ralp3-epf-sagA (ERES) pathogenicity region, which is identified in selected M serotypes (M1, 4, 12, 28 and 49) where it controls the expression of Epf and Streptolysin S (Kreikemeyer, et al., 2007). Inactivation of Ralp3 in an M49 strain resulted in a diminished binding capacity to human plasminogen, and SpeB activity was significantly decreased (Siemens, et al., 2012). Transcriptome analysis identified 16 genes as up regulated, and 43 genes as down regulated in the ralp3 mutant. Among those down regulated were the lac operon and the fru operon (encoding proteins involved in metabolism), the putative salivaricin operon (sal), and the whole Mga core regulon. The importance of the RALPs in metabolic control will be further discussed in the corresponding section. Every S. pyogenes strain tested to date encodes at least RofA or Nra, and some strains can express several RALP family members, which results in a widely variable combination of RALP-regulated genes and patterns of gene expression between different serotypes (Beckert, Kreikemeyer, & Podbielski, 2001; Podbielski, Woischnik, Leonard, & Schmidt, 1999; Kreikemeyer, Beckert, Braun-Kiewnick, & Podbielski, 2002; Kreikemeyer, et al., 2007).
Ralp4, or RivR (which stands for RALP, roman numeral “iv”) was identified and characterized as a RALP, based on its 29% identity with RofA and 32% identity with Nra at the amino acid level (Roberts, Churchward, & Scott, 2007). Since phosphorylated CovR represses expression of RivR (Roberts, Churchward, & Scott, 2007), analysis of the RivR-regulated transcriptome in a MGAS5005 (M1T1) strain required its deletion and complementation in a ∆covR background (Roberts & Scott, 2007). This study showed that the overexpression of RivR resulted in the altered transcription of 31 genes, among which there were multiple virulence related genes that were either induced, in the case of endoS and several genes of the Mga regulon (emm, scpA, fba, mga, sic, scl and grm), or repressed by RivR activity. Further characterization revealed that RivR enhances transcriptional activation by Mga in vitro, increases expression of the Mga regulon in vivo, and is necessary for virulence in a CovR-deficient strain (Roberts & Scott, 2007). Additionally, as previously indicated, RivR also acts as a repressor of hasABC and grab expression in a CovR-dependent manner in two distinct M1T1 serotype strains (MGAS2221 and MGAS5005) (Treviño, Liu, Cao, Ramirez-Peña, & Sumby, 2013), and RivR repression abrogates S. pyogenes survival in human blood (Ramalinga, Danger, Makthal, Kumaraswami, & Sumby, 2016). In contrast to the earlier report (Roberts & Scott, 2007), the Mga regulon was not activated by RivR in these M1T1 serotype strains (Treviño, Liu, Cao, Ramirez-Peña, & Sumby, 2013). Like Mga, RivR forms both dimers and a higher-molecular-mass multimer to exert its regulatory activity on hasA and grab transcripts (Ramalinga, Danger, Makthal, Kumaraswami, & Sumby, 2016). Using a library of expression plasmids in which each of the four cysteines in RivR was mutagenized to a serine, it was demonstrated that cysteine residues at positions 32, 377, and 470 are required for RivR dimerization.
Metabolite responsive regulators
Sensing and responding to changes in metabolite availability within a host is an integral part of virulence gene regulation in pathogenic bacteria, as virulence gene expression enables these organisms to infect and colonize the host. As a result, carbohydrate sources of energy, amino acid/nitrogen supplies necessary for protein and nucleic acid synthesis, as well as metal ions required for biochemical processes, are the principal metabolites that a pathogen like S. pyogenes must monitor throughout the course of host colonization. Here we summarize the current understanding of the principal virulence gene regulators in S. pyogenes whose activity is directly determined by the availability of these metabolites.
Carbohydrate catabolite regulation (CcpA, LacD.1, Mga)
Bacteria of multiple species have been observed to coordinately alter the expression of carbohydrate utilization genes and virulence factor production in response to changes in carbohydrate availability (Moreno, Schneider, Maile, Weyler, & Saier, Jr., 2001; Deutscher, Francke, & Postma, 2006). Glucose is the primary carbohydrate source of energy for many bacteria, including S. pyogenes, which generates ATP from its conversion to pyruvate through the Embden-Meyerhof pathway. However, the utilization of alternative carbohydrates requires energy input to convert them to glucose in order to be metabolized. As a result, bacteria ensure that genes involved in alternative carbohydrate utilization are not expressed when glucose is available, through a process known as carbon catabolite repression (CCR) (Deutscher, Francke, & Postma, 2006). In S. pyogenes, CCR is not restricted to the regulation of carbohydrate catabolic enzymes and can affect the expression of virulence determinants via carbohydrate-sensitive regulators, such as CcpA, LacD.1, and Mga (Table 3, 4).
Phosphoenolpyruvate Phosphotransferase System (PTS) regulation
Regulatory pathways of carbohydrate metabolism contribute to S. pyogenes pathogenesis, including those involving phosphoenolpyruvate phosphotransferase system (PTS) components. PTS activity has been associated with S. pyogenes survival in whole human blood, and mutagenesis of PTS genes affects virulence factor expression (e.g., SLS) in a murine model of soft tissue infection (Gera, Le, Jamin, Eichenbaum, & McIver, 2014; Valdes, et al., 2016). Insertional inactivation of the 14 annotated PTS EIIC-encoding genes in an M1 serotype S. pyogenes determined that the mannose-specific EII locus (manLMN) acts to prevent the early onset of SLS-mediated hemolysis (Sundar, Islam, Gera, Le Breton, & McIver, 2017). Additional investigation of PTS permease (EIIC) genes identified the annotated β-glucoside PTS transporter (bglP) as a contributor to S. pyogenes growth and survival in human blood (Braza, et al., 2020).The operon encoding bglP, including antiterminator licT and phospho-β-glucosidase bglB, is repressed by glucose and induced by salicin. Mutants that lack bglP and bglB exhibit in vitro growth defects in distinct carbohydrate sources (fructose/mannose/salicin and salicin alone, respectively) as well as altered biofilm formation and SLS production affecting ulcerative lesion progression during subcutaneous infections in mice (Braza, et al., 2020).
CcpA and LacD.1
The catabolite control protein (CcpA) is a member of the LacI/GalR family of transcription factors that is common among Gram-positive pathogens, and a thorough review of its characterization can be found in (Deutscher, Francke, & Postma, 2006). Briefly, CcpA function centers on the activity of the phosphoenolpyruvate phosphotransferase system (PTS), which regulates carbohydrate uptake via a phosphorylation relay pathway that involves cytosolic (EI, HPr) and membrane bound (EII) enzymes (Deutscher, Francke, & Postma, 2006). Of these, HPr is tasked with determining the energy level of the cell by undergoing differential phosphorylation by the PTS and an HPr kinase at conserved histidine and serine residues, respectively. Phosphorylation of the serine residue enables the interaction of HPr with CcpA in order for CcpA to act as a carbohydrate-dependent transcriptional repressor by binding DNA at a palindromic consensus sequence in the promoter or coding regions of CCR-transcripts, known as a catabolite-responsive element or cre site (Deutscher, Francke, & Postma, 2006; Fujita, Miwa, Galinier, & Deutscher, 1995). Though primarily a repressor, CcpA is also known to function as an activator of transcripts that are required for glucose-dependent growth in other Gram-positive bacteria (Grundy, Waters, Allen, & Henkin, 1993). Transcriptome analysis of a MGAS5005 (M1T1) strain and its isogenic ccpA deletion mutant revealed that the transcription of 124 genes (~6% of the S. pyogenes genome) is affected by CcpA (Kinkel & McIver, 2008). In addition to the expected repression of genes relating to non-glucose sugar utilization, CcpA has influenced expression of virulence related genes, including the sag operon (repressing) and rivR (inducing). Under glucose starved conditions, the expression of additional virulence related genes (speB, mac, spd3) were affected in the same CcpA-deficient strain (Shelburne, et al., 2008).
A combination RNAseq and ChIPseq analysis determined that CcpA affects transcript levels of 514 M1 serotype S. pyogenes genes, of which 105 demonstrated direct DNA binding by CcpA, including those that encode the cytotoxin SLS, as well as PrtS and SpeB proteases (DebRoy, et al., 2021). Abolishing the interaction of CcpA with its co-factor HPr by mutation of a valine residue (V301A) affected the transcript levels of 205 genes (40%) of the CcpA regulon. ChIPseq analysis showed the mutagenized CcpAV301A protein bound to 74% of gene targets bound by wild-type CcpA with lower affinity, thus elucidating HPr-dependent and independent virulence and metabolic gene regulation by CcpA in S. pyogenes (DebRoy, et al., 2021). Among the genes affected by Hpr-dependent CcpA regulation were prtS, lctO, arcA and malX. Analysis of CcpA interaction with a cre site in the promoter region of mga in the JRS4 (M6) strain revealed that CcpA is necessary for full expression of the virulence regulator Mga in the late logarithmic phase of growth (Almengor, Kinkel, Day, & McIver, 2007). CcpA regulatory activity appears to be exerted both directly and indirectly and varies among strains, as CcpA was observed to bind to the cre site of the mga promoter in JRS4 (Almengor, Kinkel, Day, & McIver, 2007)and speB in the HSC5 (M14) strain, but not sagA in the latter, despite still repressing the expression of the sag operon (Kietzman & Caparon, 2010).
Comparison in murine models of S. pyogenes virulence of a ∆ccpA strain with another mutant expressing CcpA locked into a high-affinity DNA-binding conformation (CcpAT307Y) suggests that CcpA activity contributes to balancing bacterial growth with host tissue invasion (Paluscio, Watson, Jr., & Caparon, 2018). The ∆ccpA and CcpAT307Y mutants displayed distinct patterns of virulence in models of acute soft tissue infection and of long-term mucosal colonization. The ∆ccpA strains exhibited reduced ability to grow in tissue, reduced tissue damage, and early clearance. The CcpAT307Y strain produced high tissue burdens and extended time of carriage, accompanied with reduced tissue damage (Paluscio, Watson, Jr., & Caparon, 2018).
The CcpA regulon shares virulence gene targets with the CovR regulon, as demonstrated by the characterization of CcpA-deficient (2221∆ccpA), CovR-deficient (2221∆covR), and doubly-deficient (2221∆ccpA∆covR) mutant strains in an M1T1 background with a functional CovS (MGAS2221), as well as by comparison with similarly constructed mutations in a CovS-deficient strain (MGAS5005) (Shelburne, et al., 2010). According to this study, CcpA and CovR co-regulate transcription of the virulence factor encoding genes nga, slo, spyCEP, sagA, sdaD2, endoS, fba, and speB. CcpA directly regulates expression of these genes in the strains examined, as CcpA and its complex with phosphorylated HPr protein, CcpA-(HPr-Ser46-P), bind with high affinity to cre sites within their promoters. However, this shared regulation is dependent upon the status of CovS functionality; CcpA influences speB, slo, and spyCEP expression during in vitro growth only when CovS is functionally active (Shelburne, et al., 2010). Notably, CovR and CcpA do not regulate each other’s expression, at least not directly in the strains and conditions examined—despite the fact that all of the virulence genes affected by CcpA inactivation are also affected by CovR inactivation (Shelburne, et al., 2010).
The CCR of virulence-related genes by CcpA occurs in conjunction with the activity of another carbohydrate-dependent regulator, called LacD.1. Identified as a glucose responsive regulator of the SpeB protease (Loughman & Caparon, 2006a), LacD.1 is a member of the Lac.1 operon and has the characteristics of a tagatose 1,6-bisphosphate aldolase involved in lactose and galactose metabolism. Though LacD.1 possesses enzymatic activity, it does not function to metabolize galactose (Loughman & Caparon, 2006a; Loughman & Caparon, 2007). LacD.1 regulatory function is independent of its catalytic activity, but the protein must bind to its substrate to exert its regulation of SpeB, a function that appears to have evolved by conferring increased fitness to S. pyogenes, as observed using competitive assays under in vitro growth conditions that mimic deep tissue environments (Cusumano & Caparon, 2013). An in vivo analysis of the carbon catabolite-responsive regulatory pathways constituted by CcpA and LacD.1 revealed that they can regulate up to 15% of the genome in response to glucose, and that together, they contribute to regulation of 60% of this subset in various combinations, including CcpA and LacD.1-specific and independent regulation, co-regulation, and even regulation independent of glucose (Kietzman & Caparon, 2011). Among the affected genes were several secreted factors, putative virulence genes, and previously characterized genes that affect virulence, such as sagA and lctO (Kietzman & Caparon, 2010). The most important virulence gene affected by CcpA and LacD.1 co-regulation is speB, which was induced by CcpA and repressed by LacD.1 both in vitro and in vivo. In the latter condition, CcpA and
LacD.1 mutants evinced mis-regulation of SpeB at separate and distinct phases of infection, which highlights the spatial and temporal role that carbon catabolite sensing appears to play in virulence-related gene regulation during the course of infection (Kietzman & Caparon, 2011). Another important finding of this study was that multiple transcripts are responsive to glucose independent of CcpA and LacD.1 activity, which indicates that other carbohydrate responsive regulators act in S. pyogenes. One such regulator appears to be the virulence gene-related transcription factor Mga.
Mga
Mga is a large 500 amino acid DNA-binding protein with an approximate molecular size of 62 kDa. Orthologous proteins can be found in many pathogenic streptococci, including Streptococcus dysgalactiae, S. pneumoniae, S. equi, S. gordonii, S. mitis, S. sanguinis, and S. uberis (Geyer & Schmidt, 2000; Vasi, Frykberg, Carlsson, Lindberg, & Guss, 2000; Hava & Camilli, 2002; Vahling & McIver, 2006). Mga was first identified in S. pyogenes through spontaneous small deletions at a locus just upstream of the emm gene that resulted in M protein-negative strains (Spanier, Jones, & Cleary, 1984), and was later confirmed as a positive regulator of emm transcription and M protein production (Caparon & Scott, 1987). The identified loci immediately upstream of emm was predicted to produce a protein with homology to a DNA-binding transcriptional regulator (Perez-Casal, Caparon, & Scott, 1991; Chen, Bormann, & Cleary, 1993) and was eventually designated as mga for multiple gene regulator of group A streptococcus (Scott, et al., 1995). Mga is expressed in all S. pyogenes strains and its encoding gene is found in all strains examined to date (Podbielski, 1992; Bessen, Manoharan, Luo, Wertz, & Robinson, 2005). Its sequence varies up to 21% among S. pyogenes strains, and two divergent alleles of mga (mga-1, mga-2) have been identified (Haanes & Cleary, 1989; Hollingshead, Readdy, Yung, & Bessen, 1993), although the expressed Mga proteins maintain greater than 97% amino acid sequence identity. The mga-1 allele is linked to serum opacity factor negative (SOF-) class I strains isolated from throat infections, while the mga-2 allele is almost exclusively observed in SOF+ class II strains associated either with skin infections or with “generalist” strains (Bessen, Manoharan, Luo, Wertz, & Robinson, 2005), which suggests that the divergent Mga regulons likely evolved to adapt to distinct tissue environments (Bessen, Manoharan, Luo, Wertz, & Robinson, 2005).
Mga induces transcription of its encoding gene (mga) (Geist, Okada, & Caparon, 1993; Podbielski, Flosdorff, & Weber-Heynemann, 1995; McIver, Thurman, & Scott, 1999) and a core set of virulence genes during exponential growth in vitro. These include cell wall-associated surface molecules in addition to M protein involved in host tissue adherence, internalization into non-phagocytic cells and avoidance of the host innate and adaptive immune responses. The regulatory role of Mga during the course of infection in different tissue environments will be covered later in this chapter. Mga induces the transcription of multiple genes located downstream of mga and emm in a discrete region of the chromosome called the mga locus, identified in all S. pyogenes genomes thus far examined. This region includes, in varying organization and combinations depending on serotype, a cell wall-associated C5a peptidase (scpA), the M-like surface proteins Mrp (fcrA) and Enn (enn), secreted inhibitor of complement (sic), and a fibronectin-binding surface adhesin (fba) (Haanes, Heath, & Cleary, 1992; Podbielski, 1993; Hollingshead, Arnold, Readdy, & Bessen, 1994). The scpA gene product is involved in cleavage of the C5a chemotaxin, which inhibits recruitment of PMNs to infectious sites (Simpson, LaPenta, Chen, & Cleary, 1990). Mrp (fcrA) binds IgG and fibrinogen, and can provide resistance to phagocytosis; while Enn generally binds IgA, though antiphagocytic properties for it have not been shown (Bessen & Fischetti, 1992; Courtney & Li, 2013). The product of sic has the ability to block the membrane attack complex (MAC) of the complement, as well as inhibit the activity of innate immune effectors, such as lysozyme and host antimicrobial peptides (Akesson, Sjöholm, & Björck , 1996; Fernie-King, et al., 2001; Frick, Akesson, Rasmussen, Schmidtchen, & Björck, 2003). Fba constitutes a fibronectin-binding surface adhesin that is able to bind the complement regulators factor H and factor H-like protein 1 to block opsonophagocytosis (Terao, et al., 2001; Pandiripally, Gregory, & Cue, 2002). However, not all Mga-regulated genes are located within the mga locus. The fibronectin-binding surface proteins SfbX and SOF that enable S. pyogenes to interact with the host ECM (Courtney, et al., 1999; Jeng, et al., 2003) are encoded by sof and sfbX 12 kb away from mga in class II SOF+ strains, and their co-transcription is activated by Mga (Green, et al., 2005). Mga also influences the expression of sclA, a gene that is found over 30 kb upstream of the mga locus in a subset of serotypes and that encodes the streptococcal collagen-like protein A (sclA/scl1). This repeat-containing surface molecule found on all S. pyogenes strains absorbs human plasma LDL and binds to α2β1 integrins on host cells, which allows for internalization and subsequent re-emergence as a means of immune evasion (Caswell, Lukomska, Seo, Höök, & Lukomski, 2007). As previously indicated, Mga can also regulate the transcription of speB, which modulates the production of the secreted SpeB cysteine protease (Podbielski, Flosdorff, & Weber-Heynemann, 1995; Ribardo & McIver, 2006).
Of the carbohydrate-responsive virulence gene regulators, Mga is the best characterized in terms of its mechanism of action. A more thorough overview of Mga binding site and domain architecture can be found in (Hondorp & McIver, 2007; Rom, Hart, & McIver, 2021). The binding of Mga to upstream promoter regions is essential for high-level transcriptional activation of core regulon genes (McIver & Myles, 2002). Mga binding sites within these promoters vary widely in location with respect to the start of transcription and have thus far been classified into three categories based on biochemical studies ((Almengor & McIver, 2004), reviewed in (Hondorp & McIver, 2007)). Initial DNase I footprinting experiments indicate that Mga protects a large 45- to 59-base-pair non-palindromic region of DNA (McIver, Thurman, & Scott, 1999; McIver, Heath, Green, & Scott, 1995), and these studies suggest a nominal consensus sequence to guide the search for Mga binding sites. A mutagenesis-based analysis of the M1T1 emm promoter binding site (Pemm1) identified 34 nucleotides within Pemm1 that had an effect either on Mga binding, on Mga-dependent transcriptional activation, or on both (Hause & McIver, 2012). Among these critical nucleotides, guanines and cytosines within the major groove were disproportionately identified as clustered at the 5' and 3' ends of the binding site, along with runs of nonessential adenines between the critical nucleotides. On the basis of these results, a Pemm1 minimal binding site of 35 bp bound Mga was found at a level comparable to the level of binding of the larger 45-bp site. However, as more sites have been found, the sequence has become less defined, due to lack of conservation between the various promoters, and exhibits only 13.4% identity with no discernible symmetry (Hause & McIver, 2012). Nonetheless, in vitro transcription assays have established that Mga is sufficient to activate expression (Almengor, Walters, & McIver, 2006), so while additional factors might modify Mga regulation in vivo, they are not required. Despite the variability in promoter sites, Mga seems to employ the same protein domain(s) to bind locations with little sequence homology, as evidenced by competition of Pemm and PscpA for Mga binding to Pmga (McIver, Thurman, & Scott, 1999).
Mga has two conserved helix–turn–helix (HTH) domains, HTH-3 (residues 53–72) and HTH-4 (residues 107–126), that map near the N-terminus of the protein (Chen, Bormann, & Cleary, 1993; Podbielski, Flosdorff, & Weber-Heynemann, 1995; McIver & Myles, 2002). These domains have been shown to be required for DNA binding and the activation of transcription for all genes studied (Vahling & McIver, 2006; McIver & Myles, 2002). While HTH-4 is absolutely essential to these processes, HTH-3 appears to serve more of an accessory role during autoactivation. These HTH domains appear to be present in other virulence regulators that do not share homology throughout the entire length of the protein with Mga, such as the RALPs. This has led to the definition of the specific Pfam domains “HTH_Mga” and “Mga,” which encompass HTH-3 and HTH-4, respectively (Finn, et al., 2006). An in silico analysis comparing Mga to proteins of known structure found in the SCOP database found established Mga DNA-binding domains (Andreeva, Howorth, Brenner, Hubbard, Chothia, & Murzin, 2004; Vahling, 2006), as well as two regions within Mga that were predicted to have strong homology to the dual PTS regulatory domains (PRD) in the antiterminator LicT (Hondorp & McIver, 2007; Rom, Hart, & McIver, 2021). The overall size and domain structure of Mga, with N-terminal DNA-binding domains and two central PRD domains, closely resembles that of known PRD-containing transcriptional activators such as MtlR (Vahling, 2006). Subsequently, the Pfam database now designates these regions as a “PRD_Mga” domain, which is a member of the PRD superfamily (Finn, et al., 2006). Once the high-level purification of Mga was achieved, gel filtration analyses, analytical ultracentrifugation and co-immunoprecipitation experiments have demonstrated that Mga oligomerizes in solution, and that its ability to oligomerize correlates with transcriptional activation (Hondorp, et al., 2012). Examination of the previously uncharacterized C-terminus detected structural homology to EIIB domains used by the PTS. Truncation analyses of Mga from an M4 and an M1 strain revealed the C-terminal region of Mga is required for oligomerization and in vivo transcriptional activity, but not DNA binding (Hondorp, et al., 2012). Conversely, the DNA binding domains of Mga are necessary, but are insufficient to induce the expression of Mga-regulated transcripts.
As mentioned, Mga contains two central PRD domains that are similar to antiterminators (such as LicT), and activators (such as MtlR and LicR) that are involved in the regulation of sugar metabolism (reviewed in Deutscher, Francke, & Postma, 2006; Hondorp & McIver, 2007; Rom, Hart, & McIver, 2021). The activity of these regulators is modulated by phosphorylation of conserved histidine residues within their PRD domain(s) via the PTS phosphorelay in response to the utilization of different carbohydrate sources, as observed in the B. anthracis Mga homolog, AtxA (Tsvetanova, et al., 2007; Hammerstrom, et al., 2015). An M4 strain with a nonfunctional PTS that results from the deletion of the EI-encoding ptsI gene exhibited a decrease in Mga activity, and characterization of purified Mga showed that it could be phosphorylated at conserved PRD histidines by EI and HPr components of the PTS in vitro (Hondorp, et al., 2013). Investigation of glucose-dependent changes in the Mga regulon using in vitro RNAseq analysis revealed distinct sets of genes were differentially expressed in high glucose (THY medium) versus low glucose (C medium) conditions (Valdes, et al., 2018). Mga regulon genes activated in C medium correlated with those repressed by CcpA. Detected medium-associated changes in the phosphorylation status of Mga support a regulatory framework in which differences in the Mga regulon correspond to the effect of environmental glucose levels on PTS-mediated regulation of Mga (Valdes, et al., 2018). Confirmation that Mga activity is directly influenced by the PTS came from examination of Mga transcriptional activity using alanine (unphosphorylated) and aspartate (phosphomimetic) substitution mutations of conserved Mga PRD histidines, which established that a doubly-phosphorylated PRD1 phosphomimetic Mga (D/DMga4) is completely inactive in vivo and shuts down the expression of the Mga regulon (Hondorp, et al., 2013). Although the D/DMga4 protein is still able to bind DNA in vitro, homo-multimerization of Mga is disrupted and the protein is unable to activate transcription. As a result, carbohydrate availability can be linked to Mga-dependent expression of virulence-related genes. These studies also highlighted the role of a PRD-containing virulence regulator (PCVR) like Mga (Hondorp & McIver, 2007; Rom, Hart, & McIver, 2021) for pathogenesis, as both non-phosphorylated and phosphomimetic PRD1 Mga mutants were attenuated in a model of S. pyogenes invasive skin disease (Hondorp, et al., 2013). A similar study in an M59 serotype background that is highly polymorphic in Mga found that phosphomimetic substitutions at both PRD1 and PRD2 conserved histidine residues are inhibitory to Mga-dependent gene expression and attenuated S. pyogenes virulence by influencing Mga-dependent global gene regulation (Sanson, et al., 2015). Conversely, non-phosphorylation-mimicking alanine substitutions relieved inhibition and the mutants exhibited a wild-type phenotype in a mouse model of necrotizing fasciitis. These studies suggest that adequate regulation (positive and negative) of Mga activity as a PCVR is necessary for virulence and varies between genotypic backgrounds—an important finding, given that PCVRs appear to be widespread among Gram-positive pathogens (Rom, Hart, & McIver, 2021).
Mga expression is both autoregulated and influenced by other regulators. Transcription from Pmga appears not to be autoregulated in response to carbohydrate availability, as sensed by the PTS (Hondorp, et al., 2013). Nonetheless, Pmga is required for growth phase-regulated expression of the Mga regulon, which indicates that environmental regulation still occurs at the level of mga transcription (Okada, Geist, & Caparon, 1993; McIver & Scott, 1997). Such regulation of Pmga does not appear to occur through the direct influence of two-component signal transduction systems (Ribardo, Lambert, & McIver, 2004), although TCSs are involved in inducing transcription of Mga-regulated virulence genes in other serotypes, as was seen in the TrxRS-mediated activation of Pmga demonstrated by analysis of a luciferase reporter fusion in a MGAS5005 (M1) strain (Leday, et al., 2008). As previously indicated, CcpA binds to Pmga and maintains high-level mga expression during exponential growth (Almengor, Kinkel, Day, & McIver, 2007). Deletion analysis of Pmga identified a region overlapping the Mga binding site I that is involved in repression of mga transcription (McIver, Thurman, & Scott, 1999). Some RALPs (i.e., RofA, RALP3 and Nra) are known to repress mga expression (Beckert, Kreikemeyer, & Podbielski, 2001; Podbielski, Woischnik, Leonard, & Schmidt, 1999; Kwinn, et al., 2007). Another regulator, Rgg/RopB (Federle, 2012), has also been shown to repress Mga expression (Chaussee, et al., 2002); however, direct interaction of these at Pmga has not been demonstrated. In contrast, RivR (Ralp4) activity in an M1 strain is able to enhance the activation of mga and Mga-regulated genes both in vitro and in vivo, though this is controversial (Roberts, Churchward, & Scott, 2007; Treviño, Liu, Cao, Ramirez-Peña, & Sumby, 2013; Roberts & Scott, 2007). The inactivation of amrA, which produces a putative Wzx integral membrane sugar exporter, was found to be essential for exponential-phase expression of mga in a serotype M6 strain (Ribardo & McIver, 2003), possibly by altering sugar levels in the cell.
All of these studies suggest a general model for how changes in environmental carbohydrates influence expression of the Mga-regulated virulence repertoire of S. pyogenes (Figure 2). While glucose (the preferred carbohydrate source) is abundantly available, CcpA induction combined with reduced PTS activity and Mga autoregulation maintain the high-level expression of Mga and its regulon. As glucose becomes scarce, induction by CcpA decreases and mga transcription is reduced, but is maintained by autoregulation. As PTS activity increases to supply the bacterium with alternate carbohydrate sources, Mga activity is repressed by the PTS-dependent phosphorylation of its PRD region. Other factors like Rgg and RALPs, whose regulatory activity is mostly exerted under the stationary phase of growth when carbohydrate energy sources are exhausted, further maintain the repression of Mga until environmental carbohydrate supplies become available. Modulation of RivR by the CovR/S master regulator to influence Mga activity serves to further fine-tune the expression of the Mga regulon. Naturally, this is an oversimplified model that fails to account for the contributions made by different genomic backgrounds, the organization of regulatory regions, or the input of master regulators in controlling the Mga regulon. However, this model might be useful in guiding elucidation of the complex virulence gene regulatory network influencing S. pyogenes pathogenesis in response to changes in carbohydrate availability, which is an area of increasing research interest.
Amino acid and nitrogen availability (Rsh, CodY, and Rny)
The primary sites of S. pyogenes infection are potentially rich in nutrients in the form of abundant peptides and proteins, but are somewhat lacking in free amino acids (Steiner & Malke, 2000). As a multiple-amino-acid-auxotrophic pathogen (Ferretti, et al., 2001; Davies, Karush, & Rudd, 1965), S. pyogenes may encounter starvation for free amino acids when attempting to propagate in these host niches during specific stages of the infection process; most likely when initially contacting the skin or throat as primary infection sites (Steiner & Malke, 2001). Although for S. pyogenes found on the skin, the existence of a persistent carrier state seems unlikely, the organism may exist in an asymptomatic state harbored by the oropharynx where it appears to constitute the principal reservoir for S. pyogenes in the environment (Pichichero, 1998). The stasis-like carrier state may also typify the physiological state of bacteria that adhere to epithelial cells and succeed in invading them. Additionally, S. pyogenes may encounter host nutritional deficiencies when persisting at high cell densities at infection sites or initiating life-threatening invasive disease (such as streptococcal toxic shock syndrome, necrotizing fasciitis, or septicemia) (Steiner & Malke, 2001). Given the poly-auxotrophy of S. pyogenes and the fact that restriction of an amino acid immediately limits the availability of the corresponding aminoacyl-tRNA (Emilsson & Kurland, 1990), amino acid supply is a central problem for its survival and propagation. Multiple studies indicate that S. pyogenes has evolved a stimulus response network that involves peptidases, proteinases, and peptide and amino acid transport systems in order to generate the free amino acids necessary for growth, which counteracts amino acid starvation and enables the pathogen to mount a dynamic response to the protein-rich environment provided by its human host. In this section, we summarize the regulation of this network in relation to the control of S. pyogenes virulence.
Rsh: stringent and relaxed responses to amino acid starvation
Originally cloned and sequenced (Mechold, Steiner, Vettermann, & Malke, 1993), as well as structurally and functionally characterized (Hogg, Mechold, Malke, Cashel, & Hilgenfeld, 2004; Mechold, Cashel, Steiner, Gentry, & Malke, 1996; Mechold & Malke, 1997), the stringent factor from S. dysgalactiae subsp. equisimilis (group C streptococci) has proved to be of seminal importance to the subsequent establishment of the RelA/SpoT homolog (Rsh) superfamily of bifunctional guanosine 5’-3’ polyphosphate ((p)ppGpp) synthetases and hydrolases and their distribution and function across all living organisms (Atkinson, Tenson, & Hauryliuk, 2011)). Given the amino acid sequence identities of the order of 95% (97% similarity) between the group C streptococci query sequence (739 residues) and the available S. pyogenes sequences, as well as identical linkage relationships of the respective genomic regions (Ferretti, et al., 2001; Mechold, Steiner, Vettermann, & Malke, 1993), all basic properties established for the group C streptococcus protein can also safely be taken to apply to the S. pyogenes homolog. This assumption was also confirmed in a recent review on S. dysgalactiae (Alves-Barroco, et al., 2021) and, henceforth, the GCS protein and its S. pyogenes homolog will be specified as RshStr. Like all full-length Rsh proteins, RshStr synthesizes the alarmone (p)ppGpp at the expense of ATP and GTP when cognate, but deacylated tRNAs occupy the aminoacyl acceptor site of the ribosomes (Hogg, Mechold, Malke, Cashel, & Hilgenfeld, 2004; Mechold, Cashel, Steiner, Gentry, & Malke, 1996; Mechold & Malke, 1997). Consequently, ribosomal complexes on mRNA templates are stalled, which prevents wasteful macromolecular syntheses by a rapid shutdown of futile RNA synthesis in stressful metabolic conditions, like amino acid starvation. Alarmone signaling pathways are primarily directed towards transcriptional inhibition of the stable RNAs, such as rRNA and tRNA (the so-called stringent response, reviewed in (Potrykus & Cashel, 2008). Accordingly, under specific nutritional stress conditions, S. pyogenes synthetase-deficient RshStr insertion mutants with rshStr truncated at codons 220 or 129 show survival rates about three orders of magnitude lower than wild types. The basic reason for this deficiency is the energetically wasteful continuation of RNA synthesis during amino acid deprivation (the so-called relaxed response (Steiner & Malke, 2000; Mechold & Malke, 1997; Potrykus & Cashel, 2008)).
As shown by functional RshStr analyses, this enzyme has also a strong (p)ppGpp hydrolytic activity that gives rise to GTP/GDP plus pyrophosphate (Mechold, Cashel, Steiner, Gentry, & Malke, 1996). The first-order rate constants of (p)ppGpp turnover reflect short half-lives of 20 and 60 sec for the penta- and tetraphosphates, respectively, of the alarmone, which enables the cells to recover quickly from the stasis-like survival state under improved nutritional conditions (Mechold, Cashel, Steiner, Gentry, & Malke, 1996). Defined RshStr fragments created by cloning and functionally analyzed by enzymatic activity assays showed that the two opposing activities reside in the 1-385 residue N-terminal half, bridged by an exposed hinge region to the C-terminal half, which is suggested to be involved in regulatory intramolecular interactions or intermolecular interactions with the ribosome (Atkinson, Tenson, & Hauryliuk, 2011; Mechold, Murphy, Brown, & Cashel, 2002). Dissecting the N-terminal half more precisely by a nested deletion analysis, combined with enzymatic activity assays of purified peptide fragments, revealed non-overlapping minimal fragments of residues 1-198 and 211-347 for full hydrolytic and synthetic activities, respectively (Mechold, 2009).
One challenging problem posed by the opposing enzymatic activities of RshStr concerned the prevention of futile cycling of the alarmone metabolism, which would waste ATP and hamper the rapid adjustment of (p)ppGpp levels as dictated by the prevailing niches of infection in vivo and indeed demonstrated in vitro (Mechold & Malke, 1997; Mechold, Murphy, Brown, & Cashel, 2002) and Figure 3). However, the resolved X-ray structure of the catalytic RshStr region (residues 1-385), which is the first to be established for any Rsh homolog, presented this fragment in distinct hydrolase-OFF/synthetase-ON and hydrolase-ON/synthetase-OFF conformations (Hogg, Mechold, Malke, Cashel, & Hilgenfeld, 2004). The structure localized the opposing enzymatic activities >30 A apart and rendered them mutually exclusive by a combination of induced fit principles and nucleotide ligand-induced intramolecular allosteric signaling between the opposing active sites (Hogg, Mechold, Malke, Cashel, & Hilgenfeld, 2004; Mechold, 2009). As a result, structural antagonism rules out the in vivo possibility of simultaneous (p)ppGpp synthesis and hydrolysis.

Figure 3.
Synthesis and hydrolysis of (p)ppGpp catalyzed by bifunctional RshStr.
Continuing this line of research, researchers (Mechold, Potrykus, Murphy, Murakami, & Cashel, 2013) have developed strategies that enable them to fine-tune the differential accumulation of pppGpp and ppGpp by activating different RshStr synthetic fragments in combination with E. coli pppGpp 5’-gamma phosphate hydrolase to get rid of the pentaphosphate and generate only ppGpp. They found that ppGpp is a more efficient regulator than pppGpp with respect to a plethora of five stress responses, with the most important one in the present context being rRNA synthesis regulation. Reducing the aura of the alarmone even further, multiple groups found that both nucleotides bind to a site at the interface between the beta’ and omega RNAP subunits (Ross, Vrentas, SanchezVasquez, Gaal, & Gourse, 2013; Zuo, Wang, & Steitz, 2013). This finding supports a model where ppGpp connects the core and the shelf of RNAP to form a mobile clamp around the DNA. By controlling the opening and closing of the clamp through an allosteric mechanism, ppGpp modulates RNAP activity. However, since the structural results were obtained with RNAP holoenzyme from E. coli, it remains to be seen if RNAP from S. pyogenes will behave in a similar fashion.
Apart from its primary function of stable RNA synthesis inhibition, (p)ppGpp also affects negatively the transcription of important groups of protein-encoding S. pyogenes genes, including regulators, virulence factors and genes for transport systems (Malke, Steiner, McShan, & Ferretti, 2006). Of pivotal importance is the strong negative stringent control of rshstr itself. Exposure to isoleucine plus valine starvation results in 40-fold changes in rshstr expression in an rshstr mutant, relative to the wild type. This high degree of negative autoregulation may be necessary for a gene with broad connectivity in order to sustain cellular homeostasis. Among 31 additional genes studied for their expression during the stringent response (and found to be strongly negatively controlled) were the virulence factors graB (see above) and speH encoding the superantigen pyrogenic exotoxin H. An approximately tenfold expression increase under relaxed conditions was also observed for the important branched-chain amino acid (BCAA, see below) transporter gene braB, which reflects a cost-effective means for the repression of a futile transport process under nutrient-rich conditions.
CodY: (p)ppGpp-independent response to amino acid starvation.
Experiments in which both S. pyogenes wild type and rshStr mutant strains were exposed to BCAA starvation led to the unexpected discovery of an rshStr-independent mode of global transcriptional regulation (Steiner & Malke, 2000; Steiner & Malke, 2001). This regulatory response to the deprivation of the essential amino acids isoleucine and valine is characterized by the up-regulation of a wide array of housekeeping genes, as well as accessory and dedicated virulence factors in both isogenic genotypes. Up-regulation under these conditions also applies, for example, to the CovRS regulation of virulence gene expression in a global fashion. Importantly, of the operons affected by the rshStr-independent response, the oligopeptide permease (opp), FAS regulatory system (fasBCAX) and streptolysin S operon (sag), exhibit internal transcriptional termination under nutritionally rich conditions. However, BCAA-starvation promotes full-length transcription of these operons, which supports the level of coordinate transcription of functionally related genes and contributes to the efficacy of these systems under stressful conditions. When all effects are considered, the rshStr-independent response to BCAA limitation appears to counteract the stringent response and enables S. pyogenes dynamically to exploit the protein-rich niches of their host to cope with stasis states under nutritional stress conditions.
The results summarized above were generated before anything was known about CodY action in S pyogenes. BCAAs had been shown to activate the pleiotropic repressor CodY in Lactococcus lactis (Guédon, Serror, Ehrlich, Renault, & Delorme, 2001), as well as in Bacillus subtilis (Shivers & Sonenshein, 2004). The codY of S. pyogenes was subsequently inactivated in strain NZ131 (M49) and experiments were performed to find possible instances of the codY mutants mimicking rshStr-independent stimulation of transcription upon BCAA deprivation under a variety of culture conditions, such as growth media and growth phases (Malke, Steiner, McShan, & Ferretti, 2006; Malke & Ferretti, 2007). Given the broad regulatory connectivity of codY among the Firmicutes (Sonenshein, 2007), CodY action could be thought to involve both direct and indirect effects. Direct action was suggested by finding the 15-base pair CodY binding sequence, as defined in Lactococcus lactis (den Hengst, et al., 2005; Guédon, Sperandio, Pons, Ehrlich, & Renault, 2005) upstream of several NZ131 genes, including the opp operon, the braB gene, and the negatively autoregulated codY gene itself (Malke & Ferretti, 2007). These findings obtained in silico were substantiated by electrophoretic mobility shift assays (EMSAs), which provided direct evidence for the function of the codY box in S. pyogenes (Kreth, Chen, Ferretti, & Malke, 2011). However, how much degeneracy of the consensus sequence is tolerated for function is still not known, given that a putative CodY-binding site with 4 mismatches (including loss of the inverted repeat of the box) was identified in the mga promoter (Flores, et al., 2013).
Concerning the fact that high BCAA levels render CodY a more active repressor, a unique feature of the S. pyogenes homolog deserves further consideration. Northern analysis showed for two strains, NZ131 (M49) and SF370 (M1), that codY is transcribed both monocistronically from its own promoter and dicistronically from the promoter of the co-oriented upstream aat gene that encodes aspartate aminotransferase (Malke, Steiner, McShan, & Ferretti, 2006). This implies that monocistronic transcript levels are down regulated at low BCAA levels (namely, under nutritional stress conditions) and are counterbalanced by the BCAA-independent dicistronic mode of codY transcription, with the caveat that nothing is known about the control of aat transcription.
Using quantitative RT-PCR, (qPCR), a selected set of about 50 NZ131 (M49) genes was studied for differential expression under various conditions. This included regulators with known targets, established virulence factors, physiologically important transporters, and metabolic enzyme genes (Malke, Steiner, McShan, & Ferretti, 2006; Malke & Ferretti, 2007; Malke, McShan, & Ferretti, 2009). The results revealed that CodY and RshStr did not significantly affect the expression of each other; instead, they appeared to act independently, as indicated by an rshStr-codY2 double mutant that showed a composite transcription pattern of the gene sets for the single mutants (Malke, Steiner, McShan, & Ferretti, 2006). Additional support for an independent action of the two regulatory systems was provided by their additive effects in the double mutant regarding the expression patterns of braB and graB, which are already strongly affected in both single mutants under specific conditions (Malke, Steiner, McShan, & Ferretti, 2006). Overall, specific transcription units from all four groups previously mentioned were under CodY control, often in a medium and growth-phase dependent manner. In particular, growth in human blood apparently involves environmental cues that are absent in laboratory media, which leads to partially discordant CodY-directed transcription profiles. The impact on transcription regulators (covRS, fasX, mga, sptRS) amplifies the connectivity of CodY and provides bona fide evidence for the indirect CodY regulation of diverse sets of genes. Virulence genes (cfa, emm49, graB, hasA, ideS, nga, prtS, sagA, scl, scpA, ska, slo, sof, speH), were shown to be under the control of CodY, presumably because their direct regulators (mainly covRS and mga) are in the CodY regulon. Therefore, CodY tends to upregulate the positive regulator Mga and to down-regulate the negative regulator CovRS, which indirectly enhances the expression of a broad range of virulence factors. Notably, a number of these up regulated virulence factors (such as Cfa, Ids, Nga, PrtS, ScpA, and Slo) also serve to release nutrients from the host (Malke & Ferretti, 2007). Transporter gene expression (braB, dppA, malX, opp, pbuX, ptsG, pyrP) turned out to be highly specific to the individual systems, with braB and opp being most strongly affected in a cost-effective manner, as accounted for by the culture conditions.
Regarding the metabolic enzyme genes, CodY-mediated control of the arcABC operon involved in the catabolism of arginine is particularly relevant, because it serves to integrate basic nitrogen metabolic and virulence pathways by yielding ammonia and ATP, as well as leading to host-generated nitric oxide production. Ammonia production supports survival of the cells in acidic conditions, such as those found within phagolysosomes (Degnan, et al., 2000), and nitric oxide mediates host innate immunity (Cusumano, Watson, Jr., & Caparon, 2014). Experiments carried out in near-natural conditions, namely with NZ131 (M49) and its isogenic codY1 mutant cultured in heparinized human blood and sampled at different points during the course of growth, found that arcABC transcript levels up regulated in the codY1 mutant decrease dynamically during growth (Malke, McShan, & Ferretti, 2009) and imply that strong CodY repressor activity occurs preferentially during the first hours of exposure to blood, when effective BCAA concentrations are still available. This activity does not appear to be directly or indirectly influenced by CodY action on the arc operon regulators argR and crp (Malke, McShan, & Ferretti, 2009).
Interaction of the global CodY and CovRS regulatory systems
Given that CodY and CovRS show a broad overlap regarding their target genes, their regulons were dissected using NZ131 and its codY1, covRS and codY1-covRS mutants grown in C medium (Loughman & Caparon, 2006b) to mid-exponential (ME) and early stationary (ST) phase. In contrast to the nonpolar codY1, covRS disrupting the operon at codon 174 is polar and inactivates both covR and the downstream covS, which prevents the possible cross talk of CovS with other regulators (Steiner & Malke, 2000). Transcriptome analysis using DNA microarrays (Kreth, Chen, Ferretti, & Malke, 2011) showed that CodY controls the transcription of about 17% of the core genome (1,605 genes, excluding prophage), which is a finding comparable to the 15% controlled by CovRS (Graham, et al., 2002). Regarding the action of the two systems on virulence gene control, CovR-directed repression generally appears to be stronger than CodY-mediated activation. This can be seen in Figure 4 by looking at the phenotypes of the four strains, with respect to capsule production (hasABC).
The most intriguing results concerning the relationship between the actions of CodY and CovRS were observed upon robust correlation analysis of the DNA microarray results (Kreth, Chen, Ferretti, & Malke, 2011). This revealed a strong and highly significant negative correlation between the action of CodY and CovRS (r(1603) = -0.40; p<0.0001) in ME phase cells. The negative correlation is relieved in the double mutant, but stays slightly negative (r(1603) = -0.16; p<0.0001), which indicates that CovRS-directed repression overrides CodY-mediated gene activation. When ST phase cells were subjected to the same analysis, the negative correlations between the actions of the two systems ceased to operate. This was particularly evident under expression threshold parameters (Kreth, Chen, Ferretti, & Malke, 2011) where the low r values did not reach significance.
Therefore, CodY and CovRS appear to act in opposite directions, with the expression-balancing effect being largely restricted to actively growing cells. This leads to intermediate levels of not only virulence gene transcripts, but also lessens full transcriptional control of a substantial fraction of the whole genome. This could be due to CodY and Mga governing about 17% and 10%, of the genome, respectively (Hondorp & McIver, 2007; Kreth, Chen, Ferretti, & Malke, 2011). In light of the Red Queen principle, the interaction of CodY and CovRS may indicate that the host-pathogen association has coevolved to become less harmful to either partner.
Host environment responsive regulators
As has been indicated throughout this chapter, multiple transcriptional regulators of S. pyogenes influence their targets in a strain-specific manner (such as in RALPs (Kreikemeyer, Beckert, Braun-Kiewnick, & Podbielski, 2002)) or a tissue site-specific manner (such as in MtsR, Mga, or VicR). Here we describe the role of different S. pyogenes virulence-regulators in the context of the host environments and responses in which experimental data indicate that they are of the greatest relevance to colonization and survival.
Oxidative and Acid Stress
In addition to the proteins that co-opt host processes for the pathogen’s benefit or subvert host immune responses, virulence factors also include proteins that function to protect the bacterium from environmental stressors in the host, especially those resulting from mechanisms of host immunity. S. pyogenes produces a variety of proteins to sense and respond to oxidative and acid stresses, the chemical stressors primarily encountered within its human host, and both dedicated and general regulators tightly control expression of these proteins.
PerR
Oxidative stress is the product of exposure to reactive oxygen species (ROS) that are generated from atmospheric oxygen or produced by a phagocyte oxidative burst. These include superoxide anions (O2-), hydrogen peroxide (H2O2), and highly toxic hydroxyl radicals (OH-), all of which can damage nucleic acids, proteins, and cell membranes. Like other lactic acid bacteria, S. pyogenes can also produce peroxide endogenously as a byproduct of carbohydrate metabolism (Gibson & Caparon, 1996) and consequently can generate hydroxyl radicals in an iron-rich environment as a result of the Fenton reaction (Tsou, et al., 2008). As a result, bacteria have evolved distinct inducible responses to handle peroxide and superoxide stress (Tsou, et al., 2008; Farr & Kogoma, 1991).
Superoxide dismutase (SodA) mitigates superoxide stress by converting O2- into H2O2 and O2 (Fridovich, 1997; Gerlach, Reichardt, & Vettermann, 1998), and a critical role for SodA in the aerobic growth of S. pyogenes has been proposed (Gibson & Caparon, 1996). However, S. pyogenes does not encode catalase, so peroxidases like alkyl hydroperoxidase/ alkyl hydroperoxide reductase (ahpCF), and glutathione peroxidase (gpoA) act to neutralize endogenous and exogenous peroxides, which contribute to its aerotolerance and ROS detoxification in vitro (Brenot, King, & Caparon, 2005; King, Horenstein, & Caparon, 2000). Iron chelating proteins required for iron homeostasis, such as the iron/ DNA-binding protein Dpr/MrgA (Dps-like peroxide resistance protein), have also been shown to contribute to virulence through their involvement in resistance against oxidative DNA damage (Tsou, et al., 2008; Brenot, King, & Caparon, 2005); by chelating free intracellular Fe2+, Dpr/MrgA prevents its reaction with peroxide (Fenton reaction), which minimizes the production of highly reactive hydroxyl radicals. However, the individual contributions of these factors to bacterial fitness in vivo is only moderate, as revealed by subcutaneous and intraperitoneal infection of mice with ahpC- and gpoA-deficient strains (Brenot, King, & Caparon, 2005; Brenot, King, Janowiak, Griffith, & Caparon, 2004). As indicated above, control of iron metabolism is also part of the oxidative stress response in S. pyogenes, since iron is both an essential cofactor and, when unregulated, a hazardous metal that participates in the production of ROS (Ricci, Janulczyk, & Björck, 2002; Tsou, et al., 2008).
Control of the peroxide stress response in S. pyogenes occurs mainly through the transcriptional regulator PerR, a homolog of the H2O2- and metal ion-responsive ferric uptake regulator (Fur) and other Fur-like proteins found in Bacillus subtilis and Staphylococcus aureus (Bsat, Herbig, Casillas-Martinez, Setlow, & Helmann, 1998; Horsburgh, Clements, Crossley, Ingham, & Foster, 2001). PerR was first identified in S. pyogenes as required for an inducible peroxide resistance response (King, Horenstein, & Caparon, 2000). Characterization of a PerR-deficient AP1 (M1) strain in which the DNA- and metal-binding domains of perR were deleted revealed that PerR influences iron metabolism and acts as a repressor of the peroxide response (Ricci, Janulczyk, & Björck, 2002). Further analysis revealed that the loss of PerR expression results in highly attenuated virulence in both subcutaneous and intraperitoneal models of infection (Brenot, King, & Caparon, 2005), is associated with reduced resistance to phagocytic killing in human blood and by murine macrophages in vitro, and produces severely attenuated virulence in a baboon model of S. pyogenes pharyngitis (Gryllos, et al., 2008b). PerR-dependent gene expression appears to be linked to resistance to the phagocyte oxidative burst, as increased phagocytic killing of a perR-deficient strain was abrogated in the presence of diphenyleneiodonium chloride (DPI), a general oxidative burst inhibitor (Gryllos, et al., 2008b).
Dissection of the PerR regulon of S. pyogenes initially yielded confounding results. Unlike the PerR protein of Bacillus subtilis that up-regulates the expression of genes that encode peroxidases (e.g. ahpC) (Helmann, et al., 2003; Mostertz, Scharf, Hecker, & Homuth, 2004), some peroxide responsive transcripts in S. pyogenes were directly repressed by PerR via binding of their promoter regions (e.g. mrgA) (Brenot, King, & Caparon, 2005). In contrast, other genes (e.g. ahpCF, sodA) were simultaneously expressed in both a PerR-dependent (Gryllos, et al., 2008b) and PerR-independent (Grifantini, Toukoki, Colaprico, & Gryllos, 2011) manner. Additional transcripts repressed in a PerR- and/or peroxide-dependent manner included genes involved in carbohydrate metabolism (such as the Lac. 2 operon), DNA metabolism (such as purine synthesis loci purM, purN, and purE), metal homeostasis (such as siaABC, mtsABC, and pmtA), and amino acid/peptide transport (such as the oligopeptide permease operon, oppABCDF) (Grifantini, Toukoki, Colaprico, & Gryllos, 2011; Gryllos, et al., 2008b). The reason for these seemingly contradictory results is the fact that the PerR-dependent expression was examined by comparing the transcriptomes controlled by PerR in peroxide (Grifantini, Toukoki, Colaprico, & Gryllos, 2011) with those identified by comparison of wild-type and perR-deficient transcriptomes in the absence of stress (Gryllos, et al., 2008b), which suggests that the oxidative state of the cell influences the extent of the PerR regulon. This was further suggested by an analysis of metal binding in PerR activity, which revealed that PerR transcriptional regulation is dependent on iron for maximal sensitivity to peroxide (Grifantini, Toukoki, Colaprico, & Gryllos, 2011). Characterization of the crystal structure of PerR combined with mutational analysis demonstrated that metal binding by PerR occurs at a structural site and a distinct regulatory site, the latter of which is critical to S. pyogenes virulence (Makthal, et al., 2013). Metal binding at the regulatory site is not required for DNA binding per se; rather, it promotes higher affinity PerR/DNA interactions and PerR conjugated with iron (as opposed to manganese) at this site responds to peroxide stress directly and dissociates from operator sequences.
Given that PerR does not upregulate any known peroxidase(s) or MrgA, and that PerR represses DNA and protein metabolism as well as iron/heme uptake, a mechanism was proposed for the existence of dual PerR regulons (Grifantini, Toukoki, Colaprico, & Gryllos, 2011). In this model, a transient decrease in gene expression and decreased metabolic function upon oxidative challenge would allow for DNA and protein damage repair before normal transcription resumes. Reduced iron/heme uptake (from siaABC, mtsABC repression) coupled to increased Mn2+ import, as indicated by observed PerR-dependent up regulation of the adc operon (Grifantini, Toukoki, Colaprico, & Gryllos, 2011), would lead to the replacement of Fe2+ as an enzymatic cofactor with the Fenton-insensitive Mn2+, thereby permitting optimal bacterial metabolism in oxidative environments. This would also translate into increased levels of Mn2+-bound PerR and an ensuing gradual decrease in transcriptional responses, initially driven by the oxidation of Fe2+-bound PerR species in the presence of peroxide. As a result of PerR presenting different modes of metal-dependent DNA binding, PerR appears to control two different regulons in both the presence and absence of oxidative stress (Grifantini, Toukoki, Colaprico, & Gryllos, 2011; Gryllos, et al., 2008b; Makthal, et al., 2013).
Data further elucidating how PerR-mediated metal homeostasis and oxidative stress resistance influences S. pyogenes virulence has been provided by investigation of the pmtA-encoded metal transporter (VanderWal, et al., 2017). PerR is required for induction of pmtA expression in response to excess iron, a response involved in tolerance to iron toxicity and oxidative stress, which in turn is critical to S. pyogenes survival in mouse models of invasive infection. The induction of pmtA expression is dependent on the metallation state of PerR (Fe2+ vs Mn2+) and RNAseq analysis revealed pmtA-dependent global changes in S. pyogenes gene expression in the course of adaptation to iron toxicity (VanderWal, et al., 2017). These data suggest that PerR-dependent regulation of PmtA-mediated iron homeostasis is central to S. pyogenes antioxidant defenses that contribute to S. pyogenes virulence.
CiaH/R
Recent mutational analysis of the sensor proteins of TCSs in S. pyogenes uncovered the involvement of the CiaH sensor kinase in acid and oxidative stress (Tatsuno, Isaka, Okada, Zhang, & Hasegawa, 2014). A ciaH-deficient M1 clinical isolate (1529) was significantly sensitive to hydrogen peroxide and displayed reduced survival when grown in acidic media (pH 6.0) in an atmosphere with 5% CO2. Though full characterization of the CiaH/R regulon is pending, an interesting result of this study was the observation that loss of ciaH under the conditions tested resulted in decreased expression of nrdR, a regulator of ribonucleotide reductase genes, and polA1, a putative DNA polymerase I previously reported to contribute to peroxide stress defenses in S. pyogenes (Tatsuno, Isaka, Okada, Zhang, & Hasegawa, 2014; Toukoki & Gryllos, 2013). A prior study examining CiaH/R activity in an M49 serotype strain found that the loss of ciaH expression resulted in the increased transcription of multiple divalent cation transporters and six transcripts of genes generally involved in stress response or genetic competence (arcA, csp, dnaK, epuA, hsp33, and recA), while 11 members of the PTS and four genes that encode ribosomal proteins showed decreased transcript amounts, with maximal expression of CiaHR occurring during the transition phase of growth (Riani, et al., 2007). CiaH was also implicated in the differential gene expression associated with SpeA superantigen-mediated biofilm dispersal (Babbar, Barrantes, Pieper, & Itzek, 2019). Among virulence related genes of this strain background, the expression of hemolysin (hlyX), hyaluronidase (hylA), and protein F2 (prtf2) were increased, while sfbX and DNase (mf2) transcripts were decreased in the ciaH mutant. Nonetheless, characterization of the CiaH/R regulon in vivo or under stress conditions remains to be performed.
Growth phase/quorum sensing-mediated virulence gene control (Rgg/RopB, Rgg2/3, Sil, TrxSR, PepO)
A theme running through this chapter is that S. pyogenes continuously senses the conditions of its surrounding environment and simultaneously regulates the expression of its genomes to maintain and enhance its survival. Batch culture is the simplest method to investigate such bacterial adaptation to the depletion of sources of energy and resources for growth, as well as the accumulation of metabolic end products. S. pyogenes exhibits two primary growth phases in culture: exponential and stationary. The former is characterized by preferential use of glucose as the source of energy that is fermented to lactic acid, continuous cell division, expansion of the bacterial population and the expression of a regulatory program corresponding to the prevailing metabolic status of the cell (Chaussee, Dmitriev, Callegari, & Chaussee, 2008). The stationary phase of growth is evidenced by the depletion of glucose, acidification of the media, the arrest of cell division, and population growth and transition into a radically different regulatory program. Examination of the growth phase-dependent changes in gene expression in S. pyogenes has revealed that, unlike other bacteria that employ a variety of alternative sigma factors to modulate gene expression in response to changes in the environment (Helmann & Moran, Jr., 2001; Beyer-Sehlmeyer, Kreikemeyer, Hörster, & Podbielski, 2005), S. pyogenes alters the growth phase-associated patterns of gene expression through interactions among transcriptional regulators. Therefore, growth phase-dependent regulation of S. pyogenes virulence involves global regulators (such as CovR/S or RALPs), metabolite responsive regulators (namely, CodY and Mga) and dedicated regulators that have been observed to have genome-wide effects in response to changing growth phases.
One such dedicated virulence regulator, which was first identified by its influence on growth-phase dependent SpeB production, is the Rgg-family transcriptional regulator Rgg/RopB, which induces maximal expression of the extracellular SpeB protease (Chaussee, Ajdic, & Ferretti, 1999; Lyon, Gibson, & Caparon, 1998) during stationary phase growth (Unnikrishnan, Cohen, & Sriskandan, 1999). Rgg/RopB is thus a central component of the cell density-associated regulation of SpeB expression. The SpeB-inducing peptide (SIP) intercellular signaling system employs an eight-amino acid leaderless peptide to influence global gene expression through Rgg/RopB (Do, et al., 2017). S. pyogenes produce SIP, encoded in the intergenic region between ropB and speB, during growth at high cell density. The cell-to-cell SIP signaling pathway involves synthesis and secretion of SIP and reimportation of the peptide into the cytosol, followed by SIP interaction with Rgg/RopB, which in turn results in SIP-dependent modulation of RopB/DNA interaction and regulatory activity that terminates in RopB-mediated induction of speB expression (Do, et al., 2017). SIP signaling appears to be functional during S. pyogenes growth in human saliva and blood, and SIP-mediated speB expression contributes to S. pyogenes colonization of the mouse oropharynx, as well as survival in human blood (Makthal, et al., 2018).
Investigation of the mechanism by which SIP signaling influences RopB-mediated induction of SpeB expression revealed a pH-sensing mechanism involving a pH-sensitive histidine switch of the SIP-binding pocket of RopB (Do, et al., 2019). Environmental acidification induces protonation of the His144 residue and reorganization of hydrogen bonding networks in RopB to enhance SIP recognition. Proteomic and qPCR approaches to analyze the influence of Rgg/RopB on the expression of other extracellular proteins in a SpeB-deficient NZ131 (M49) strain found that, during the stationary phase of growth, the loss of Rgg/RopB expression led to a decreased transcription of genes that encode lysozyme, autolysin and ClpB (a heat shock protein), but produced more streptodornase (DNase encoded by sdb/mf) and a DNA entry nuclease (Chaussee, Watson, Smoot, & Musser, 2001). As described in an earlier section, transcriptome analysis using DNA microarrays revealed that Rgg/RopB affects the stationary-phase expression of amino acid metabolic genes. Further characterization of rgg inactivation in the NZ131 (M49) strain found Rgg/RopB also controls amino acid and non-glucose carbohydrate metabolism genes (Chaussee, Somerville, Reitzer, & Musser, 2003; Chaussee, Callegari, & Chaussee, 2004; Dmitriev, et al., 2006). These studies also uncovered that transcripts that encode virulence factors involved in cytolysin-mediated translocation of NAD-glycohydrolase (spn), including the immunity factor (ifs) and streptolysin O (slo), were more abundant in the rgg-deficient strain, which correlated to increased levels of NADase (SPN) and SLO activity in both exponential and stationary growth phases (Dmitriev, et al., 2006). These data suggested that Rgg/RopB both directly and indirectly exerts growth-phase dependent control of virulence proteins and that it represses the transcription of spn, ifs, and slo during the exponential phase of growth, as well as controlling the degradation of the secreted proteins during the stationary phase by inducing the expression of the SpeB protease. More recent transcriptomic analyses report differential expression of ∼14% of the S. pyogenes genome, including upregulation of virulence factors (e.g. Spi, SagP, NdoS, and Sdn streptodornase) that promote pathogen dissemination and infection. Do et al. demonstrated that SIP system signaling and RopB-mediated induction of SpeB is required for S. pyogenes virulence in various murine infection models (Do, et al., 2017) and is conserved across various S. pyogenes serotypes (Makthal, et al., 2018).
Growth-phase–dependent changes in gene expression in S. pyogenes involve connections between Rgg and more globally acting regulatory networks. The inactivation of rgg in NZ131 (M49) increases the transcription of several virulence-associated genes, including speH (which encodes a superantigen), scl1/sclA (which encodes an adhesion protein), ska, hasABC, mf, mf3, grab, and mac, in addition to targets of the Mga regulon (emm, scpA, sagA, and slo) (Chaussee, et al., 2002). The altered expression of these virulence genes correlates with changes in the transcription of several regulatory genes, including mga, covR/S, and fasBCAX; indicating that Rgg/RopB influences other global regulators to exert its genome-wide modulation of virulence. Further evidence of this is in the increased transcription of the TCS genes lytR and lytS in an rgg-deficient strain during the exponential growth phase; in the stationary phase of growth, the transcripts of FasB, CpsY, and the RALP4 (RivR) regulator were less abundant in the mutant strain (Dmitriev, McDowell, Kappeler, Chaussee, Rieck, & Chaussee, 2006). However, inter-serotype and intra-serotype variations are a common feature of the Rgg/RopB regulon. The sequencing of rgg/ropB in 171 invasive serotype M3 strains identified 19 distinct alleles (Carroll, et al., 2011). The observed rgg/ropB polymorphisms alter regulator function, as inactivation of the gene in strains producing distinct Rgg/RopB variants had dramatically divergent effects on global gene expression. Iso-allelic S. pyogenes strains that differed by as little as a single amino acid in Rgg/RopB all exhibited differing transcript levels of speB. Comparison of parental, rgg/ropB-inactivated, and rgg/ropB iso-allelic strains in mouse infection models manifested differences in virulence and disease manifestations (Carroll, et al., 2011). Additionally, rgg-deficient mutants in three different strains, which represented M1 (SF370 and MGAS5005) and M49 (CS101) serotypes, all exhibited different changes in gene expression in the stationary phase of growth (Dmitriev, McDowell, & Chaussee, 2008). Specifically, the inactivation of rgg alters transcription of regulatory genes in SF370 and CS101 strains, but not in MGAS5005. These studies identified speB and an adjacent hypothetical gene (spy2040; later precisely defined as the SIP coding region (Do, et al., 2017) as the only transcripts similarly affected by rgg inactivation in all three strains. Nevertheless, a strain-specific Rgg/RopB subregulon was also detected, which could vary by as little as a single gene (nrdD) in the MGAS5005 strain, and by as much as 43 genes in SF370 (Dmitriev, McDowell, & Chaussee, 2008).
Given such variation among strains for Rgg/RopB-dependent gene regulation, it was important to define the mechanism of Rgg/RopB-mediated control of transcription in S. pyogenes. Chromatin immunoprecipitation followed by DNA microarray (ChIP-chip) analysis identified 65 DNA binding sites for Rgg in the NZ131 chromosome: 35 of them within noncoding DNA and 43% of which were adjacent to genes previously identified as regulated by Rgg (Anbalagan, McShan, Dunman, & Chaussee, 2011). EMSA demonstrated Rgg binding to noncoding DNA upstream of speB, and the genes that encode PulA, Spd-3, a predicted transcriptional regulator (Spy49_1113), prophage-associated genes that encode a putative integrase (Spy49_0746) and a surface antigen (Spy49_0396), both in vivo and in vitro. Rgg-mediated transcriptional regulation of these genes via an active promoter was confirmed using a luciferase reporter system (Anbalagan, McShan, Dunman, & Chaussee, 2011). Further characterization of Rgg-mediated promoter activation found that Rgg is unable to bind to the speB promoter to induce transcription when bound to LacD.1 during exponential growth, but as levels of glycolytic intermediates decrease upon entry into stationary phase, a change in LacD.1 conformation results in dissociation from Rgg and activation of SpeB expression (Loughman & Caparon, 2006a). This LacD.1-dependent effect on the DNA binding specificity of Rgg in response to changes in environmental carbohydrates was not limited to SpeB production, as demonstrated for emm49, sof, sfbX49, and speH genes in NZ131 (M49) using in vitro DNA-binding and in vivo transcriptional fusion assays (Anbalagan, Dmitriev, McShan, Dunman, & Chaussee, 2012). These results further support the paradigm that growth-phase–dependent gene modulation in S. pyogenes is based on the interactions of multiple transcriptional regulators, which vary greatly among strains, and which necessitates further examination of the manner in which regulatory networks are structured in different serotypes in order to understand strain differences and similarities when infecting a host.
Rgg2/3, PepO
Recent research into transcriptional regulation by Rgg homologs in streptococci suggests that Rgg-family regulators also serve as cytoplasmic receptors for intercellular signaling peptides that resemble the quorum-sensing pathways of other Gram-positive bacteria (Fontaine, et al., 2010; Ibrahim, et al., 2007a; Mashburn-Warren, Morrison, & Federle, 2010). Signaling peptides, which are commonly called autoinducers or pheromones, are detected either extracellularly by transmembrane sensor kinases, or intracellularly by import to the cytoplasm, where they engage a signal transduction pathway or a transcription factor to influence gene regulation (reviewed in (Cook & Federle, 2014)). Such signaling peptide-based intercellular communication (such as quorum sensing) among Firmicutes is an established paradigm, according to which, ribosome-dependent polypeptides are produced as inactive pro-peptides, are secreted from the cell by the general secretory (Sec) system or through designated ABC transporters, and are processed into active signaling molecules by a variety of proteases (Cook & Federle, 2014). The newly-defined activity for Rgg proteins in streptococci as quorum sensing receptors comes from experimental analysis of genes that encode Rgg members and adjacent open reading frames (ORFs) that encode short, secreted peptides, as exemplified by the S. mutans and S. thermophilus ComR protein, which, together with the short peptide ComS, positively regulates competence development (Ibrahim, et al., 2007a; Mashburn-Warren, Morrison, & Federle, 2010). Including Rgg/RopB, S. pyogenes has four identifiable genes encoding Rgg-family proteins: comR (spy49_0032), rgg/ropB (spy49_1691), rgg2 (spy49_0415), and rgg3 (spy49_0449c) (Chang, LaSarre, Jimenez, Aggarwal, & Federle, 2011). Additionally, the analysis of unannotated small ORFs throughout Gram-positive genomes identified ORFs coding for short hydrophobic peptides (shp) adjacent to both rgg2 and rgg3 in every sequenced S. pyogenes genome (Ibrahim, et al., 2007b). The shp genes were shown to encode 22 and 23 amino acid peptides that are processed to mature pheromones, that serve to induce expression of the related neighboring genetic loci (i.e. rgg2/3), and that positively regulate their own transcription in a NZ131 (M49) strain (Chang, LaSarre, Jimenez, Aggarwal, & Federle, 2011). As mentioned above, the expression of the shp genes promotes biofilm biogenesis in S. pyogenes, an activity mediated by the regulatory activity of Rgg2 and Rgg3. The function of the peptides depends on a trans-membrane peptidase (Eep) for processing, and the oligopeptide permease (Opp) for importation (Chang, LaSarre, Jimenez, Aggarwal, & Federle, 2011), as previously observed in other Gram-positive bacteria (Solomon, Su, Shyn, & Grossman, 2003; Doeven, Abele, Tampé, & Poolman, 2004).
Though Rgg2/3 lack recognizable primary-sequence similarity to any other quorum-sensing components, structural prediction algorithms reveal a potentially similar secondary and tertiary structure to PlcR and PrgX, two prototypical members of the RNPP protein family found throughout Gram-positive bacteria that serve as receptors for imported signaling peptides (Federle, 2012). Rgg2 and Rgg3 each control the transcription of the promoters driving that drive both shp genes, but do so with antagonistic activities. In the absence of pheromones, Rgg3 binds to DNA at both shp promoters and represses their transcription; but when pheromones are present, Rgg3 releases DNA and transcription initiates (Chang, LaSarre, Jimenez, Aggarwal, & Federle, 2011). Rgg2 appears to act only as a transcriptional activator when the shp-encoded pheromones are transported into the cell. Regulation of the peptide-responsive promoters by the antagonist Rgg2 and Rgg3 transcriptional regulators involves competition for highly conserved, shared binding sites located proximal to the −35 nucleotide in the target promoters, which results in concentration-dependent, exclusive occupation of the binding site that can be skewed in favor of Rgg2 in vitro by the presence of the pheromones (Lasarre, Aggarwal, & Federle, 2013). Therefore, the net response to pheromones is to induce shp and rgg2/3 promoter expression, which results in amplification through a positive feedback loop.
CovRS influences signaling by the Rgg2/3 quorum sensing pathway via PepO, a neprilysin-like metallo-endopeptidase that is capable of preferentially degrading SHP2 over SHP3 pheromone (Wilkening, Chang, & Federle, 2016; Pérez Morales, et al., 2018). Inactivating mutations in CovR were shown to disrupt induction of the Rgg2/3 pathway, a phenotype reverted by inactivation of PepO expression and peptidase activity (Wilkening, Chang, & Federle, 2016). The data generated by Wilkening et al suggests a regulatory model in which repression of pepO expression by the CovRS system prolongs stability of shp-encoded pheromones, whereas de-repression of pepO expression enhances PepO-mediated SHP degradation (Wilkening, Chang, & Federle, 2016). PepO has also been associated with growth phase-dependent regulation of speB expression (Brouwer, et al., 2018). Loss of PepO in M1 serotype S. pyogenes results in enhanced SpeB production during exponential phase growth in a CovRS-dependent manner, as indicated by the enhanced expression of pepO and decreased expression of speB observed in a the CovS-null 5448AP background (Brouwer, et al., 2018). These findings provide further evidence that the Rgg family of transcriptional regulators function as quorum-sensing effector proteins and comprise the first functional quorum-sensing pathway conserved in S. pyogenes.
Sil
The streptococcal invasion locus, or Sil, is a bacteriocin-like peptide-based quorum sensing system identified in approximately 25% of S. pyogenes strains (Michael-Gayego, Dan-Goor, Jaffe, Hidalgo-Grass, & Moses, 2013). This locus was originally identified by a transposon insertion into the silC gene of the JS95 (M14) strain that resulted in attenuated virulence in a mouse model of necrotizing fasciitis. Sil is composed of genes encoding a TCS (silAB), an ABC transporter (silDE) and the silC/silCR locus (Hidalgo-Grass, et al., 2002). The silCR locus, which overlaps a majority of the silC gene but is transcribed from the reverse strand, encodes the peptide pheromone silCR. The SilAB and silDE/CR polypeptides appear to be co-transcribed from two promoters, termed P1 and P3 respectively, with the latter induced upon addition of SilCR in a SilA-dependent fashion, which controls several putative bacteriocin-related genes (Eran, et al., 2007; Belotserkovsky, et al., 2009). As indicated before, the Sil system is not present or functional in a majority of S. pyogenes strains. SilC is not found in M1 or M3 serotype strains; the ATG start codon has been mutated in M14 strains and the putative pheromone transporter SilD is truncated in M18 strains (Hidalgo-Grass, et al., 2004). Though SilCR was originally reported to limit infection by promoting neutrophil-mediated clearance and preventing systemic spread in mice inoculated with an M14 strain (Hidalgo-Grass, et al., 2004), a conflicting study later found that the addition of the SilCR peptide induced expression of scpC, sagA, and siaA in vitro and of the latter two genes in vivo in an M1 strain, which resulted in impaired lesion healing in infected mice (Salim, de Azavedo, Bast, & Cvitkovich, 2008). These conflicting results and the paucity of S. pyogenes strains with an active Sil indicate that its function in virulence may be either strain- or serotype-specific.
TrxSR
Another transcriptional regulator recently linked to quorum sensing in S. pyogenes is the TCS encoded by the trxSR operon. Originally studied due to its homology to a virulence-related TCS in S. pneumoniae, an insertional mutation in the response regulator gene, trxR, in a MGAS5005 (M1T1) strain led to a significant reduction in lesion size, lesion severity, and lethality in a murine skin infection model (Leday, et al., 2008). Examination of TrxR activity showed that CovR directly represses the trxR promoter in vitro and TrxR induces transcription of Mga-regulated virulence genes by activation of Pmga, as demonstrated by analysis of a luciferase reporter fusion. Complementation of the trxR-deficient MGAS5005 mutant restored expression of Mga regulon genes and restored virulence in the mouse model to wild type levels (Leday, et al., 2008). Investigation of Sil activity during adherence to host cells uncovered that secretion of Slo and Sls produces endoplasmic reticulum stress, which in turn results in increased asparagine (ASN) synthetase expression and production of ASN (Baruch, et al., 2014). Sensing of the released ASN by both a JS95 (M14) and a MGAS5005 (M1) strain alters the expression of ~17% of S. pyogenes genes, of which about one-third are regulated by TrxSR. Given that MGAS5005 lack an active Sil, the induced expression of the streptolysin toxins and the down-regulation of proliferation-related transcripts in the absence of ASN was determined to be TrxSR-dependent (Baruch, et al., 2014). These results were further confirmed by the loss of ASN-mediated activation of Sil in a trxR-deficient JS95 strain, the loss of ASN-mediated regulation of TrxSR in a trxR-deficient MGAS5005 strain, and the observation that treatment with asparaginase both arrests S. pyogenes growth in human blood and blocks proliferation in a mouse model of human bacteremia. Therefore, TrxSR appears to constitute a quorum sensing-like system in S. pyogenes, which regulates gene expression in response to sensed ASN produced by host cells under stress. Recent investigation of TrxSR-related phenotypes found that a ∆trxS mutant in the M1 serotype strain SF370 failed to produce biofilms or activate the mga promoter in an acidified environment (Isaka, et al., 2021). Accordingly, M protein production was altered in the TrxS-lacking mutant, which is consistent with the transcriptional regulation of emm.
Other quorum sensing mechanisms (SpoV, Acyl homoserine lactones)
Another peptide involved in intercellular communication that influences gene expression in response environmental stressors is SpoV (Streptococcal peptide controlling Virulence), a secreted peptide encoded near the gene encoding Streptolysin O (slo) (Herrera, Callegari, & Chaussee, 2021). Exposure to synthetic SpoV peptide derivatives enhanced slo transcript abundance in an M49 isolate, but not in an M3 isolate. Conversely, deletion of the spoV gene (∆spoV) decreased slo transcript levels in both M49 and M3 background. The M3 ∆spoV mutant also exhibited reduced extracellular SLO protein levels, SLO-specific hemolytic activity and was deficient in the ability to survive in murine blood. Pre-incubation of the ∆spoV mutant with synthetic SpoV peptide derivatives promoted S. pyogenes survival (Herrera, Callegari, & Chaussee, 2021).
Acyl homoserine lactones (AHLs) have been described as the quorum-sensing molecules of the canonical LuxI/R quorum sensing system in Gram negative bacteria (Plančak, Musić, & Puhar, 2015). A study that investigated the contribution of AHL activity to virulence of an M6 serotype S. pyogenes strain determined that AHLs with fatty acid side chains ≥12 carbon atoms induced expression of the LuxR transcriptional regulator and inhibited expression of the sag operon (Saroj, Holmer, Berengueras, & Jonsson, 2017). LuxR was shown to interact with a region upstream of the sagA gene and that bactericidal activity of AHLs in S. pyogenes at millimolar concentrations involved the ferrichrome transporter FtsABCD, which is responsible for AHL transport into the streptococcal cell (Saroj, Holmer, Berengueras, & Jonsson, 2017).
FasBCA/X
The fibronectin/fibrinogen binding/hemolytic activity/streptokinase (Fas) regulator of S. pyogenes was identified in the M49 serotype strain, based on homologies to the histidine protein kinase (HPK) and response regulator components of Staphylococcus aureus (Agr) and S. pneumoniae (Com) quorum-sensing systems (Kreikemeyer, Boyle, Buttaro, Heinemann, & Podbielski, 2001). Despite their homology to the Com system, autoinducing peptides thus far detected in culture supernatants do not function with the Fas regulator (Chang, LaSarre, Jimenez, Aggarwal, & Federle, 2011; Belotserkovsky, et al., 2009), which makes this unlikely to be another quorum-sensing system. The fasBCA operon is found in all tested M serotypes and is transcribed as a polycystronic message that expresses two predicted HPKs (FasB and FasC) and one response regulator (FasA). Insertional mutagenesis that disrupts fasBCA and fasA revealed that the expression of the Fas operon down-regulates transcription of genes that encode S. pyogenes adhesins (fbp54, mrp) and concomitantly induces the expression of genes that encode secreted virulence factors (sagA and ska) in a growth-phase-dependent manner (Kreikemeyer, Boyle, Buttaro, Heinemann, & Podbielski, 2001). A FasA-dependent 300 nucleotide monocistronic transcript that does not encode a peptide sequence, designated fasX, was also identified downstream of fasBCA. Deletion mutagenesis of fasX resulted in a similar phenotype to that of the fasBCA or fasA insertional mutations, whereas complementation in trans of the fasX deletion restored the wild-type fasBCA regulation, which suggests that fasX, encodes a putative non-translated RNA that is the main effector molecule of the Fas regulon (Kreikemeyer, Boyle, Buttaro, Heinemann, & Podbielski, 2001). Two virulence factor-encoding mRNAs have been confirmed as targets of FasX regulation, with the first being streptokinase (ska), which FasX base-pairs to at the first nine nucleotides of the ska mRNA in an interaction that increases the stability of the mRNA, and which results in a 10-fold increase in ska transcript abundance and streptokinase protein expression (Ramirez-Peña, Treviño, Liu, Perez, & Sumby, 2010). FasX must remain bound to its mRNA target to enhance stability, and the reversible nature of the FasX:ska mRNA interaction implies that that induction by FasX can be attenuated by decreasing FasX transcription or increasing FasX turnover. The other confirmed FasX-regulated virulence factor is the first gene in the pilus biosynthesis operon, cpa, which encodes the collagen binding minor pilus protein located at the pilus tip (Liu, Treviño, Ramirez-Peña, & Sumby, 2012). FasX base pairs to 16 of the first 17 nucleotides of cpa mRNA in order to repress pilus biosynthesis gene expression by inhibiting cpa mRNA translation and reducing the access of ribosomes to the cpa mRNA ribosome binding site. Such opposing regulatory activity on adhesins and streptokinase, a known agent of S. pyogenes dissemination, suggests that FasX is a virulence regulator that modulates the transition from localized colonization to systemic spread (Miller, Cao, Pflughoeft, & Sumby, 2014). Further evidence of this is the observation that complementation of a hypervirulent M3 strain to correct a naturally occurring mutation in fasC significantly reversed the streptokinase activity characteristic of the strain by enhancing the levels of FasX and increasing ska transcription (Cao, et al., 2014).
Comparison of fasX-lacking and complemented derivatives of serotype M1, M2, M6 and M28 serotype strains revealed that FasX reduces expression of pili encoded in the S. pyogenes fibronectin, collagen, and T-antigen (FCT) genomic region (Danger, et al., 2015). FasX bound and inhibited the translation of different FCT-region mRNAs in each serotype. FasX enhanced ska expression in each serotype to different degrees, and likewise enhanced S. pyogenes virulence in a human plasminogen-expressing murine model of bacteremia (Danger, et al., 2015). Further research revealed that FasX also downregulates expression of the adhesion- and internalization-promoting, fibronectin-binding proteins PrtF1 and PrtF2 (Danger, Makthal, Kumaraswami, & Sumby, 2015). FasX post-transcriptionally regulates PrtF1/2 expression by base pairing to the prtF1 and prtF2 mRNAs at the mRNA ribosome-binding sites within their 5' untranslated regions. FasX/prtF mRNA duplex formation blocks ribosome access, which results in inhibition of mRNA translation (Danger, Makthal, Kumaraswami, & Sumby, 2015).
Saliva
Multiple studies have examined virulence factor expression and transcriptional changes of S. pyogenes in body fluids, including saliva (Graham, et al., 2005; Shelburne, et al., 2005a; Shelburne, et al., 2005b). An MGAS5005 strain exposed to human saliva produces a variety of factors in a growth-phase dependent manner, including Sic, SpeB, streptococcal pyrogenic exotoxin A, Mac, streptococcal phospholipase A2, the lantibiotic salivaricin A (SalA), DNases, streptolysin S, and the 67-kDa myosin cross-reactive protein (spy0470) (Shelburne, et al., 2005a; Shelburne, et al., 2005b). Transcriptome profiling during exponential and stationary growth phases in blood identified a two component regulatory system, termed SptR/S, as essential to the persistence of S. pyogenes in human saliva (Shelburne, et al., 2005a). SptR/S acts as a transcriptional inducer of numerous virulence genes, including spd, spd3, sic, hasA, and speB, as well as genes that encode complex carbohydrate transporters and utilization enzymes. During exponential growth in saliva transcripts involved in pathogen-host interactions (such as emm and sic), oxidative stress response (e.g., ahpC and mtsA), ATP generation and pH balancing (such as the arginine deiminase operon) are up regulated, in accordance with the nutritional limitations and the low pH found in saliva; whereas in the stationary growth phase, starch-degrading and carbohydrate-metabolism genes are differentially regulated, either positively (malX, malE, amyA, agaD) or negatively (pulA, malE) (Shelburne, et al., 2005a). Additional virulence regulators observed to display activity in the saliva environment include RofA and several stress-response regulators (PerR, HrcA, Spy0583 and Ihk/Irr), which are all up regulated. Additional studies that examined the role of other virulence regulators found that CcpA and CovR influence gene expression in response to saliva, as it was observed that a ccpA-deficient MGAS2221 (M1) strain failed to induce the expression of speB, sagA, and arcA upon exposure to saliva and that a covR-deficient mutant in the same background exhibited reduced induction of these genes, as well as spyCEP, slo, and amyA, when grown in human saliva (Shelburne, et al., 2010).
Salivaricin A is one of the S. pyogenes factors induced in the presence of saliva. The salivaricin (sal) locus of S. pyogenes is ∼85% homologous to the sal locus of Streptococcus salivarius, the commensal bacterium in which SalA was originally identified. Paradoxically, only M4 serotype strains produce active SalA and are immune to the lantibiotic produced by S. salivarius, yet the sal locus is highly conserved (>92% homology) among sequenced S. pyogenes serotypes (Phelps & Neely, 2005; Upton, Tagg, Wescombe, & Jenkinson, 2001), suggesting an alternative function for the sal operon in S. pyogenes pathogenesis. In general, lantibiotic loci are transcribed as operons. In addition to the pre-lantibiotic peptide, these operons encode modifying enzymes to produce the mature lantibiotic, an immunity protein or specialized ABC transporter to provide resistance to the lantibiotic and a TCS to regulate expression of the operon (Willey & van der Donk, 2007). Transcription of the sal locus in S. pyogenes was determined to occur from two promoters, one of which is upstream of the operon. The other one is an internal promoter in the coding region of salY, directly upstream of the salKR genes that encode the putative TCS regulating expression of the sal locus (Namprachan-Frantz, Rowe, Runft, & Neely, 2014). Transposon insertions into salY and salK, as well as in frame deletion of salK, resulted in attenuated virulence of an HSC5 (M14) strain in the zebrafish infection model (Phelps & Neely, 2005). Additionally, the product of salR was found to repress activity of the salKR promoter, as did SalA, whereas human serum increased expression from the promoter (Namprachan-Frantz, Rowe, Runft, & Neely, 2014). Altogether, this indicates that regulation of the sal operon is complex and involves multiple inputs, while its activity influences S. pyogenes virulence through some as yet uncharacterized mechanisms.
Another regulator found to be involved in virulence in the context of human saliva is the maltose repressor, MalR, which, like CcpA, is a LacI/GalR family member. Insertional inactivation of MalR in the MGAS2221 strain reduced colonization of the mouse orophyarnx, but did not detrimentally affect invasive infection (Shelburne, et al., 2011), which suggests a specificity of MalR activity for the mucous membrane environment. The observed MalR transcriptome is limited to only 25 genes, and a highly conserved MalR DNA-binding sequence required for DNA interaction in vitro was identified. Inactivation of one of the transcriptional targets of MalR, the cell wall anchored carbohydrate binding and degrading enzyme encoded by pulA, significantly reduced S. pyogenes adhesion to human epithelial cells and mouse oropharyngeal colonization (Shelburne, et al., 2011).
Blood
Severe invasive infection by S. pyogenes, which involves its dissemination into blood and other sterile deep tissue sites, is most frequently caused by strains of serotypes M1T1, M3, and M12 (O'Loughlin, et al., 2007). Clinical isolates of these invasive strains exhibit hypervirulence and an enhanced capacity to invade soft tissues and evade neutrophil responses, in contrast with the more commonplace pharyngitis isolates (Kansal, et al., 2010; Li, et al., 2013). Thus, it has been of great importance to elucidate the virulence determinants required for S. pyogenes dissemination and survival in blood.
Characterization of the MGAS5005 (M1) transcript profile during growth in human blood revealed that the transcriptome of S. pyogenes undergoes extensive modulation in this environment (Graham, et al., 2005). Three-quarters (n = 1467) of encoded genes were differentially transcribed, with changes in expression that indicate the broad metabolic adaptation of S. pyogenes to the blood environment, shutting down glycolysis and activating amino acid-fermentation pathways. Induced virulence genes included Mga-dependent (such as emm and sic) and Mga-independent genes (hasABC, sagA, mac, slo, speA, speC, and smeZ), whereas speB and crgR transcripts were less abundant. In terms of regulation, exposure to blood resulted in the coordinated accumulation of mga, rofA, fasBCA and ihk/irr transcripts, as well as a temporal increase in covR/S expression. From these data, a regulatory model of S. pyogenes adaptation to the blood environment, composed of three phases, was proposed: persistence, adaptation, and dissemination. Persistence is mainly characterized by immune evasion through the up-regulation of mga and fasBCA, as well as down-regulation of crgR. Adaptation is typified by initiating cell contact, proteolysis, and peptide scavenging (along with up-regulation of covRS and ihk/ irr) and dissemination was defined as tissue destruction, cell necrosis, and apoptosis, accompanied by the up-regulation of RALPs and pyrogenic toxin antigens.
Further analysis of S. pyogenes virulence gene regulation in blood involved the examination of 51 gene transcripts in a codY-deficient NZ131 (M49) strain, which revealed differential responses for 26 genes that occasionally differed from responses seen in laboratory media (Malke & Ferretti, 2007). Differentially expressed regulators in this study included covR, fasX, mga, and sptR/S. Virulence genes with altered expression included cfa, emm49, grab, hasA, ideS, nga, prtS, scl, scpA, ska, slo, sof, and speH, as did genes encoding amino acid transporters and metabolic enzymes. Degenerate derivatives of the CodY binding box potentially serving as a cis-regulatory element for CodY regulation were identified in the upstream regions of 15 genes of the NZ131 genome, and these genes featured sequence motifs identical to the NZ131 CodY box in all completely sequenced S. pyogenes genomes. However, these genes consisted almost exclusively of metabolic and transporter encoding ORFs rather than virulence factors or regulators, which suggests that the observed differential transcription of the majority of virulence genes was caused by the indirect regulation by CodY of the S. pyogenes regulatory network.
Efforts have been recently made to identify the essential genes necessary for the survival of S. pyogenes in blood. Transposon-site hybridization (TraSH) analysis of a complex mariner-based transposon mutant library in a 5448 (M1T1) strain subjected to negative selection in human blood identified 81 genes that are important to S. pyogenes fitness in the blood environment (Le Breton, et al., 2013). This approach found regulatory genes already known to play a role in the survival of S. pyogenes in blood, such as mga, perR, and ralp3, as well as genes previously reported for their contribution to sepsis in other pathogens, such as genes involved in de novo nucleotide synthesis (purD, purA, pyrB, carA, carB, guaB), sugar metabolism (scrB, fruA), zinc uptake (adcC), and transcriptional regulators (cpsY). The LysR family transcriptional regulator CpsY is a transcriptional regulator found in several streptococci that regulate methionine transport and amino acid metabolism (MetR, MtaR). In S. pyogenes, CpsY is involved in resistance to human neutrophils and a ∆cpsY M1 strain does not exhibit the in vitro phenotypes of homologous mutants in other streptococcal species (Vega, et al., 2017). Furthermore, the S. pyogenes CpsY regulon is distinct from the regulons of its homologs and includes known virulence factors (mntE, speB, spd, nga/spn, prtS/spyCEP, and sse), as well as cell surface-associated factors of S. pyogenes (emm1, mur1.2, sibA/cdhA, and M5005_Spy0500). CpsY activity appears to be specific to the human host, as loss of CpsY does not result in S. pyogenes virulence defects in murine models of infection (Vega, et al., 2017). Further work characterizing genes that contribute to S. pyogenes behavior in blood will be important to identifying the major determinants of invasive infection.
The contribution of CovR/S regulation to S. pyogenes dissemination and survival in blood is also being actively investigated, due to this regulator’s association with invasive infection (reviewed in (Langshaw, Pandey, & Good, 2018)). Mutations in covS that attenuate the activity of its gene product toward CovR are a common cause of hypervirulence and enhancement of soft tissue invasion and innate immune evasion in invasive clinical isolates (Ikebe, et al., 2010; Shea, et al., 2011; Maamary, et al., 2012; Tatsuno, Okada, Zhang, Isaka, & Hasegawa, 2013; Garcia, et al., 2010; Masuno, et al., 2014) and animal passaged clones that arise from experimental invasive infection in mice (Sumby, Whitney, Graviss, DeLeo, & Musser, 2006; Walker, et al., 2007; Engleberg, Heath, Miller, Rivera, & DiRita, 2001). One outstanding feature of hypervirulent S. pyogenes strains with inactivated covS (CovR+S-) is the lack of detectable SpeB in culture supernatants (Maamary, et al., 2010). CovR phosphorylation decreases in the absence of active CovS, resulting in de-repression of virulence factor expression. Although there is evidence the virulence factor SpeB is negatively regulated by CovR (Heath, DiRita et al. 1999), expression of SpeB is almost completely repressed in a ∆covS mutant (Treviño, Perez et al. 2009). In an M1 strain, non-phosphorylated CovR acts as a transcriptional repressor for the SpeB-inducing regulator Rgg (Chiang-Ni, Chu et al. 2016). In addition, the expression of the Rgg-repressing regulator LacD.1 is upregulated in the ∆covS mutant. Chiang-Ni et al showed that overexpression of rgg but not inactivation of lacD.1 in the ∆covS mutant partially restored speB expression, indicating that only rgg repression, but not lacD.1 upregulation, contributes to the speB repression in a ∆covS mutant (Chiang-Ni, Chu et al. 2016). Intact covS greatly enhances the speB expression that is necessary for establishing early infectivity, after which the downregulation of speB expression promotes virulence (Cole, Barnett, Nizet, & Walker, 2011). This switch in speB expression is further facilitated by covS gene mutations that allow CovR to repress speB expression, thereby permitting other proteins (such as the products of sda1, ska, and slo) to avoid degradation by SpeB and sustaining their activities as S. pyogenes virulence factors (Aziz, et al., 2004; Cole, et al., 2006; Liang, et al., 2013). The selection for CovR+S- strains suggests that only colonies maximally discharging their virulence factors are able to survive in host compartments that are particularly hostile to S. pyogenes (namely, blood), whereas at sites without host immune surveillance, CovS is important for alleviating the energy burden of producing virulence gene products and allows for long-term colonization.
The consistency of gene mutations in covS that engender hypervirulent strains was examined by comparing the invasive globally disseminated 5448 (CovR+S+, M1T1) strain and the non-virulent engineered strain AP53 (CovR+S+) in a number of mouse-passage studies, where the same wild type bacteria were used to infect different mice (Mayfield, et al., 2014). Infection and dissemination sites were screened for SpeB-deficient clones also exhibiting increased sda1 and ska expression and activity. Characterization of the isolated clones showed many types of mutations that occur in the covS gene (such as frame-shift insertions, deletions, and in-frame small and large deletions), which indicates that, when establishing infection, the entire covS gene is susceptible to mutations and that clones that contain CovS-inactivating mutations affect numerous phenotypes, which leads to serious invasive disease. Most interestingly, characterization of CovS function in clinical isolates with single amino acid substitutions demonstrated that point mutations partially, but not completely, impaired the function of the covS alleles (Tatsuno, Okada, Zhang, Isaka, & Hasegawa, 2013). Unlike covS-knockout (ΔcovS) mutants, S. pyogenes strains that exhibit partial loss of CovS function stemming from covS alleles with point mutations were not impaired for growth in culture. As a result, complete loss of CovS function may confer greater virulence at the expense of the ability to respond to environmental cues recognized by CovS, which explains the abundance of point mutations among hypervirulent clinical isolates. Both the consequences of different covS mutations on S. pyogenes adaptation to host environments like blood and the mechanism driving these mutations require further study, though recent evidence hints that the latter entails interaction with host innate immunity, as described in the next section.
Host innate immunity (Ihk/Irr, CovR/S, RocA, LiaFSR)
Polymorphonuclear leukocytes (PMNs) and macrophages constitute host innate cellular immunity, engaging in pathogen removal once bacteria spread from their primary infection sites to deeper tissues or reach the blood stream. These cell types kill invading pathogens by producing extracellular factors, like neutrophil extracellular traps (NETs) and antimicrobial peptides (CAMPs) or by phagocytosing and destroying bacteria through phagosome acidification, ROS production via the oxidative burst, and/or the delivery of CAMPs and cytolytic toxins through granule fusion (reviewed in (Okumura & Nizet, 2014)). S. pyogenes evades or survives killing by these host immune processes in order to colonize and persist in the host through a variety of virulence factors under tight control by some of the regulators already discussed in this chapter, as well as through other dedicated transcriptional regulators.
Ihk/Irr
This TCS of S. pyogenes has been mentioned throughout the chapter, given that its expression is influenced in multiple environments (e.g. blood, saliva) and by other regulators (such as Nra). Multiple lines of evidence indicate that Ihk/Irr is a central regulator of S. pyogenes responses to innate cellular host immunity. It was initially identified in the investigation of Mga regulation (McIver, Subbarao, Kellner, Heath, & Scott, 1996) and later as part of the CovR/S regulon (Federle, McIver, & Scott, 1999), displaying homology with the ArlS/R virulence-gene regulating TCS of Staphylococcus aureus (Fournier, Klier, & Rapoport, 2001). Ihk/Irr exhibits an essential role in evasion of polymorphonuclear leukocyte (PMN)-mediated killing and is highly expressed during S. pyogenes-induced acute pharyngitis (Voyich, et al., 2003). In addition to the observed up regulation of virulence-factors encoded by sic, mac, speH, endoS, smeZ, speB and srtA, expression of the ihk and irr genes is also induced during phagocytosis by human PMNs. Deletion of the response regulator encoded by irr in the JRS4 (M6) strain analyzed did not enhance phagocytosis or production of ROS by PMNs in the presence of S. pyogenes, which suggests that the Ihk/Irr regulon enhances the survival of S. pyogenes within phagocytes (Voyich, et al., 2003). Further characterization of Ihk/Irr in JRS4 by genomewide microarray-based analysis of the Irr-dependent transcriptome showed Ihk/Irr controls the expression of genes involved in cell wall synthesis, oxidative stress responses (such as trx/thioredoxin, nrdH, nrdR), and of several virulence factor-encoding genes, including fbp (fibronectin-binding protein), mf, mf3 (both DNases), and sagA (streptolysin S) (Voyich, et al., 2004). In this same study, expression of ihk and irr transcripts was highly up regulated by S. pyogenes exposure to neutrophil primary granules and was moderately induced by hydrogen peroxide challenge, while the loss of irr expression resulted in induction (vicR) or repression (codY) of virulence-related regulator transcripts. Additionally, an irr-deficient strain in JRS4 (M6) was attenuated for virulence in vivo, as mice infected with the wild type strain formed abscesses more rapidly and that were of larger size, as compared to mice inoculated with the irr mutant, and infection with the irr-deficient strain was cleared more quickly from the blood (Voyich, et al., 2004). Examination of the S. pyogenes transcriptome using a 5448 (M1T1) strain challenged with human monocyte-derived macrophages demonstrated Ihk/Irr is involved in the early stages of adaptation to the environment within phagocytic cells (Hertzén, et al., 2012). In this study, intracellular bacteria exhibited increased expression of ihk and irr, in addition to higher expression of genes involved in cell wall synthesis and energy production, but only in the early stages of infection, as over time, ihk/irr transcript levels diminished with a concomitant rise in covR/S transcripts. An Ihk/Irr deficient mutant in the 5448 background produced lower intracellular bacterial counts from infected macrophages (Hertzén, et al., 2012), which demonstrate that Ihk/Irr TCS is required for S. pyogenes survival of innate cellular host immunity across strain backgrounds and phagocytic cell types.
CovR/S
Since it influences many of the transcripts involved in innate immune evasion (Federle, McIver, & Scott, 1999; Heath, DiRita, Barg, & Engleberg, 1999; Levin & Wessels, 1998; Treviño, et al., 2009), a great deal of research has examined CovR/S function in the interaction of S. pyogenes with host innate immunity. These studies uncovered that mutations in covRS associated with the loss of SpeB protease production and enhanced synthesis of the hyaluronic acid capsule are responsible for hypervirulent phenotypes (Li, et al., 2013; Aziz, et al., 2004; Cole, et al., 2006; Engleberg, Heath, Vardaman, & DiRita, 2004). Along with the lack of SpeB production (SpeB-) as a defining trait of S. pyogenes variants with covRS mutations, concomitant expression of the sda1-encoded DNase was observed to be critical to the selection of covRS mutations in 5448 (M1T1) during infection in mice (Walker, et al., 2007). High-level expression of sda1 facilitates the degradation of DNA in NETs, which promotes resistance to neutrophil-mediated killing at the initial site of infection (Buchanan, et al., 2006; Sumby, et al., 2005), while the deletion of sda1 abolished selection for covRS mutation in vivo following subcutaneous mouse infection (Walker, et al., 2007). In addition to Sda1, the capsule synthetase gene hasA and the M protein gene emm are required for the selection of SpeB− variants (Cole, et al., 2010). As previously mentioned, the loss of the broad-spectrum cysteine protease activity of SpeB inhibits the degradation of Sda1 and several additional virulence proteins, like streptokinase and the M1 protein, which are therefore able to interact with host plasminogen and accumulate plasmin activity on the bacterial cell surface to facilitate systemic spread (Aziz, et al., 2004; Cole, et al., 2006; Kansal, McGeer, Low, NorrbyTeglund, & Kotb, 2000). However, introduction of the Sda1-encoding prophage into an SF370 strain did not facilitate the selection of SpeB- mutants in vivo (Venturini, et al., 2013), and hypervirulent variants with covS mutations arise in strains that lack sda1 (Tsatsaronis, et al., 2013).
Genotype-dependent variations aside, the association of virulence factors required for phagocyte evasion with covS-deficient hypervirulent isolates suggests that interaction with host innate cellular immunity may be involved in the selection of S. pyogenes invasive strains. Screening infection sites for SpeB- isolates in neutrophil-depleted mice inoculated with MGAS2221 (CovR+S+, M1T1) revealed that neutrophils are the primary selection pressure for covRS mutation, as the lack of neutrophils drastically reduced the number of covS-deficient clones recovered from animal passaging (Li, et al., 2014). Additionally, an isolate with a null covS mutation from said passaging displayed enhanced survival and an enhanced capacity to evade innate immune responses. A novel role for CovRS was identified in the course of investigation of extracellular membrane-derived vesicle (MV) formation in S. pyogenes. MV structures form on and near their surface of S. pyogenes when growing in culture and following exposure to the antimicrobial peptide LL-37 (Biagini, et al., 2015; Uhlmann, et al., 2016). Analysis of MV content identified virulence-related protein substrates of the general secretory pathway, differentially abundant RNA species relative to cellular RNA, as well as substantial differences in the content, distributions, and fatty acid compositions of lipids in MVs relative to the S. pyogenes cell membrane (Resch, et al., 2016). The regulatory activity of an intact CovRS system was found to downregulate production of MVs, independently of SpeB expression and capsule biosynthesis. Further work is needed to characterize the mechanism(s) through which S. pyogenes -phagocyte interaction selects for invasive genotypes and the ways in which this contributes to S. pyogenes epidemiology.
Non-immune cells
The host cells infected by S. pyogenes are predetermined by the major entry ports the bacterium uses to enter the human body, thus making tonsillar epithelial cells and skin keratinocytes the primary targets in cases of infection of mucous membranes and the skin (Fiedler, Sugareva, Patenge, & Kreikemeyer, 2010). A prerequisite for colonization of these sites is the need to specifically adhere to epithelial cell surfaces, a task for which adhesion- and pilus-encoding genes found in a genotype-specific pattern within the FCT region are predominantly responsible. As previously indicated in this chapter, different members of the RALP family act as the primary regulators that influence the expression of FCT region genes in a serotype-dependent manner, but they are not the only ones to regulate them.
MsmR
MsmR is an AraC/XylS-type regulator that is identified in the FCT region and is shown to counteract Nra activity in the M49 serotype (Nakata, Podbielski, & Kreikemeyer, 2005). Microarray analysis of an msmR mutant in the M49 background showed that the genes located within or adjacent to the FCT region (prtF, cpa, spy0128, prtF2, nga, spy0166, slo, and spy0170), as well as genes of the Mga region (scl, spy2006, fbaA, scpA, sof sfbX, and hasA) were downregulated in the msmR mutant. Thus, MsmR acts as a positive regulator of all fibronectin-binding protein genes (prtF2, fbaA, sof, and sfbX) in serotype M49 (Nakata, Podbielski, & Kreikemeyer, 2005). In addition, the genes that encode cytolysin-mediated translocation system proteins, such as nga and slo, are upregulated by MsmR. Most of the upregulated genes in the msmR deficient mutant encode for prophage-associated proteins and stress-response factors, and the regulatory effects observed for MsmR on FCT region genes are in opposition to those of Nra/Ralp regulators (Nakata, Podbielski, & Kreikemeyer, 2005). MsmR activity, like that of the RALPs, appears to be strain-specific, as examination of an Alab49 (M53) strain showed that MsmR acts as a repressor of nra and pilus gene transcription, rather than as an inducer of such genes, as observed in the M49 genotype (Luo, Liziano, & Bessen, 2008). Comparison of M1 and M3 serotype strain transcriptomes revealed that low levels of pilus expression correlated with low levels of nra transcription, suggesting Nra induces pilus expression in M3 S. pyogenes (Calfee, et al., 2018). Concomitantly, low nra transcription and pilus expression reduced adherence to host cells and enhanced survival in human blood. Specificity appears to correlate to the regulator repertoire present in a strain, as replacement of the native nra-lineage allele and its respective upstream region with a rofA-lineage allele at the nra/rofA locus in the Alab49 strain produced a chimeric strain in which the polarity of MsmR activity was reversed, which made it an activator instead of a repressor of pilus gene expression, and without altering Nra regulatory activity on same said genes (Lizano, Luo, Tengra, & Bessen, 2008). This result highlights the importance of examining the activity of virulence gene regulators in S. pyogenes in the context of the regulatory network they constitute, as it shows that the exchange of orthologous forms of a regulatory gene can generate new phenotypes by altering the circuitry of the transcriptional regulatory network.
FasX
As previously described, the Fas regulator affects expression of S. pyogenes adhesins and streptokinase, which are factors involved in localized colonization and dissemination, respectively. To gain further insight into Fas function in the interaction with host epithelial cells, researchers used Affymetrix human genome DNA-arrays to measure the temporal and global transcriptional responses of HEp-2 cells infected with a fasX-deficient mutant and its M49 isogenic parent strain (Klenk, et al., 2005). A total of 86 HEp-2 cell genes were differentially transcribed upon infection, and included an increased expression of genes encoding fibronectin and integrin-α5 proteins involved in S. pyogenes host cell adherence and internalization. Activity of the Fas regulator appeared to promote high adherence and internalization rates, massive cytokine gene transcription and cytokine release, host cell apoptosis via a caspase-2 activation pathway, and cytotoxicity (Klenk, et al., 2005); whereas the absence of FasX decreased secreted IL-8 levels to below those of non-infected cells, which highlights the involvement of the Fas regulator in defining localized colonization vs. systemic spread in S. pyogenes infection.
Alternative Mechanisms of Gene Regulation
mRNA half-life of the S. pyogenes transcriptome influenced by RNase Y
Knowledge of global mRNA half-lives in bacterial species in general and pathogens in particular is limited, but can provide valuable insights on the adaptability of a species to changing environmental conditions. In Bacillus subtilis and Staphylococcus aureus, numerous transcription units are up or down regulated in mutants that carry a defective rny gene, which encodes the endoribonuclease RNase Y responsible for the initiation of mRNA degradation (Lehnik-Habrink, et al., 2011; Marincola, et al., 2012). A NZ131 rny mutant was assessed using a novel approach (steepest-slope method) for precise mRNA half-life determination on a transcriptome-wide scale (Chen, Itzek, Malke, Ferretti, & Kreth, 2013). The results encompassed 1,485 S. pyogenes genes (87.1% of the core genome) and revealed median and mean transcriptome half-lives of 0.89 min and 1.24 min, respectively, in the wild-type strain. The corresponding values for the rny mutant amount to 1.81 min and 2.79 min, respectively. In the wild type, the overwhelming majority of these genes (85%) show “unstable” mRNAs with half-lives shorter than 2.0 min, and only a small minority (2.3%) exhibit half-lives longer than 5.0 min (“stable” mRNAs). In contrast, the rny mutant gave corresponding percentage changes that changed to 56% and 12%, respectively. These data compare well with a 2-fold increase of overall mRNA stability, as affected by a non-functional rny gene. Compared to other bacterial species, which show longer transcriptome half-lives, the high mRNA turnover rate in S. pyogenes suggests that this species is particularly capable of adaptation to fast-changing environments during the course of the infection process (Figure 5).

Figure 5.
Independence and interaction of the regulatory systems important for amino acid starvation, mRNA stability, and global virulence in S. pyogenes.
RNase Y influences the expression of S. pyogenes virulence factors in a growth phase and nutrient-related manner (Kang, Caparon, & Cho, 2010). The influence of RNAse Y (previously dubbed CvfA) was most pronounced at the stationary phase of growth and in low carbohydrate media, conditions under which up to 30% of the transcriptome exhibited altered expression. Differentially expressed virulence genes included streptokinase ska, cfa, slo , emm, speB, sagA (Kang, Caparon, & Cho, 2010). While regulation of gene expression dependent on nutritional stress is commonly linked to the stringent response, loss of RNAse Y did not alter the levels of ppGpp; rather, RNAse Y interacted with enolase to control the transcript decay rates of virulence factors or their regulators (Kang, Caparon, & Cho, 2010). An RNAse Y-lacking mutant exhibited attenuated virulence in murine infection models. mRNA stability is also differentially affected in important functional groups of S. pyogenes genes (Chen, Itzek, Malke, Ferretti, & Kreth, 2013). For example, Clusters of Orthologous Groups (COGs (Tatusov, Koonin, & Lipman, 1997)) involved in energy production and translation are about 2- to 3-times more stable than the transcriptome average in the wild type S. pyogenes. The vast majority of mRNA in all 20 COGs studied showed a 1.7- 3.3-fold increase in the rny mutant. However, the stability profile of the different functional groups stays about the same in wild type and mutant strains, indicating that RNase Y does not preferentially affect certain functional groups. Resolving the general picture of rny-directed mRNA half-life control to the level of individual target genes leads to the identification of mRNAs, the half-lives of which are not significantly influenced by RNase Y (upp, ska, cfa; (Chen, Itzek, Malke, Ferretti, & Kreth, 2013)).
Further investigation of virulence gene regulation through mRNA stability in S. pyogenes revealed that RNase Y processing of the 5' untranslated (UTR) region of speB mRNA alters speB expression at the transcriptional level (Broglia, et al., 2018). Two RNase Y cleavage sites were identified downstream of a guanosine (G) residue, which in turn was found to be required for RNase Y processing of speB. Partial deletion of the speB 5' UTR resulted in accumulation of speB mRNA in stationary growth, due to retarded mRNA degradation (Chen, Mashburn-Warren, Merritt, Federle, & Kreth, 2017). In contrast to the observed role of RNAse Y in mRNA turnover during stationary growth (Kang, Caparon, & Cho, 2010; Broglia, et al., 2018), Chen et al. observed a weak interaction between the truncated speB 5' UTR and RNase Y compared with the wild-type, which suggests other unidentified RNA-degrading components are involved in the phenotypes produced by speB UTR truncation.
A study to define S. pyogenes mRNAs processed by RNase Y examined the transcriptomes of a S. pyogenes M49 strain and an isogenic RNase Y mutant (Δrny) using RNAseq and identified operons that display segmental stability in the wild type but not in the Δrny background (Chen, Raghavan, Qi, Merritt, & Kreth, 2019), which suggests RNAse Y processing. Of the 15 operons with mRNAs potentially processed by RNase Y, several associated with virulence were selected for confirmation (folC1, prtF, speG, ropB, and ypaA), of which only folC1 was confirmed to be processed, though whether solely RNAse Y was responsible is unclear (Chen, Raghavan, Qi, Merritt, & Kreth, 2019).
Cyclic di-AMP second messenger (c-di-AMP)
Cyclic di-AMP (c-di-AMP) is a second messenger nucleotide recently identified in Gram-positive bacteria, including Streptococcus species, Bacillus subtilis, and Staphylococcus aureus (Corrigan, Abbott, Burhenne, Kaever, & Gründling, 2011; Kamegaya, Kuroda, & Hayakawa, 2011; Gándara & Alonso, 2015; Andrade, et al., 2016). Li et al. provide a thorough review of the molecular mechanisms that involve cyclic dinucleotide messengers in streptococci (Li, et al., 2019). Diadenylate cyclases (DACs) synthesize c-di-AMP from two molecules of ATP or ADP through a condensation reaction (Pesavento & Hengge, 2009). Conversely, c-di-AMP phosphodiesterases (PDEs) degrade c-di-AMP and convert it into the linear form of phosphoadenyl adenosine (pApA) (Bai, et al., 2013). In S. pyogenes, DacA is not essential for growth in rich media, and a ∆dacA mutant produces no detectable biofilm and exhibits increased susceptibility to environmental stressors, such as high salt, low pH, reactive oxygen species, and cell wall-targeting antibiotics (Fahmi, Faozia, Port, & Cho, 2019). Conversely, a mutant that lacks the c-di-AMP phosphodiesterase Pde2 displays a lower growth rate and increased biofilm formation, distinct from a null mutant of another phosphodiesterase, GdpP, which suggests that Pde2 and GdpP play distinctive roles in c-di-AMP signaling. In addition to biofilm formation and stress response, c-di-AMP controls virulence in S. pyogenes, since DacA and Pde2 are required for the production of SpeB, and ∆dacA and ∆pde2 mutants are highly attenuated in a mouse model of subcutaneous infection (Fahmi, Faozia, Port, & Cho, 2019). K+ transport appears to be involved in c-di-AMP-mediated SpeB expression, as deletion of the transporter encoded by ktrB in a ΔdacA background restores SpeB expression (Faozia, Fahmi, Port, & Cho, 2021). KtrB forms a membrane K+ channel as a subunit of the KtrAB transport system, while KtrA is a cytosolic gating protein controlling K+ transport by binding ligands, including c-di-AMP. SpeB induction in the ΔdacA mutant using a K+ specific ionophore suggests cellular K+ balance contributes to SpeB production. A ΔdacA ΔktrB double mutant both produces wild-type levels of SpeB and is partially restored in its capacity to form biofilm and respond to stressors (Faozia, Fahmi, Port, & Cho, 2021). However, reduced virulence of a ∆dacA mutant in a murine subcutaneous infection model is not restored by ktrB deletion, which suggests that c-di-AMP controls not only cellular K+ balance but also other metabolic and/or virulence pathways.
Mobile genetic elements (Plasmids/prophage/ICE/transposons)
Multiple studies indicate that mobile genetic elements (MGEs), such as plasmids, prophage, integrative conjugative elements (ICEs) of S. pyogenes contribute to streptococcal fitness and disease potential. Bacteriophage encoding virulence factors (e.g. DNAse, superantigens) can enhance virulence of S. pyogenes (Sela, Euler, Correa da Rosa, & Fischetti, 2018) and ICEs encoding antimicrobial resistance genes may reduce antibiotic susceptibility (Ben Zakour, et al., 2015). An ICE dubbed RD2 enhances vaginal colonization by M28 S. pyogenes in mice (Jain, et al., 2019), and RD2-conjugants in other serotype backgrounds display a similar phenotype (Roshika, et al., 2021). The presence of the RD2 influenced expression of >100 core chromosomal S. pyogenes genes, including virulence factors, in a serotype-dependent manner (Jain, et al., 2019; Roshika, et al., 2021). Investigation of RD2 heterogeneity in the M28 serotype revealed that variation in a homo-polymeric tract located between RD2 genes Spy1336 and Spy1337 alters transcript levels of both genes in vitro, affects virulence in murine and nonhuman primate models of necrotizing myositis, and contributes to differences in global gene expression (Eraso, et al., 2020). The protein encoded by Spy1337 is a member of the AraC family of transcriptional regulators associated with genes encoding surface antigens, while Spy1336 encodes R28 protein, a homolog of the immunogenic Alp3 adhesin of Group B Streptococcus, which is suggestive that Spy1336/Spy1337 is a virulence factor/cognate regulator pair acquired via MGE that contributes to S. pyogenes virulence. Another example of MGE contribution to virulence regulation in S. pyogenes is the prophage-like ICE, SpyCIM1, which was shown to alter virulence and metabolism gene expression in an emm1 strain during exponential growth, when SpyCIM1 is episomal to the S. pyogenes chromosome (Hendrickson, et al., 2015).
Small RNAs (sRNA)
Analysis of sRNA regulators in S. pyogenes by sRNA sequencing of an M1 S. pyogenes strain identified 197 and 428 putative regulatory RNAs by visual inspection and bioinformatics screening of the sequencing data, respectively (Le Rhun, Beer, Reimegård, Chylinski, & Charpentier, 2016). Of these, 35 were assigned a predicted function (i.e. T-boxes, ribosomal protein leaders, characterized riboswitches or sRNAs), and 92 were novel putative sRNAs. Le Rhun et al. experimentally validated the expression of 30 novel sRNAs and antisense RNAs, indicating that expression of 9 sRNAs is affected by RNase III and/or RNase Y (Le Rhun, Beer, Reimegård, Chylinski, & Charpentier, 2016). Further investigation of RNAse III-targeted sRNAs in S. pyogenes defined 12 differentially expressed regions in an RNAse III-lacking strain that identified 6 novel putative sRNAs (Rath, Pitman, Cho, & Bai, 2017). Deletion of the RivX sRNA resulted in reduction of mga expression levels; thus, it was re-named MarS for Mga-activating regulatory sRNA (Pappesch, et al., 2017). RivX/MarS is conserved across streptococci, encoded downstream of rivR, and interacts with the 5' UTR of the mga transcript. Transcript and proteome analyses revealed concomitant reduction in expression of several Mga-activated genes, including M-protein, that stem from absence of MarS. Loss of MarS in turn resulted in increased susceptibility to phagocytosis and reduced adherence to human keratinocytes, as well as reduced dissemination to the liver, kidney, and spleen in a mouse infection model (Pappesch, et al., 2017).
CRISPR/Cas9
Clustered regularly interspaced short palindromic repeats (CRISPR) and CRISPR-associated (Cas) genes are an adaptive immune system prokaryotic organisms employ to defend against plasmids, bacteriophage and transposons (Barrangou, et al., 2007). Type II CRISPR-Cas systems occur only in bacteria, and not in archaea (Haft, Selengut, Mongodin, & Nelson, 2005) and are detected in various bacterial pathogens, including S. pyogenes (Louwen, Staals, Endtz, van Baarlen, & van der Oost, 2014)]. The Cas9 nuclease of S. pyogenes has become a tool of great interest in genome engineering, as it enables precise and efficient gene editing in a broad range of living cells that range from bacteria (Jiang, Bikard, Cox, Zhang, & Marraffini, 2013) to human cell lines (Cong, et al., 2013). The Cas9 nuclease has also been associated with S. pyogenes virulence, as a Cas9-lacking mutant in an M1 S. pyogenes strain exhibited reduced adherence to epithelial cells, reduced growth in human whole blood ex vivo, and attenuation of virulence in a murine necrotizing skin infection model (Gao, et al., 2019). Quantitative proteomic analysis of the Δcas9 strain indicated a significant reduction in the abundance of S. pyogenes virulence factors (SagH, ScpA, Sic, Grab, SpeJ) and changes in known virulence regulator levels (i.e. upregulation of CovRS, FasBCAX, Rgg; downregulation of PerR, Mga, YvqE/LiaS), which suggests that Cas9 influences S. pyogenes global gene regulation (Gao, et al., 2019).
Type I Restriction Modification System and the S. pyogenes Methylome
It has become possible to quickly and reliably determine the complete methylation profile of bacterial genomes (Murray, et al., 2012) as part of the single molecule, real-time (SMRT) sequencing procedure (Flusberg, et al., 2010). The methylated bases, created by DNA methyltransferases and present practically in all bacterial genomes, not only serve functions in restriction-modification systems (Euler, Ryan, Martin, & Fischetti, 2007), but also regulate the transcription of virulence genes (Casadesús & Low, 2006). Since the previous edition of this chapter, genome-wide epigenetic data has been published for S. pyogenes, and SMRT sequencing analysis has identified 412 putative N6-methyladenosine (m6A) sites throughout the genome of an M28 serotype S. pyogenes strain (Nye, et al., 2019). Deletion of the Restriction, Specificity, and Methylation gene subunits (∆RSM) of a putative Type I restriction modification system resulted in abrogation of all detectable m6A at the recognition sites in the generated ΔRSM strain, which also failed to prevent transformation with foreign-methylated DNA. RNA-sequencing determined that 20 genes were significantly down-regulated in the ΔRSM strain relative to its isogenic parent (Nye, et al., 2019). Among these was mga, which suggested that the ablated Type I restriction modification system influenced virulence gene expression. This hypothesis was supported by the observations that the ΔRSM strain elicited an enhanced host immune response in a murine subcutaneous infection model (e.g.. greater inflammation and tissue damage, increased levels of pro-inflammatory cytokines), survived poorly within human neutrophils, and had reduced adherence to human epithelial cells (Nye, et al., 2019). A more comprehensive analysis of DNA methylation in S. pyogenes using a database of 224 S. pyogenes genomes encompassing 80 M serotypes determined that nearly all S. pyogenes strains encode a type I restriction modification (RM) system that lacks the hsdS' alleles that influence global gene expression in other streptococcal species (DebRoy, et al., 2021). This study confirmed the presence of the S. pyogenes Type I restriction modification system on the core chromosome, as well as identified the sporadic presence of Type II orphan methyltransferases on prophages. Furthermore, they assigned 13 methylation patterns to the S. pyogenes population and confirmed that inactivation of the Type I restriction modification system abrogated DNA methylation detectable via either SMRT or MinION sequencing, as well as significantly increasing plasmid transformation rates (DebRoy, et al., 2021). In contrast to the report from Nye et al, the inactivation of the Type I system in a different M28 serotype strain and an M87 strain did not alter either mga transcript levels nor Mga-regulated gene expression. Further research will potentially elucidate whether the methylome is environment-dependent, or if a given methylome determines the tissue specificity of infection. Likewise, it will be interesting to find out whether the methylome of carrier strains differs from that of their invasive counterparts, which might explain how CodY turns a gene activator. Clearly, we need to gain knowledge about the epigenetic regulation of transcription, and can foresee exciting new fields of research arising in the near future.
Overall Perspectives
A survey such as this of existing knowledge on virulence gene regulation in S. pyogenes yields several overarching observations about the nature of virulence control in this strictly human pathogen. First, as introduced by the concept of the Red Queen argument, the regulatory networks of S. pyogenes have evolved in concert with its host niche. A clear example of this is the organization of various pathogenicity regions and regulons in the S. pyogenes genome (such as the FCT region, ERES, and CovRS regulon) whose structure and regulation correlates with the tissue environment and isolate in which it is found. Second, that the function of any given regulator and its contribution to virulence must be considered in the context of its interacting network, as multiple regulators have been shown to have varying, or even opposing, activity that depends on the strain genotype (such as CcpA or RALPs). Third, that the control of metabolic function and virulence are inextricably linked in a complex feedback network. This should come as no surprise, as the ultimate purpose of virulence factors is to ensure and enhance the survival of a pathogen in its environment, which in the case of S. pyogenes is the human host. Therefore, S. pyogenes has connected sensing of internal and environmental resources (such as carbohydrates and amino acids) to regulation of virulence proteins that act to evade host immunity, as well as proteins involved in nutrient acquisition (Figure 6). Fourth, in order to further the development of more effective therapeutics, it is necessary to study the S. pyogenes “regulome” as a system in constant flux, as it is very susceptible to mutation and dependent upon the context of the surrounding environment. Faster and more practical techniques for whole-genome characterization are becoming available, which make this sort of investigation less unwieldy, and it appears that this is the area of greatest promise to define the mechanisms that determine the success of S. pyogenes as a pathogen. Finally, Churchward et al. (Churchward, 2007; Churchward, Bates, Gusa, Stringer, & Scott, 2009) first suggested the two-component CovR/S regulatory network of S. pyogenes plays a Janus-like role in virulence gene expression. This includes the streptokinase gene (ska) where the forward face of Janus indicates promotion of the spreading activity of the pathogen, thus increasing its invasiveness. In contrast, the backward face of Janus reflects the use of streptokinase as a thrombolytic therapeutic agent for treating heart infarction in clinical medicine. The same analogy is valid for the production of a large number of host-damaging virulence factors (forward face) compared to many strains that provide the CRISPR-Cas9 chromosomal segment (backward face) for usage in genome editing in many domains of life (Marraffini, 2010; Marraffini, 2015).
Throughout this chapter, we have called attention to areas of research in S. pyogenes pathogenesis that either require deeper examination (such as the contribution of CovS-inactivating mutations to invasive disease) or that have the potential for uncovering novel regulatory relationships important to virulence (namely, PTS-dependent regulation of Mga activity). Research in S. pyogenes virulence regulation has the potential to drive the development of novel therapeutics to treat disease (such as targeting the stringent response or immune evasion signaling), as well as those in which innovative approaches promise to broaden our understanding of gene regulation in S. pyogenes pathogenesis.
References
- Almengor A. C., Kinkel T. L., Day S. J., McIver K. S. The catabolite control protein CcpA binds to Pmga and influences expression of the virulence regulator Mga in the Group A streptococcus. Journal of Bacteriology. 2007;189(23):8405–8416. [PMC free article: PMC2168945] [PubMed: 17905980]
- Alves-Barroco C., Caço J., Roma-Rodrigues C., Fernandes A. R., Bexiga R., Oliveira M., et al. Santos-Sanches I. New Insights on Streptococcus dysgalactiae subsp. dysgalactiae Isolates. Frontiers in Microbiology. 2021 July 15;12:686413. [PMC free article: PMC8319831] [PubMed: 34335512]
- Andrade W. A., Firon A., Schmidt T., Hornung V., Fitzgerald K. A., Kurt-Jones E. A., et al. Kaminski P.-A. Group B Streptococcus Degrades Cyclic-di-AMP to Modulate STING-Dependent Type I Interferon Production. Cell Host & MIcrobe. 2016 July 13;20(1):49–59. [PMC free article: PMC5382021] [PubMed: 27414497]
- Arias C. A., Panesso D., McGrath D. M., Qin X., Mojica M. F., Miller C., et al. Weinstock G. M. Genetic basis for in vivo daptomycin resistance in enterococci. The New England Journal of Medicine. 2011 September 8;365(10):892–900. [PMC free article: PMC3205971] [PubMed: 21899450]
- Babbar A., Barrantes I., Pieper D. H., Itzek A. Superantigen SpeA attenuates the biofilm forming capacity of Streptococcus pyogenes. Journal of Microbiology. Journal of Microbiology. 2019 July;57(7):626–636. [PubMed: 31054134]
- Bai Y., Yang J., Eisele L. E., Underwood A. J., Koestler B. J., Waters C. M., et al. Bai G. Two DHH subfamily 1 proteins in Streptococcus pneumoniae possess cyclic di-AMP phosphodiesterase activity and affect bacterial growth and virulence. Journal of Bacteriology. 2013 November;195(22):5123–5132. [PMC free article: PMC3811582] [PubMed: 24013631]
- Barrangou R., Fremaux C., Deveau H., Richards M., Boyaval P., Moineau S., et al. Horvath P. CRISPR provides acquired resistance against viruses in prokaryotes. Science. 2007 March 23;315(5819):1709–1712. [PubMed: 17379808]
- Ben Zakour N. L., Davies M. R., You Y., Chen J. H., Forde B. M., Stanton-Cook M., et al. Walker M. J. Transfer of scarlet fever-associated elements into the group A Streptococcus M1T1 clone. Scientific Reports. 2015 November 2;5:15877. [PMC free article: PMC4629146] [PubMed: 26522788]
- Bernard P. E., Duarte A., Bogdanov M., Musser J. M., Olsen R. J. Single Amino Acid Replacements in RocA Disrupt Protein-Protein Interactions To Alter the Molecular Pathogenesis of Group A. Infection and Immunity. 2020 October 19;88(11):e00386–20. [PMC free article: PMC7573446] [PubMed: 32817331]
- Bernard P. E., Kachroo P., Eraso J. M., Zhu L., Madry J. E., Linson S. E., et al. Olsen R. J. Polymorphisms in Regulator of Cov Contribute to the Molecular Pathogenesis of Serotype M28 Group A Streptococcus. The American Journal of Pathology. 2019 October;189(10):2002–2018. [PMC free article: PMC6892226] [PubMed: 31369755]
- Bernard P. E., Kachroo P., Zhu L., Beres S. B., Eraso J. M., Kajani Z., et al. Olsen R. J. RocA Has Serotype-Specific Gene Regulatory and Pathogenesis Activities in Serotype M28 Group A Streptococcus. Infection and Immunity. 2018 October 25;86(11):e00467–18. [PMC free article: PMC6204712] [PubMed: 30126898]
- Biagini M., Garibaldi M., Aprea S., Pezzicoli A., Doro F., Becherelli M., et al. Norais N. The Human Pathogen Streptococcus pyogenes Releases Lipoproteins as Lipoprotein-rich Membrane Vesicles. Molecular and Cellular Proteomics. 2015 August;14(8):2138–2149. [PMC free article: PMC4528243] [PubMed: 26018414]
- Biswas I., Scott J. R. Identification of rocA, a positive regulator of covR expression in the Group A streptococcus. Journal of Bacteriology. Journal of Bacteriology. 2003 May;185(10):3081–3090. [PMC free article: PMC154078] [PubMed: 12730168]
- Braza R. E., Silver A. B., Sundar G. S., Davis S. E., Razi A., Islam E., et al. McIver K. S. Phosphotransferase System Uptake and Metabolism of the β-Glucoside Salicin Impact Group A Streptococcal Bloodstream Survival and Soft Tissue Infection. Infection and Immunity. 2020 September 18;88(10):e00346–20. [PMC free article: PMC7504967] [PubMed: 32719156]
- Broglia L., Materne S., Lécrivain A.-L., Hahnke K., Le Rhun A., Charpentier E. RNase Y-mediated regulation of the streptococcal pyrogenic exotoxin B. RNA Biology. 2018;15(10):1336–1347. [PMC free article: PMC6284565] [PubMed: 30290721]
- Brouwer S., Cork A. J., Ong C.-L. Y., Barnett T. C., West N. P., McIver K. S., Walker M. J. Endopeptidase PepO Regulates the SpeB Cysteine Protease and Is Essential for the Virulence of Invasive M1T1 Streptococcus pyogenes. Journal of Bacteriology. 2018 March 26;200(8):e00654–17. [PMC free article: PMC5869480] [PubMed: 29378883]
- Buckley S. J., Davies M. R., McMillan D. J. In silico characterisation of stand-alone response regulators of Streptococcus pyogenes. PLoS One. 2020 October 19;15(10):e0240834. [PMC free article: PMC7571705] [PubMed: 33075055]
- Calfee G., Danger J. L., Jain I., Miller E. W., Sarkar P., Tjaden B., et al. Sumby P. Identification and Characterization of Serotype-Specific Variation in Group A Streptococcus Pilus Expression. Infection and Immunity. 2018 January 22;86(2):e00792–17. [PMC free article: PMC5778356] [PubMed: 29158432]
- Chaussee M. S., Watson R. O., Smoot J. C., Musser J. M. Identification of Rgg-regulated exoproteins of Streptococcus pyogenes. Infection and Immunity. 2001;69(2):822–831. [PMC free article: PMC97958] [PubMed: 11159974]
- Chen Z., Mashburn-Warren L., Merritt J., Federle M. J., Kreth J. Interference of a speB 5' untranslated region partial deletion with mRNA degradation in Streptococcus pyogenes. Molecular Oral Microbiology. 2017 October;32(5):390–403. [PMC free article: PMC10030001] [PubMed: 28371435]
- Chen Z., Raghavan R., Qi F., Merritt J., Kreth J. Genome-wide screening of potential RNase Y-processed mRNAs in the M49 serotype Streptococcus pyogenes NZ131. MicrobiologyOpen. 2019 April;8(4):e00671. [PMC free article: PMC6460267] [PubMed: 29900693]
- Chiang-Ni C., Chiou H.-J., Tseng H.-C., Hsu C.-Y., Chiu C.-H. RocA Regulates Phosphatase Activity of Virulence Sensor CovS of Group A Streptococcus. mSphere. 2020 May 20;5(3):e00361–20. [PMC free article: PMC7380576] [PubMed: 32434842]
- Chiang-Ni C., Tseng H.-C., Hung C.-H., Chiu C.-H. Acidic stress enhances CovR/S-dependent gene repression through activation of the covR/S promoter in emm1-type Group A Streptococcus. International Journal of Medical Microbiology. 2017 September;307(6):329–339. [PubMed: 28648357]
- Chiang-Ni C., Tseng H.-C., Shi Y.-A., Chiu C.-H. Effect of Phosphatase Activity of the Control of Virulence Sensor (CovS) on Clindamycin-Mediated Streptolysin O Production in Group A. Infection and Immunity. 2019 November 18;87(12):e00583–19. [PMC free article: PMC6867833] [PubMed: 31527126]
- Churchward G. The two faces of Janus: virulence gene regulation by CovR/S in group A streptococci. Molecular Microbiology. 2007;64(1):34–41. [PubMed: 17376070]
- Churchward G., Bates C., Gusa A. A., Stringer V., Scott J. R. Regulation of streptokinase expression by CovR/S in Streptococcus pyogenes: CovR acts through a single high-affinity binding site. Microbiology. 2009;155(Pt 2):566–575. [PMC free article: PMC4130213] [PubMed: 19202105]
- Cole J. N., Nizet V. Bacterial Evasion of Host Antimicrobial Peptide Defenses. Microbiology Spectrum. 2016 January 29;4(1) [PMC free article: PMC4804471] [PubMed: 26999396]
- Cong L., Ran F. A., Cox D., Lin S., Barretto R., Habib N., et al. Zhang F. Multiplex genome engineering using CRISPR/Cas systems. Science. 2013 February 15;339(6121):819–823. [PMC free article: PMC3795411] [PubMed: 23287718]
- Corrigan R. M., Abbott J. C., Burhenne H., Kaever V., Gründling A. c-di-AMP is a new second messenger in Staphylococcus aureus with a role in controlling cell size and envelope stress. PLoS Pathogens. 2011 September;7(9):e1002217. [PMC free article: PMC3164647] [PubMed: 21909268]
- Danger J. L., Cao T. N., Cao T. H., Sarkar P., Treviño J., Pflughoeft K. J., Sumby P. The small regulatory RNA FasX enhances Group A Streptococcus virulence and inhibits pilus expression via serotype-specific targets. Molecular Microbiology. 2015 April;96(2):249–262. [PMC free article: PMC4390479] [PubMed: 25586884]
- Danger J. L., Makthal N., Kumaraswami M., Sumby P. The FasX Small Regulatory RNA Negatively Regulates the Expression of Two Fibronectin-Binding Proteins in Group A Streptococcus. Journal of Bacteriology. 2015 December;197(23):3720–3730. [PMC free article: PMC4626899] [PubMed: 26391206]
- DebRoy. Genome-wide analysis of in vivo CcpA binding with and without its key co-factor HPr in the major human pathogen group A Streptococcus. Molecular Microbiology. 2021 June;115(6):1207–1228. S., Aliaga-Tobar, V., Galvez, G., Arora, S., Liang, X., Horstmann, N., . . . Shelburne, S. A. [PMC free article: PMC8359418] [PubMed: 33325565]
- DebRoy, S., Shropshire, W. C., Tran, C. N., Hao, H., Gohel, M., Galloway-Peña, J., . . . Shelburne, S. A. (2021, December 22). Characterization of the Type I Restriction Modification System Broadly Conserved among Group A Streptococci. mSphere, 6(6), e0079921. [PMC free article: PMC8597746] [PubMed: 34787444]
- Do H., Makthal N., VanderWal A. R., Rettel M., Savitski M. M., Peschek N., et al. Kumaraswami M. Leaderless secreted peptide signaling molecule alters global gene expression and increases virulence of a human bacterial pathogen. Proceedings of the National Academy of Sciences of the United States of America. 2017 September 18;114(40):E8498–E8507. [PMC free article: PMC5635878] [PubMed: 28923955]
- Do H., Makthal N., VanderWal A. R., Saavedra M. O., Olsen R. J., Musser J. M., Kumaraswami M. Environmental pH and peptide signaling control virulence of Streptococcus pyogenes via a quorum-sensing pathway. Nature Communications. 2019 June 13;10:2586. [PMC free article: PMC6565748] [PubMed: 31197146]
- Eraso J. M., Kachroo P., Olsen R. J., Beres S. B., Zhu L., Badu T., et al. Musser J. M. Genetic heterogeneity of the Spy1336/R28-Spy1337 virulence axis in Streptococcus pyogenes and effect on gene transcript levels and pathogenesis. PLoS One. 2020 March 26;15(3):e0229064. [PMC free article: PMC7098570] [PubMed: 32214338]
- Fahmi T., Faozia S., Port G. C., Cho K. H. The Second Messenger c-di-AMP Regulates Diverse Cellular Pathways Involved in Stress Response, Biofilm Formation, Cell Wall Homeostasis, SpeB Expression, and Virulence in Streptococcus pyogenes. Infection and Immunity. 2019 May 21;87(6):e00147–19. [PMC free article: PMC6529668] [PubMed: 30936159]
- Faozia S., Fahmi T., Port G. C., Cho K. H. c-di-AMP-Regulated KtrAB affects biofilm formation, stress response, and SpeB expression in Streptococcus pyogenes. Infection and Immunity. 2021 March 17;89(4):e00317–20. [PMC free article: PMC8090949] [PubMed: 33468578]
- Feng W., Minor D., Liu M., Li J., Ishaq S. L., Yeoman C., Lei B. Null Mutations of Group A Streptococcus Orphan Kinase RocA: Selection in Mouse Infection and Comparison with CovS Mutations in Alteration of In Vitro and In Vivo Protease SpeB Expression and Virulence. Infection and Immunity. 2016 December 29;85(1):e00790–16. [PMC free article: PMC5203639] [PubMed: 27795364]
- Finn M. B., Ramsey K. M., Dove S. L., Wessels M. R. Identification of Group A Streptococcus Genes Directly Regulated by CsrRS and Novel Intermediate Regulators. mBio. 2021 August 31;12(4):e0164221. [PMC free article: PMC8406183] [PubMed: 34253064]
- Flores A. R., Jewell B. E., Yelamanchili D., Olsen R. J., Musser J. M. A Single Amino Acid Replacement in the Sensor Kinase LiaS Contributes to a Carrier Phenotype in Group A Streptococcus. Infection and Immunity. 2015 November;83(11):4237–4246. [PMC free article: PMC4598398] [PubMed: 26283331]
- Gándara C., Alonso J. C. DisA and c-di-AMP act at the intersection between DNA-damage response and stress homeostasis in exponentially growing Bacillus subtilis cells. DNA Repair. 2015 March;27:1–8. [PubMed: 25616256]
- Ganz T., Selsetd M. E., Szklarek D., Harwig S. S., Daher K., Bainton D. F., Lehrer R. I. Defensins. Natural peptide antibiotics of human neutrophils. The Journal of Clinical Investigation. 1985 October;76(4):1427–1435. [PMC free article: PMC424093] [PubMed: 2997278]
- Gao N. J., Al-Bassam M. M., Poudel S., Wozniak J. M., Gonzalez D. J., Olson J., et al. Valderrama J. A. Functional and Proteomic Analysis of Streptococcus pyogenes Virulence Upon Loss of Its Native Cas9 Nuclease. Frontiers in Microbiology. 2019 August 22;10:1967. [PMC free article: PMC6714885] [PubMed: 31507572]
- García-Fernández E., Koch G., Wagner R. M., Fekete A., Stengel S. T., Schneider J., et al. Lopez D. Membrane Microdomain Disassembly Inhibits MRSA Antibiotic Resistance. Cell. 2017 November 30;171(6):1354–1367.e20. [PMC free article: PMC5720476] [PubMed: 29103614]
- Gera K., Le T., Jamin R., Eichenbaum Z., McIver K. S. The phosphoenolpyruvate phosphotransferase system in Group A Streptococcus acts to reduce Streptolysin S activity and lesion severity during soft tissue infection. Infection and Immunity. 2014 March;82(3):1192–1204. [PMC free article: PMC3957985] [PubMed: 24379283]
- Haft D. H., Selengut J., Mongodin E. F., Nelson K. E. A guild of 45 CRISPR-associated (Cas) protein families and multiple CRISPR/Cas subtypes exist in prokaryotic genomes. PLoS Computational Biology. 2005 November;1(6):e60. [PMC free article: PMC1282333] [PubMed: 16292354]
- Hendrickson C., Euler C. W., Nguyen S. V., Rahman M., McCullor K. A., King C. J., et al. McShan W. M. Elimination of Chromosomal Island SpyCIM1 from Streptococcus pyogenes Strain SF370 Reverses the Mutator Phenotype and Alters Global Transcription. PLoS One. 2015 December 23;10(12):e0145884. [PMC free article: PMC4689407] [PubMed: 26701803]
- Herrera A. L., Callegari E. A., Chaussee M. S. The Streptococcus pyogenes signaling peptide SpoV regulates streptolysin O and enhances survival in murine blood. Journal of Bacteriology. 2021 March 15;203(11):e00586–20. [PMC free article: PMC8117530] [PubMed: 33722844]
- Hondorp E. R., McIver K. S. The Mga virulence regulon: infection where the grass is greener. Molecular Microbiology. 2007;66(5):1056–1065. [PubMed: 18001346]
- Horstmann N., Sahasrabhojane P., Yao H., Su X., Shelburne S. A. Use of a Phosphorylation Site Mutant To Identify Distinct Modes of Gene Repression by the Control of Virulence Regulator (CovR) in Streptococcus pyogenes. Journal of Bacteriology. 2017 August 22;199(18) [PMC free article: PMC5573087] [PubMed: 28289082]
- Horstmann N., Tran C. N., Brumlow C. Phosphatase activity of the control of virulence sensor kinase CovS is critical for the pathogenesis of group A streptococcus. PLoS Pathogens. 2018 October 31;14(10):e1007354. DebRoy, S., Yao, H., Gonzalez, G. N., . . . Shelburne, S. A. [PMC free article: PMC6231683] [PubMed: 30379939]
- Isaka M., Okamoto A., Miura Y., Tatsuno I., Maeyama J.-I., Hasegawa T. Streptococcus pyogenes TrxSR Two-Component System Regulates Biofilm Production in Acidic Environments. Infection and Immunity. 2021 October 15;89(11):e0036021. [PMC free article: PMC8519301] [PubMed: 34424754]
- Isaka M., Tatsuno I., Maeyama J.-I., Matsui H., Zhang Y., Hasegawa T. The YvqE two-component system controls biofilm formation and acid production in Streptococcus pyogenes. APMIS. 2016 July;124(7):574–585. [PubMed: 27061781]
- Jain I., Danger J. L., Burgess C., Uppal T., Sumby P. The group A Streptococcus accessory protein RocA: regulatory activity, interacting partners and influence on disease potential. Molecular Microbiology. 2020 January;113(1):190–207. [PMC free article: PMC7028121] [PubMed: 31660653]
- Jain I., Miller E. W., Danger J. L., Pflughoeft K. J., Sumby P. RocA Is an Accessory Protein to the Virulence-Regulating CovRS Two-Component System in Group A Streptococcus. Infection and Immunity. 2017 October 18;85(11):e00274–17. [PMC free article: PMC5649027] [PubMed: 28808155]
- Jain I., Sarkar P., Danger J. L., Medicielo J., Roshika R., Calfee G., et al. Sumby P. A Mobile Genetic Element Promotes the Association Between Serotype M28 Group A Streptococcus Isolates and Cases of Puerperal Sepsis. The Journal of Infectious Diseases. 2019 July 31;220(5):882–891. [PMC free article: PMC6667793] [PubMed: 31107945]
- Jiang W., Bikard D., Cox D., Zhang F., Marraffini L. A. RNA-guided editing of bacterial genomes using CRISPR-Cas systems. Nature Biotechnology. Nature Biotechnology. 2013 January 29;31:233–239. [PMC free article: PMC3748948] [PubMed: 23360965]
- Kamegaya T., Kuroda K., Hayakawa Y. Identification of a Streptococcus pyogenes SF370 gene involved in production of c-di-AMP. Nagoya Journal of Medical Science. 2011 February;73(1-2):49–57. [PMC free article: PMC11254362] [PubMed: 21614937]
- Kang S. O., Caparon M. G., Cho K. H. Virulence gene regulation by CvfA, a putative RNase: the CvfA-enolase complex in Streptococcus pyogenes links nutritional stress, growth-phase control, and virulence gene expression. Infection and Immunity. 2010 June;78(6):2754–2767. [PMC free article: PMC2876558] [PubMed: 20385762]
- Khan A., Davlieva M., Panesso D., Rincon S., Miller W. R., Diaz L., et al. Arias C. A. Antimicrobial sensing coupled with cell membrane remodeling mediates antibiotic resistance and virulence in Enterococcus faecalis. Proceedings of the National Academy of Sciences of the United States of America. 2019 December 9;116(52):26925–26932. [PMC free article: PMC6936494] [PubMed: 31818937]
- Kietzman C. C., Caparon M. G. CcpA and LacD.1 affect temporal regulation of Streptococcus pyogenes virulence genes. Infection and Immunity. 2010;78(1):241–252. [PMC free article: PMC2798178] [PubMed: 19841076]
- Langshaw E. L., Pandey M., Good M. F. Cellular interactions of covR/S mutant group A Streptococci. Microbes and Infection. 2018 Oct-Nov;20(9-10):531–535. [PubMed: 29287985]
- Le Rhun A., Beer Y., Reimegård J., Chylinski K., Charpentier E. RNA sequencing uncovers antisense RNAs and novel small RNAs in Streptococcus pyogenes. RNA Biology. 2016;13(2):177–195. [PMC free article: PMC4829319] [PubMed: 26580233]
- Li Z., Zhang X., Cheng L., Xu X., Zhou X., Wu H., Peng X. Signal Transduction of streptococci by Cyclic Dinucleotide Second Messengers. Current Issues in Molecular Biology. 2019;32:87–122. [PubMed: 31166170]
- Lin Y., Sanson M. A., Vega L. A., Shah B., Regmi S., Cubria M. B., Flores A. R. ExPortal and the LiaFSR regulatory system coordinate the response to cell membrane stress in Streptococcus pyogenes. mBio. 2020 September 15;11(5) [PMC free article: PMC7492735] [PubMed: 32934083]
- Lizano S., Luo F., Tengra F. K., Bessen D. E. Impact of orthologous gene replacement on the circuitry governing pilus gene transcription in streptococci. PLoS One. 2008;3(10):e3450. [PMC free article: PMC2565503] [PubMed: 18941636]
- López D., Kolter R. Functional Microdomains in Bacterial Membranes. Genes & Development. 2010 September 1;24(17):1893–1902. [PMC free article: PMC2932971] [PubMed: 20713508]
- Louwen R., Staals R. H., Endtz H. P., van Baarlen P., van der Oost J. The role of CRISPR-Cas systems in virulence of pathogenic bacteria. Microbiology and Molecular Biology Reviews. 2014 March;78(1):74–88. [PMC free article: PMC3957734] [PubMed: 24600041]
- Lynskey N. N., Goudling D., Gierula M., Turner C. E., Dougan G., Edwards R. J., Sriskandan S. RocA truncation underpins hyper-encapsulation, carriage longevity and transmissibility of serotype M18 group A streptococci. PLoS Pathogens. 2013 December;9(12):e1003842. [PMC free article: PMC3868526] [PubMed: 24367267]
- Lynskey N. N., Turner C. E., Heng L. S., Sriskandan S. A truncation in the regulator RocA underlies heightened capsule expression in serotype M3 Group A streptococci. Infection and Immunity. 2015 April;83(4):1732–1733. [PMC free article: PMC4363422] [PubMed: 25784754]
- Lynskey N. N., Velarde J. J., Finn M. B., Dove S. L., Wessels M. R. RocA Binds CsrS To Modulate CsrRS-Mediated Gene Regulation in Group A. mBio. 2019 July 16;10(4) [PMC free article: PMC6635533] [PubMed: 31311885]
- Makthal N., Do H., VanderWal A. R., Olsen R. J., Musser J. M., Kumaraswami M. Signaling by a Conserved Quorum Sensing Pathway Contributes to Growth. Infection and Immunity. 2018 April 23;86(5):e00169–18. [PMC free article: PMC5913841] [PubMed: 29531135]
- Makthal N., Do H., Wendel B. M., Olsen R. J., Helmann J. D., Musser J. M., Kumaraswami M. Group A Streptococcus AdcR Regulon Participates in Bacterial Defense against Host-Mediated Zinc Sequestration and Contributes to Virulence. Infection and Immunity. 2020 July 21;88(8):e00097–20. [PMC free article: PMC7375770] [PubMed: 32393509]
- Marraffini L. A. Impact of CRISPR Immunity on the Emergence of Bacterial Pathogens. Future Medicine. 2010 May 5;5(5):693–695. [PubMed: 20441541]
- Marraffini L. A. CRISPR-Cas Immunity in Prokaryotes. Nature. 2015 October 1;526(7571):55–61. [PubMed: 26432244]
- Mascher T. Intramembrane-sensing histidine kinases: a new family of cell envelope stress sensors in Firmicutes bacteria. FEMS Microbiology Letters. 2006 November;264(2):133–144. [PubMed: 17064367]
- Melo M. N., Ferre R., Castanho M. A. Antimicrobial peptides: linking partition, activity and high membrane-bound concentrations. Nature Reviews Microbiology. 2009 March;7:245–250. [PubMed: 19219054]
- Miller E. W., Danger J. L., Ramalinga A. B., Horstmann N., Shelburne S. A., Sumby P. Regulatory rewiring confers serotype-specific hyper-virulence in the human pathogen group A Streptococcus. Molecular Microbiology. 2015 October;98(3):473–489. [PMC free article: PMC4675629] [PubMed: 26192205]
- Miller E. W., Pflughoeft K. J., Sumby P. Reply to "A truncation in the regulator RocA underlies heightened capsule expression in serotype M3 group A streptococci". Infection and Immunity. 2015 April;83(4):1734. [PMC free article: PMC4363452] [PubMed: 25784755]
- Nye T. M., Jacob K. M., Holley E. K., Nevarez J. M., Dawid S., Simmons L. A., Watson M. E. Jr. DNA methylation from a Type I restriction modification system influences gene expression and virulence in Streptococcus pyogenes. PLoS Pathogens. 2019 June 17;15(6):e1007841. [PMC free article: PMC6597129] [PubMed: 31206562]
- Paluscio E., Watson M. E. Jr, Caparon M. G. CcpA Coordinates Growth/Damage Balance for Streptococcus pyogenes Pathogenesis. Scientific Reports. 2018 September 24;8:14254. [PMC free article: PMC6155242] [PubMed: 30250043]
- Pappesch R., Warnke P., Mikkat S., Normann J., Wisniewska-Kucper A., Huschka F., et al. Patenge N. The Regulatory Small RNA MarS Supports Virulence of Streptococcus pyogenes. Scientific Reports. 2017 September 25;7:12241. [PMC free article: PMC5613026] [PubMed: 28947755]
- Pérez Morales T. G., Ratia K., Wang D.-S., Gogos A., Bloem L., Driver T. G., Federle M. J. A novel chemical inducer of Streptococcus quorum sensing acts by inhibiting the phermone-degrading endopeptidase PepO. The Journal of Biological Chemistry. 2018 January 19;293(3):931–940. [PMC free article: PMC5777264] [PubMed: 29203527]
- Pesavento C., Hengge R. Bacterial nucleotide-based second messengers. Current Opinion in Microbiology. 2009 April;12(2):170–176. [PubMed: 19318291]
- Plančak D., Musić L., Puhar I. Quorum Sensing of Periodontal Pathogens. Acta Stomatologica Croatica. 2015 September;49(3):234–241. [PMC free article: PMC4993591] [PubMed: 27688408]
- Port G. C., Cusumano Z. T., Tumminello P. R., Caparon M. G. SpxA1 and SpxA2 Act Coordinately To Fine-Tune Stress Responses and Virulence in Streptococcus pyogenes. mBio. 2017 March 28;8(2):e00288–17. [PMC free article: PMC5371413] [PubMed: 28351920]
- Ramalinga A., Danger J. L., Makthal N., Kumaraswami M., Sumby P. Multimerization of the Virulence-Enhancing Group A Streptococcus Transcription Factor RivR Is Required for Regulatory Activity. Journal of Bacteriology. 2016 December 13;199(1) [PMC free article: PMC5165092] [PubMed: 27795318]
- Rath E. C., Pitman S., Cho K. H., Bai Y. Identification of streptococcal small RNAs that are putative targets of RNase III through bioinformatics analysis of RNA sequencing data. BMC Bioinformatics. 2017 December 28;18:540. [PMC free article: PMC5751559] [PubMed: 29297355]
- Resch U., Tsatsaronis J. A., Le Rhun A., Stübiger G., Rohde M., Kasvandik S., et al. Charpentier E. A Two-Component Regulatory System Impacts Extracellular Membrane-Derived Vesicle Production in Group A Streptococcus. mBio. 2016 November 1;7(6):e00207–16. [PMC free article: PMC5090034] [PubMed: 27803183]
- Rojas-Tapias D. F., Helmann J. D. Roles and regulation of Spx family transcription factors in Bacillus subtilis and related species. Advances in Microbial Physiology. 2019;75:279–323. [PMC free article: PMC7055150] [PubMed: 31655740]
- Rom J. S., Hart M. T., McIver K. S. PRD-Containing Virulence Regulators (PCVRs) in Pathogenic Bacteria. Frontiers in Cellular and Infection Microbiology. 2021 October 19;11:772874. [PMC free article: PMC8560693] [PubMed: 34737980]
- Roobthaisong A., Aikawa C., Nozawa T., Maruyama F., Nakagawa I. YvqE and CovRS of Group A Streptococcus Play a Pivotal Role in Viability and Phenotypic Adaptations to Multiple Environmental Stresses. PLoS One. 2017;12(1):e0170612. [PMC free article: PMC5266302] [PubMed: 28122066]
- Rosch J. W., Caparon M. G. The ExPortal: an organelle dedicated to the biogenesis of secreted proteins in Streptococcus pyogenes. Molecular Microbiology. 2005 November;58(4):959–968. [PubMed: 16262783]
- Rosch J., Caparon M. A microdomain for protein secretion in Gram-positive bacteria. Science. 2004 June 4;304(5676):1513–1515. [PubMed: 15178803]
- Roshika R., Jain I., Medicielo J., Wächter J., Danger J. L., Sumby P. The RD2 Pathogenicity Island Modifies the Disease Potential of the Group A Streptococcus. Infection and Immunity. 2021 July 15;89(8):e0072220. [PMC free article: PMC8281230] [PubMed: 33820819]
- Sanson M. A., Vega L. A., Shah B., Regmi S., Cubria M. B., Horstmann N., et al. Flores A. R. The LiaFSR transcriptome reveals an interconnected regulatory network in group A streptococcus. Infection and Immunity. 2021 October 15;89(11):e0021521. [PMC free article: PMC8519277] [PubMed: 34370508]
- Sarkar P., Danger J. L., Jain I., Meadows L. A., Beam C., Medicielo J., et al. Sumby P. Phenotypic Variation in the Group A Streptococcus. mSphere. 2018 October 17;3(5):e00519–18. [PMC free article: PMC6193603] [PubMed: 30333182]
- Saroj S. D., Holmer L., Berengueras J. M., Jonsson A.-B. Inhibitory role of acyl homoserine lactones in hemolytic activity and viability of Streptococcus pyogenes M6 S165. Scientific Reports. 2017 March 17;7:44902. [PMC free article: PMC5355980] [PubMed: 28303956]
- Sela U., Euler C. W., Correa da Rosa J., Fischetti V. A. Strains of bacterial species induce a greatly varied acute adaptive immune response: The contribution of the accessory genome. PLoS Pathogens. 2018 January 11;14(1):e1006726. [PMC free article: PMC5764401] [PubMed: 29324905]
- Sundar G. S., Islam E., Gera K., Le Breton Y., McIver K. S. A PTS EII mutant library in Group A Streptococcus identifies a promiscuous Man-family PTS transporter influencing SLS-mediated hemolysis. Molecular Microbiology. 2017 February;103(3):518–533. [PMC free article: PMC5263093] [PubMed: 27862457]
- Uhlmann J., Rohde M., Siemens N., Kreikemeyer B., Bergman P., Johansson L., Norrby-Teglund A. LL-37 Triggers Formation of Streptococcus pyogenes Extracellular Vesicle-Like Structures with Immune Stimulatory Properties. Journal of Innate Immunity. 2016;8(3):243–257. [PMC free article: PMC6738779] [PubMed: 26641861]
- Valdes K. M., Sundar G. S., Belew A. T., Islam E., El-Sayed N. M., Le Breton Y., McIver K. S. Glucose Levels Alter the Mga Virulence Regulon in the Group A Streptococcus. Scientific Reports. 2018 March 21;8(1):4971. [PMC free article: PMC5862849] [PubMed: 29563558]
- Valdes K. M., Sundar G. S., Vega L. A., Belew A. T., Islam E., Binet R., et al. McIver K. S. The fruRBA Operon Is Necessary for Group A Streptococcal Growth in Fructose and for Resistance to Neutrophil Killing during Growth in Whole Human Blood. Infection and Immunity. 2016 March 24;84(4):1016–1031. [PMC free article: PMC4807502] [PubMed: 26787724]
- VanderWal A. R., Makthal N., Pinochet-Barros A., Helmann J. D., Olsen R. J., Kumaraswami M. Iron Efflux by PmtA Is Critical for Oxidative Stress Resistance and Contributes Significantly to Group A Streptococcus Virulence. Infection and Immunity. 2017 May 23;85(6):e00091–17. [PMC free article: PMC5442632] [PubMed: 28348051]
- Vega L. A., Caparon M. G. Cationic antimicrobial peptides disrupt the Streptococcus pyogenes ExPortal. Molecular Microbiology. 2012 September;85(6):1119–1132. [PMC free article: PMC3646575] [PubMed: 22780862]
- Vega L. A., Port G. C., Caparon M. G. An association between peptidoglycan synthesis and organization of the Streptococcus pyogenes ExPortal. mBio. 2013 September 24;4(5):e00485–13. [PMC free article: PMC3781834] [PubMed: 24065630]
- Vega L. A., Valdes K. M., Sundar G. S., Belew A. T., Islam E., Berge J., et al. McIver K. S. The Transcriptional Regulator CpsY Is Important for Innate Immune Evasion in Streptococcus pyogenes. Infection and Immunity. 2017 March;85(3):e00925–16. [PMC free article: PMC5328483] [PubMed: 27993974]
- Vyas H. K., Proctor E.-J., McArthur J., Gorman J., Sanderson-Smith M. Current Understanding of Group A Streptococcal Biofilms. Current Drug Targets. 2019;20(9):982–993. [PMC free article: PMC6700754] [PubMed: 30947646]
- Wilkening R. V., Chang J. C., Federle M. J. PepO, a CovRS-controlled endopeptidase, disrupts Streptococcus pyogenes quorum sensing. Molecular Microbiology. 2016 January;99(1):71–87. [PMC free article: PMC4987125] [PubMed: 26418177]
- Zhu L., Olsen R. J., Horstmann N., Shelburne S. A., Fan J., Hu Y., Musser J. M. Intergenic Variable-Number Tandem-Repeat Polymorphism Upstream of rocA Alters Toxin Production and Enhances Virulence in Streptococcus pyogenes. Infection and Immunity. 2016 June 23;84(7):2068–2093. [PMC free article: PMC4936349] [PubMed: 27141081]
- Abstract
- Introduction
- Mechanisms of regulation
- Master regulators/Virulence regulator control
- Metabolite responsive regulators
- Amino acid and nitrogen availability (Rsh, CodY, and Rny)
- Virulence-related metabolic regulators
- Host environment responsive regulators
- Alternative Mechanisms of Gene Regulation
- Overall Perspectives
- References
- Review Virulence-Related Transcriptional Regulators of Streptococcus pyogenes.[Streptococcus pyogenes: Basic ...]Review Virulence-Related Transcriptional Regulators of Streptococcus pyogenes.Vega LA, Malke H, McIver KS. Streptococcus pyogenes: Basic Biology to Clinical Manifestations. 2016
- Antagonistic Rgg regulators mediate quorum sensing via competitive DNA binding in Streptococcus pyogenes.[mBio. 2013]Antagonistic Rgg regulators mediate quorum sensing via competitive DNA binding in Streptococcus pyogenes.Lasarre B, Aggarwal C, Federle MJ. mBio. 2013 Jan 2; 3(6). Epub 2013 Jan 2.
- Review Stand-alone response regulators controlling global virulence networks in streptococcus pyogenes.[Contrib Microbiol. 2009]Review Stand-alone response regulators controlling global virulence networks in streptococcus pyogenes.McIver KS. Contrib Microbiol. 2009; 16:103-119. Epub 2009 Jun 2.
- Inactivation of the Rgg2 transcriptional regulator ablates the virulence of Streptococcus pyogenes.[PLoS One. 2014]Inactivation of the Rgg2 transcriptional regulator ablates the virulence of Streptococcus pyogenes.Zutkis AA, Anbalagan S, Chaussee MS, Dmitriev AV. PLoS One. 2014; 9(12):e114784. Epub 2014 Dec 8.
- Review Virulence factor regulation and regulatory networks in Streptococcus pyogenes and their impact on pathogen-host interactions.[Trends Microbiol. 2003]Review Virulence factor regulation and regulatory networks in Streptococcus pyogenes and their impact on pathogen-host interactions.Kreikemeyer B, McIver KS, Podbielski A. Trends Microbiol. 2003 May; 11(5):224-32.
- Virulence-Related Transcriptional Regulators of Streptococcus pyogenes - Strepto...Virulence-Related Transcriptional Regulators of Streptococcus pyogenes - Streptococcus pyogenes: Basic Biology to Clinical Manifestations
Your browsing activity is empty.
Activity recording is turned off.
See more...