This work is licensed under a Creative Commons Attribution-NonCommercial-NoDerivatives 4.0 International License.
NCBI Bookshelf. A service of the National Library of Medicine, National Institutes of Health.
Imagine the future of energy. The future might look like a new power plant on the edge of town—an inconspicuous biore-actor that takes in yard waste and locally-grown crops like corn and wood chips and churns out electricity to area homes and businesses. Or the future may take the form of a stylish-looking car that refills its tank at hydrogen stations. Maybe the future of energy looks like a device on the roof of your own home – a small appliance, connected to the household electric system, that uses sunlight and water to produce the electricity that warms your home, cooks your food, powers your television, and washes your clothes.
All these futuristic energy technologies may become reality some day, thanks to the work of the smallest living creatures on earth: microorganisms. “Microbial energy conversion” is the shorthand term for technologies like these. In microbial energy technologies, microorganisms make fuels out of raw organic materials, thereby converting the chemical energy in the biomass into chemical energy in the form of ethanol or hydrogen, for example. In addition, microbes can convert solar energy to hydrogen. Those fuels are then burned to make electrical energy or, in the case of internal combustion engines, kinetic energy to power a car. Another technology that falls under the heading of microbial energy conversion is the microbial fuel cell, a bioreactor in which bacteria transform the chemical energy in biomass directly into electrical energy.
The world faces a potentially crippling energy crisis in the next 30 to 50 years. Global populations are climbing, driving an ever-increasing demand for energy to power manufacturing, transportation, heat, and other needs.
The world faces a potentially crippling energy crisis in the next 30 to 50 years. Global populations are climbing, driving an ever-increasing demand for energy to power manufacturing, transportation, heat, and other needs. “World energy consumption is projected to increase by 71 percent from 2003 to 2030” (Energy Information Administration/International Energy Outlook 2006). Petroleum, the foundation of the current transportation system, peaked in production in the U.S. in the mid 1980s, and world production is projected to peak in the next 25 to 50 years. Moreover, the burning of fossil fuels and the resulting release of carbon dioxide and combustion pollutants has brought about global climate change, the effects of which we are only beginning to understand. The means of preventing the twin catastrophes of energy scarcity and environmental ruin is not clear, but one part of the solution may lie in microbial energy conversion.
The American Academy of Microbiology convened a colloquium March 10-12, 2006, in San Francisco, California, to discuss the production of energy fuels by microbial conversions. The status of research into various microbial energy technologies, the advantages and disadvantages of each of these approaches, research needs in the field, and education and training issues were examined, with the goal of identifying routes for producing biofuels that would both decrease the need for fossil fuels and reduce greenhouse gas emissions. Colloquium participants made a number of recommendations for moving forward with research and education in this field.
Microorganisms may be used to generate a number of fuels, including ethanol, hydrogen, methane, lipids, and butanol, among which ethanol production is the most mature technology. As a liquid, ethanol is relatively easy to store and it can fit into the existing fuel infrastructure. However, ethanol adsorbs water readily and cannot be shipped through common-carrier pipelines, which inevitably contain water. Processes that generate higher molecular weight alcohols, such as butanol, can be produced with similar technologies and are more compatible with existing infrastructure. Currently, the production of ethanol from the most abundant forms of biomass, namely cellulose and lignocellulose, is comparatively difficult and expensive. Future research in alcohol production needs to focus on increasing the productivity and yield of processes that make alcohol from biomass and processes that generate alternative alcohols.
Hydrogen, a potent energy carrier, may be produced in any of a variety of ways. Oxygenic photosynthesis in cyanobacteria and other microbes can be harnessed to make hydrogen from water in a promising technology that may meet energy needs in the long term. The resources needed for this process, water and sunlight, are in practically unlimited supply, but the efficiency of the process is low. We need new photobioreactor designs to help increase efficiency of light capture and hydrogen removal in these systems. Hydrogen also may be produced using the cellular machinery of nitrogen fixation, through fermentation of biomass, through iron metabolism in photosynthesis, by metabolizing carbon storage compounds, or by microbial mats.
Methane is another useful microbially-produced fuel. Methano-genesis is a relatively simple, predictable microbial process, and methane fits into the infrastructure in place of natural gas. Although methane production is a well-explored area, much of the current data have derived from operations optimized for waste disposal, not for methane generation. Research in methane production should focus on optimizing the productivity of methanogenic microbial communities and bioreactor design.
The study of microbial fuel cells is in its infancy, and yield and current density are low in today's systems, but the potential to make great leaps of progress in yield and performance is great. Research in microbial fuel cells should focus, in part, on the discovery and development of novel bacteria capable of transferring electrons from biomass substrates to an electrode.
Overarching research needs in the field include bioprospecting, the search for novel microorganisms and genes that can aid in energy conversion. Research is also needed to explore the dynamics of microbial communities, enzymology, the biology of non-growing cells, modeling, genomics, nanotechnology, new microbiological techniques, and bioreactor engineering.
Specific education and training in microbial energy conversion technologies are needed to provide the foundation for insights that will allow major increases in biofuel generation. We need our brightest and best minds to meet the challenges of this multidisciplinary effort.
Front Matter
The American Academy of Microbiology is the honorific leadership group of the American Society for Microbiology. The mission of the American Academy of Microbiology is to recognize scientific excellence and foster knowledge and understanding in the microbiological sciences. The Academy strives to include underrepresented scientists in all its activities.
The American Academy of Microbiology is grateful for the generosity of the following for support of this project:
- U.S. Department of Energy (Office of Science (BER), Grant No. DE-FG02-06ER64162)
- U.S. Army Research Office
- U.S. Department of Agriculture (Award No. 2006-35504-16709)
- National Science Foundation (Grant No. MCB-0544950)
- Cargill, Inc.
- Genomatica, Inc.
The opinions expressed in this report are those solely of the colloquium participants and do not necessarily reflect the official positions of our sponsors or the American Society for Microbiology.
BOARD OF GOVERNORS, AMERICAN ACADEMY OF MICROBIOLOGY
R. John Collier, Ph.D. (Chair), Harvard University Medical School
Kenneth I. Berns, M.D., Ph.D., University of Florida Genetics Institute
Arnold L. Demain, Ph.D., Drew University
E. Peter Greenberg, Ph.D., University of Washington
Carol A. Gross, Ph.D., University of California, San Francisco
J. Michael Miller, Ph.D., Centers for Disease Control and Prevention
Stephen A. Morse, Ph.D., Centers for Disease Control and Prevention
Harriet L. Robinson, Ph.D., Emory University
George F. Sprague, Jr., Ph.D., University of Oregon
David A. Stahl, Ph.D., University of Washington
Judy A. Wall, Ph.D., University of Missouri-Columbia
COLLOQUIUM STEERING COMMITTEE
Judy A. Wall, Ph.D., (Chair), University of Missouri-Columbia
Lonnie O. Ingram, Ph.D., (Co-Chair), University of Florida
Jean E. Brenchley, Ph.D., The Pennsylvania State University
Caroline S. Harwood, Ph.D., University of Washington
Lee Lynd, D.E., Dartmouth College
Carol A. Colgan, Director, American Academy of Microbiology
SPONSOR REPRESENTATIVES
Parag R. Chitnis, Ph.D., National Science Foundation
Michael L. Knotek, Ph.D., U.S. Department of Energy
Sherry Tove, Ph.D., U.S. Army Research Office
Sharlene Weatherwax, Ph.D., U.S. Department of Energy
COLLOQUIUM PARTICIPANTS
Birgitte Ahring, Ph.D., Technical University of Denmark
Frances Arnold, Ph.D., California Institute of Technology
Hans Blaschek, Ph.D., University of Illinois
Brian H. Davison, Ph.D., Oak Ridge National Laboratory
Arnold L. Demain, Ph.D., Drew University
Roy Doi, Ph.D., University of California, Davis
Douglas Eveleigh, Ph.D., Rutgers University
J. Gregory Ferry, Ph.D., The Pennsylvania State University
Yuri A. Gorby, Ph.D., Pacific Northwest National Laboratory
Gerhard Gottschalk, Sc.D., Institut fur Mikrobiologie und Genetics, Goettingen, Germany
Barbel Hahn-Hagerdal, Ph.D., Lund University, Lund, Sweden
Mark T. Holtzapple, Ph.D., Texas A&M University
Peter Lindblad, Ph.D., Uppsala University, Villavagen, Sweden
Derek Lovley, Ph.D., University of Massachusetts-Amherst
Paul W. Ludden, Ph.D., University of California, Berkeley
Julie A. Maupin-Furlow, Ph.D., University of Florida
Kenneth H. Nealson, Ph.D., University of Southern California
Dianne K. Newman, Ph.D., California Institute of Technology
Nancy Nichols, Ph.D., U.S. Department of Agriculture, Peoria, Illinois
John Reeve, Ph.D., Ohio State University
John Saddler, Ph.D., University of British Columbia, Vancouver, Canada
Bernhard Schink, Sc.D., University of Konstanz, Germany
Michael Seibert, Ph.D., National Renewal Energy Laboratory, Golden, Colorado
Alfred Spormann, Ph.D., Stanford University
Joseph M. Suflita, Ph.D., University of Oklahoma
Robert F. Tabita, Ph.D., Ohio State University
Larry P. Walker, Ph.D., Cornell University
Paul J. Weimer, Ph.D., University of Wisconsin, Madison
William Whitman, Ph.D., University of Georgia
Ann C. Wilkie, Ph.D., University of Florida
EXECUTIVE SUMMARY
Imagine the future of energy. The future might look like a new power plant on the edge of town—an inconspicuous biore-actor that takes in yard waste and locally-grown crops like corn and wood chips and churns out electricity to area homes and businesses. Or the future may take the form of a stylish-looking car that refills its tank at hydrogen stations. Maybe the future of energy looks like a device on the roof of your own home – a small appliance, connected to the household electric system, that uses sunlight and water to produce the electricity that warms your home, cooks your food, powers your television, and washes your clothes.
All these futuristic energy technologies may become reality some day, thanks to the work of the smallest living creatures on earth: microorganisms. “Microbial energy conversion” is the shorthand term for technologies like these. In microbial energy technologies, microorganisms make fuels out of raw organic materials, thereby converting the chemical energy in the biomass into chemical energy in the form of ethanol or hydrogen, for example. In addition, microbes can convert solar energy to hydrogen. Those fuels are then burned to make electrical energy or, in the case of internal combustion engines, kinetic energy to power a car. Another technology that falls under the heading of microbial energy conversion is the microbial fuel cell, a bioreactor in which bacteria transform the chemical energy in biomass directly into electrical energy.
The world faces a potentially crippling energy crisis in the next 30 to 50 years. Global populations are climbing, driving an ever-increasing demand for energy to power manufacturing, transportation, heat, and other needs.
The world faces a potentially crippling energy crisis in the next 30 to 50 years. Global populations are climbing, driving an ever-increasing demand for energy to power manufacturing, transportation, heat, and other needs. “World energy consumption is projected to increase by 71 percent from 2003 to 2030” (Energy Information Administration/International Energy Outlook 2006). Petroleum, the foundation of the current transportation system, peaked in production in the U.S. in the mid 1980s, and world production is projected to peak in the next 25 to 50 years. Moreover, the burning of fossil fuels and the resulting release of carbon dioxide and combustion pollutants has brought about global climate change, the effects of which we are only beginning to understand. The means of preventing the twin catastrophes of energy scarcity and environmental ruin is not clear, but one part of the solution may lie in microbial energy conversion.
The American Academy of Microbiology convened a colloquium March 10-12, 2006, in San Francisco, California, to discuss the production of energy fuels by microbial conversions. The status of research into various microbial energy technologies, the advantages and disadvantages of each of these approaches, research needs in the field, and education and training issues were examined, with the goal of identifying routes for producing biofuels that would both decrease the need for fossil fuels and reduce greenhouse gas emissions. Colloquium participants made a number of recommendations for moving forward with research and education in this field.
Microorganisms may be used to generate a number of fuels, including ethanol, hydrogen, methane, lipids, and butanol, among which ethanol production is the most mature technology. As a liquid, ethanol is relatively easy to store and it can fit into the existing fuel infrastructure. However, ethanol adsorbs water readily and cannot be shipped through common-carrier pipelines, which inevitably contain water. Processes that generate higher molecular weight alcohols, such as butanol, can be produced with similar technologies and are more compatible with existing infrastructure. Currently, the production of ethanol from the most abundant forms of biomass, namely cellulose and lignocellulose, is comparatively difficult and expensive. Future research in alcohol production needs to focus on increasing the productivity and yield of processes that make alcohol from biomass and processes that generate alternative alcohols.
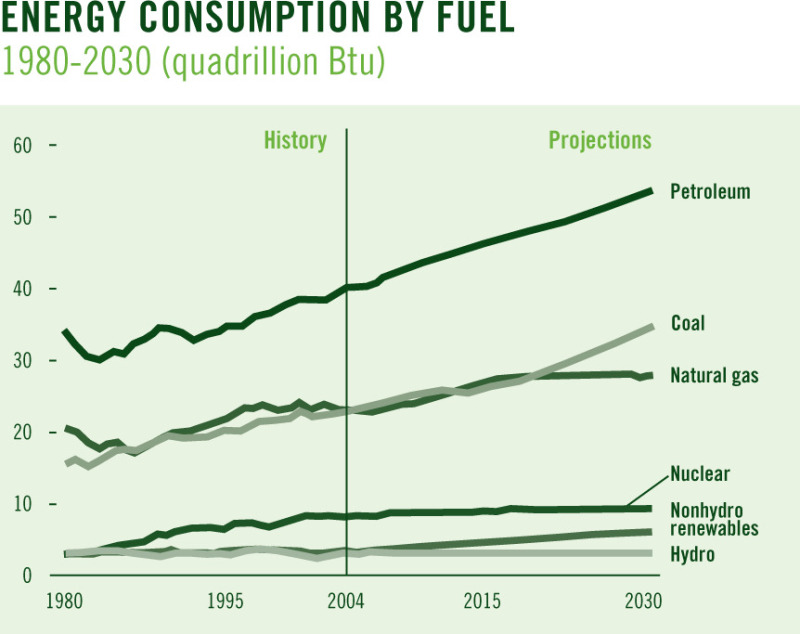
(Energy Information Administration/Annual U.S. Energy Outlook 2006)

(Energy Information Administration/Annual U.S. Energy Outlook 2006)
Hydrogen, a potent energy carrier, may be produced in any of a variety of ways. Oxygenic photosynthesis in cyanobacteria and other microbes can be harnessed to make hydrogen from water in a promising technology that may meet energy needs in the long term. The resources needed for this process, water and sunlight, are in practically unlimited supply, but the efficiency of the process is low. We need new photobioreactor designs to help increase efficiency of light capture and hydrogen removal in these systems. Hydrogen also may be produced using the cellular machinery of nitrogen fixation, through fermentation of biomass, through iron metabolism in photosynthesis, by metabolizing carbon storage compounds, or by microbial mats.
Methane is another useful microbially-produced fuel. Methano-genesis is a relatively simple, predictable microbial process, and methane fits into the infrastructure in place of natural gas. Although methane production is a well-explored area, much of the current data have derived from operations optimized for waste disposal, not for methane generation. Research in methane production should focus on optimizing the productivity of methanogenic microbial communities and bioreactor design.
The study of microbial fuel cells is in its infancy, and yield and current density are low in today's systems, but the potential to make great leaps of progress in yield and performance is great. Research in microbial fuel cells should focus, in part, on the discovery and development of novel bacteria capable of transferring electrons from biomass substrates to an electrode.
Overarching research needs in the field include bioprospecting, the search for novel microorganisms and genes that can aid in energy conversion. Research is also needed to explore the dynamics of microbial communities, enzymology, the biology of non-growing cells, modeling, genomics, nanotechnology, new microbiological techniques, and bioreactor engineering.
Specific education and training in microbial energy conversion technologies are needed to provide the foundation for insights that will allow major increases in biofuel generation. We need our brightest and best minds to meet the challenges of this multidisciplinary effort.
INTRODUCTION
Microbial energy technologies employ microorganisms either to manufacture fuels or to generate electricity directly through the breakdown of organic materials. Energy is never actually produced; rather, it can be converted from one form to another. In many microbial energy conversions, microorganisms transform the chemical energy in biomass into energy-bearing fuels. In contrast, microbial fuel cells use microbes to harvest electrons from bio-mass to generate an electric current, thereby transforming chemical energy into electrical energy.
PROS AND CONS OF MICROBIAL ENERGY CONVERSION
Microbial energy conversion has several advantages over petroleum- and natural gas-based energy. Primary among these benefits is the fact that the processes involved in microbial energy transformations are more environmentally friendly than fossil fuel technologies. For example, microbial energy modes do not involve the use or production of hazardous materials. More importantly, all of the microbial energy transformation processes described here, except for photosynthetic hydrogen generation, involve the conversion of biomass into chemical or electrical energy. Biomass conversion is a “carbon-neutral” process, meaning potentially it neither increases nor decreases the total amount of carbon dioxide (an important greenhouse gas) in the atmosphere.
Microorganisms are also capable of selecting their substrate from among a complex mixture of chemicals, an ability that minimizes the need to purify or refine the raw materials to separate substrates from non-substrates. Finally, microbial energy technologies can be used to produce energy locally, close to where the energy is needed. “Distributed” energy systems like these can minimize the cost of energy transfer, a big consideration where energy needs are small.
Although biology is to thank for many of the advantages of using microorganisms in energy conversion, biology is also behind many of the drawbacks to using these technologies. Many of the microbial strains and enzymes used to produce fuels, for example, are sensitive to the presence of their products, so there is often a need for physical separation of intermediates or products from the reaction center in order to drive the process forward. Hydrogenases and nitrogenases, which produce hydrogen (a potentially very powerful biofuel), are extremely sensitive to oxygen. Hence, hydrogen-generating bioreactors must be kept under strictly anoxic conditions, a state that is not always easy to maintain.
MEETING THE WORLD'S ENERGY NEEDS
Although the field is promising, microbial energy conversion cannot possibly meet all of the world's diverse energy needs. Different applications can call for radically different solutions. Microbial sources of energy may fit into some niches (depending on the amount of energy needed and the locale), but it is necessary to make a wide variety of sound energy technologies available to decision makers.
Currently, the choices for providing energy are limited. Policy makers and the research community must begin to pursue a broader array of potential energy technologies. A diverse energy portfolio that includes an assortment of microbial energy choices will allow communities and consumers to select the best energy solution for their own particular needs.
Funding agencies and governments alike need to prepare for future energy needs by investing both in the microbial energy technologies that work today and in the untested technologies that will serve the world's needs tomorrow. More mature bio-processes, such as ethanol production from starchy materials and methane from waste digestors, will find applications in the short term. However, innovative techniques for liquid fuel or biohydrogen production are among the longer term possibilities that should also be vigorously explored, starting now.
KEY MICROBIAL ENERGY CONVERSION PATHS
Microorganisms can help meet human energy needs in any of a number of ways. In their most obvious role in energy conversion, microorganisms can generate fuels, including ethanol, hydrogen, methane, lipids, and butanol, which can be burned to produce energy. Alternatively, bacteria can be put to use in microbial fuel cells, where they carry out the direct conversion of biomass into electricity. Microorganisms may also be used some day to make oil and natural gas technologies more efficient by sequestering carbon or by assisting in the recovery of oil and natural gas from the subsurface.
BIOETHANOL
When manufactured from crop sources, like corn or wood, ethanol is commonly referred to as “bioethanol.” Theoretically, bioethanol production should be able to yield 0.5 g of ethanol per gram of raw biomass, which translates into an energy recovery of approximately 90%. Current technologies recover roughly 60% of the energy in raw biomass.
Bioethanol is flexible; it can be manufactured from any of a number of different substrates (including starch and lignocellu-lose), by a selection of different microorganisms, and in a variety of different engineered circumstances. Corn is a common substrate for bioethanol manufacture because the process is relatively free of technical obstacles and because, in the U.S. at least, the use of corn as a substrate for energy production is subsidized by the federal government. Microorganisms are involved in the transformation of corn to bioethanol in two ways: they catalyze the hydrolysis of starches using amylases and amyloglucosidases, and they ferment the resulting sugars to bioethanol. The fermentation step is generally carried out by yeast, but certain strains of bacteria, including Zymomonas mobilis and recombinant strains of Escherichia coli and Klebsiella oxytoca, are also capable of producing high yields of bioethanol.
Lignocellulose includes such diverse sources as switchgrass, corn stalks, and wood chips. Fungal enzymes and fermentative yeasts are then used to transform lignocellulose first to sugars and then to bioethanol. The low lignocellulose reactivity limits the production of bioethanol, and as a result, the concentration of bioethanol in a lignocellulose reactor does not reach the high concentrations seen in corn reactors. Hence, bioethanol-producing microbes in corn-based reactor systems must be tolerant of higher ethanol concentrations than microbes in lignocellulose reactors.
Consolidated bioprocessing (CBP) systems, in which anaerobic bacteria produce their own hydrolytic enzymes and ferment the polysaccharide oligomers, also can be used to produce bioethanol. CPB systems bypass the enzyme extraction step, thereby theoretically enhancing the efficiency of bioethanol production. Recent successes in understanding the genetics of the cellulose fermenter Clostridium thermocellum, which is often used in CPB systems, are encouraging. Low yield, low bioethanol concentration, low volumetric productivity, low product tolerance in the bacteria, growth medium requirements, and intractable genetics still pose difficulties in applying CPB, however.
The MixAlco process1 is another promising technology for mixed alcohol production. MixAlco may be used to convert any biodegradable material into a mixture of carboxylate salts, which may be chemically converted to primary or secondary alcohols and other valuable products, including carboxylic acids, ketones, esters, ethers, and aldehydes. For any given product, the molecular weight distribution may be influenced by adjusting the reaction parameters. The substrate, which can range from municipal waste to cattle manure to energy crops, is pretreated with lime and oxygen to increase its digestibility and then fed into a mixed culture fermenter. Currently, the pilot plant is digesting paper waste and manures, but pretreatment can enable the use of more recalcitrant biomass. The solids retention time for MixAlco, a measure of how long it takes for a material to be completely digested, varies from 20 days to three months. The system has been operated at a pilot scale (8,000 liters), and it is claimed that the process can generate roughly 86 gallons of mixed secondary alcohols or 125 gallons of mixed primary alcohols per dry ton of biomass. Under anaerobic conditions, the products of MixAlco are stable and may be stored or shipped to a central processing facility. The culture used for fermentation has yet to be thoroughly described, but it is known from clone libraries that it is composed of roughly 20 species, half of which have been previously described.
In a method known as the Gaddy process (patented process developed by James Gaddy of Fayetteville, Arkansas), carbon monoxide gas derived from biomass is converted to bioethanol by a proprietary culture of microorganisms, in which Clostridium ljungdahlii, plays a central role. The cost of introducing gas into the liquid culture and extracting the bioethanol is high, but as refinements have been implemented, the process has become more cost-effective and may be as low as $0.80 per gallon of bioethanol.
Bioethanol is flexible: it can be manufactured from any of a number of different substrates (including starch and lignocellulose), by a selection of different microorganisms, and in a variety of different engineered circumstances.
Advantages
Bioethanol is a versatile fuel with established protocols for manufacture and use. Because it is a liquid, bioethanol fits into the current fuel infrastructure, although transport requires special handling to prevent water accumulation. Bioethanol can be cost-competitive with petroleum, but given the amount of research and development that has gone into ethanol-yielding processes to date, there may not be much room for improvement in yield and efficiency of bioethanol production. Hence, progress in bioethanol-related technology in the future likely will be incremental.
Disadvantages and Technical Difficulties
Although bioethanol production has been successful in a number of different respects, technical issues have arisen in efforts to produce bioethanol at a commercial scale. Unsatisfactory yield has been one problem. Efforts should be made to change the efficiency of the bioethanol reaction in order to improve yields.
Using solid substrates in converting lignocellulose to sugars poses another dilemma in bioethanol production. The substrate availability in solid materials is quite low in comparison to liquid materials, a limitation that constricts the rates and yields. It may be possible to use decrystallizing enzymes or cellulosomes (enzyme complexes that degrade cellulose) to open the structures of the organic material and make the cellulose more available to microorganisms.
Cellulose and lignocellulose are in much greater supply than starch and sugars and are, therefore, preferred substrates for ethanol production. However, producing ethanol from cellulose and lignocellulose is comparatively difficult and expensive. A number of challenges must be overcome in order to make the conversion of cellulose and lignocellulose to ethanol more cost-competitive, including:
- Optimizing different biomass pretreatment processes for different substrates,
- Engineering more efficient enzymes for lignocellulose and hemicellulose degradation,
- Developing more efficient fermenting organisms, and
- Reducing the cost of producing cellulose- and lignocellulose-degrading enzymes.
The expense of enzyme production is the biggest economic barrier to lignocellulose conversion to bioethanol. Fungal cellulases and beta-glucosidases produced in separate aerobic reactors can be extracted in very high yields, but because these enzymes have low specific activities, they must be used in large quantities to achieve lignocellulose conversion. A great deal of effort and funding has been directed toward improving the specific activity of these enzymes in order to increase the cost-effectiveness of lignocellulose conversion to bioethanol, but this work has failed to achieve significant success.
Pretreated wood may contain phenolics, furan compounds, and short-chain fatty acids that are inhibitory to fermenting microorganisms. Hence, wood products usually are subjected to a costly detoxification step prior to fermentation. Microorganisms that can tolerate these inhibitory compounds do exist, however, so it should be possible to avoid having to remove inhibitors.
Ethanol adsorbs water readily and cannot be shipped through common-carrier pipelines, which inevitably contain water. Ethanol currently is shipped by truck or rail and splash blended at the terminal, which adds a great deal of cost. Higher molecular weight alcohols, such as butanol, are more compatible with the existing liquid fuel infrastructure.
Research Needs
Progress in bioethanol production is served best by moving forward with pilot plant studies of the enzymes and microorganisms in use today while continually exploring the development of new biocatalysts. New enzymes and microbes should be targeted to bridge specific technology gaps in order to enhance productivity and reduce the cost of bioethanol production.
Pilot plant studies are also needed to compare currently available strategies for cellulose and hemicellulose conversion to bioethanol. A systematic comparison will enable an unbiased assessment of the advantages and disadvantages of each conversion system.
There is a pronounced need to study and understand the biology of the microorganisms currently used in bioethanol production. For example, mechanisms of product toxicity in microbes used in consolidated bioprocessing systems, like Clostridium thermocellum, require further research. The enzymology of biomass depolymerization (the breakdown of recalcitrant, multi-unit compounds into smaller compounds) also requires intensive study in order to optimize this process.
The possibility of making improvements in the microorganisms currently used in bioethanol production, including increasing their substrate ranges or introducing cellulases into their cells or onto their surfaces, should be explored.
It is also important to plan for the future by developing new ethanologens, thereby expanding the portfolio of organisms and enzymes that could reduce costs and enhance the efficiency of bioethanol production. For example, it may be possible to engineer or develop naturally occurring thermophilic bacteria that produce ethanol. Thermophiles that contain pyruvate decarboxylases, which could increase ethanol yield and decrease the toxicity of the end products to the bacteria, would be particularly useful in bioethanol production. The possibility of using anaerobic fungi as sources of novel cellulases should also be explored.
Development of a “super bug,” an organism that makes all of the required enzymes for cellulose and hemicellulose degradation and ferments the products into ethanol, is an appealing idea. However, the various biomass substrates for ethanol production vary so greatly that no single organism can be a solution for all feedstocks.
Biomass pretreatment, which is performed to release ethanol-yielding substances from raw biomass, is often harsh on the enzymes and microorganisms that carry out the ethanol fermentation. There is a need to increase the compatibility between biomass pretreatment methods, hydrolytic enzymes, and biocatalysts. Broad comparative data in this area, including comparisons between the various hydrolytic enzymes, would be helpful in addressing these technology gaps.
HYDROGEN
The greatest advantage of hydrogen over other microbially produced energy carriers lies in its flexibility; it can be produced in a number of different ways by many different organisms. Storage and distribution of hydrogen are problematic, however. Development of a liquid fuel with a high hydrogen content could solve some storage and transport issues, allowing the use of hydrogen to mesh with the distribution technology and infrastructure already in place today.
A variety of approaches should be explored to advance the long-term goal of efficient microbial hydrogen production. Hydrogen production by cyanobacteria, anoxygenic photosynthetic bacteria, and green algae need to be explored, as does the potential for hydrogen production in organisms yet to be discovered. Metabolic engineering of hydrogen production in known microbes should also be researched. New genomics and genetic exchange tools are needed to fully realize the possibilities of engineering and optimizing hydrogen production in microorganisms.
Coupling Oxygenic Photosynthesis and Hydrogenases
With the aid of the sun and CO2, cyanobacteria, microalgae, and macroalgae all produce biomass by the metabolic pathway known as “photosystem II.” In the absence of CO2, some of these photosynthetic organisms can produce hydrogen. Employing oxygenic photosynthesis to produce hydrogen on a commercial scale has great potential in the long term, but there are also technical difficulties and disadvantages to using the process.
Development of a liquid fuel with a high hydrogen content could solve some storage and transport issues, allowing the use of hydrogen to mesh with the distribution technology and infrastructure already in place today.
Advantages
The resources necessary to make hydrogen phototrophically, namely water and sunlight, are virtually unlimited. Moreover, the process does not rely on the use of biomass, reduced sulfur compounds, or reduced iron, as do other modes of microbial hydrogen production. Aquatic phototrophic microorganisms are the preferred organisms for making hydrogen, since they produce a lignin-free biomass that is easily digested under anaerobic conditions, making waste handling a relatively simple matter. Since exploration of this field has begun relatively recently, opportunities for improving phototrophic hydrogen production are numerous, and there is the potential to make substantial gains in efficiency and yield.
Disadvantages and Technical Difficulties
Some technical difficulties with using oxygenic phototrophic hydrogen production as a mode of energy conversion include inefficiencies of light capture in bioreactor systems and inefficiencies in the water splitting/manganese chemistry involved. Hydrogen-producing phototrophs currently extract light at about 0.1% efficiency in bioreactors. Methods to enhance penetration of solar energy to the photosynthetic machinery of the cell are needed to improve bioreactor efficiency and to facilitate expansion of these systems for industrial bioreactors. Film covers for photobioreactors that can cover thousands of square kilometers are durable, light-transparent, resistant to ultraviolet radiation, and gas impermeable are also needed.
Research Needs
Hydrogenases, the proteins that generate hydrogen, are inhibited by even very small quantities of hydrogen, so hydrogen must be removed from the system as soon as it is created in order for the process to continue efficiently. New and improved techniques for accomplishing hydrogen removal are needed and may require the involvement of materials scientists. Hydrogenases are also damaged by the other product of oxygenic photosynthesis, oxygen. It may be possible to optimize hydrogen production by employing naturally oxygen-tolerant hydrogenases like iron hydrogenases for the purpose, but further work is required to understand these enzymes and to enhance their electron turnover. The products of phototrophic hydrogen evolution, hydrogen and oxygen, are explosive. The design of the reactors used for this purpose needs to be improved to overcome these inefficiencies and hazards.
Photobioreactor development is also needed. New bioreactors should be designed modularly to enable creation of other value-added products by the photosystem. For example, a well-designed photobioreactor could be made into a microbial fuel cell, directing electrons from the photosystem to make electricity instead of hydrogen.

(“Worldwide Look at Reserves and Production,” Oil and Gas Journal, vol. 47 (Dec. 19, 2005), pp. 24-25.)

(History: Energy Information Administration, International Energy Annual (IEA) 2003 (May-July 2005), website http://www.eia.doe.gov/iea/. Projections: IEA, System for the Analysis of Global Energy Markets (2006).)
Other research needs with respect to photosynthetic hydrogen generation include:
- Detailed molecular investigations of the processes involved,
- Research into the possibility of switching biofilm systems “on” and “off,”
- Research into the thermodynamic limitations of the system, including the limits on photosynthetic biomass and hydrogen production,
- Research into improving the poor photosynthetic efficiency of phototrophic organisms,
- Research into the creation and utilization of possible co-products made by phototrophic bacteria, and
- Studies on the function of natural rhodopsin-based systems and their potential utility as a bio-based photosynthetic system for energy production.
Hydrogen Generation through Nitrogenase
In the presence of nitrogen, nitrogenases catalyze the production of hydrogen and ammonia. In the absence of nitrogen, nitrogenases will form hydrogen exclusively in a process that requires tremendous amounts of chemical energy (in the form of ATP formed by harvesting light energy) and electrons from bio-mass or certain inorganic compounds. Without a nitrogen source, and under non-growing conditions, the efficiency of the conversion of electrons to hydrogen can reach close to 100%. So long as electrons are in good supply, this process is very stable and can continue for two weeks.2
Advantages
Hydrogen generation from nitrogenases is a natural process that does not require much engineering or manipulation to produce hydrogen successfully. Nitrogenase hydrogen production is essentially irreversible and not inhibited by hydrogen, as opposed to hydrogenases. That said, the process has not been subjected to much investigation, so it is possible that improvements in the efficiency and speed of the process could be made.
Disadvantages and Technical Difficulties
On the down side, the low efficiency of light capture in bioreactor systems that convert light energy to ATP for hydrogen generation by nitrogenases is a problem. Moreover, extraction of electrons from biomass could be a rate-limiting step in this process, but it may be possible to circumvent this limitation by using inorganic compounds as electron donors. This possibility needs to be explored further. Nitrogenases are not fast-working enzymes; they transform electrons into hydrogen relatively slowly.
Research Needs
Research needs with respect to hydrogen production by nitrogenases include biocatalyst discovery, research into possible electron donors for the process, and bioreactor design. Nitrogen fixation is carried out by a diverse array of microorganisms, but the hydrogen-producing capabilities of most of these organisms and of several types of nitrogenase enzymes remain to be explored. A recently discovered nitrogenase from a species of Streptomyces, which has a molybdenum cofactor and is more oxygen resistant than other nitrogenases, may be an efficient hydrogen producer. Metagenomic searches could help in the discovery of other, better nitrogenases, and it may be possible to find more tractable nitrogen-fixing organisms that are both easy to cultivate and economically viable. Very few organisms with alternative nitrogenases have been isolated, but the enrichment of new isolates should not be technically difficult. Alternatively, it may be advisable to engineer known organisms to increase hydrogen production rates, create a more stable enzyme, or enable the use of a broader spectrum of substrates.
The bioreactors used for hydrogen production by nitrogen-fixing organisms require improvements in order to increase hydrogen yield and reduce microbial contamination problems. The possibility of employing nitrogen fixers that use inorganic compounds (including reduced iron, hydrogen sulfide, thiosulfate, and elemental sulfur) as electron donors for hydrogen production should be explored. In the long term, inorganic substances are the preferred electron source for hydrogen production by nitrogenases because they are more abundant than biomass substrates and their use allows hydrogen production in the absence of carbon substrates, which is a benefit in view of the overabundance of carbon dioxide in the atmosphere.
When water is used as an electron donor, hydrogen production also generates oxygen, which can damage oxygen-sensitive nitrogenases. The combination of oxygen and hydrogen presents another problem: it is highly explosive and could pose a serious safety hazard. It may be possible to use an oxygen-consuming organism in coculture with the nitrogen fixer to sap the oxygen as it is created and spare the hydrogen. Alternatively, organisms like Trichodesmium, which produces hydrogen and oxygen at different times, could be employed to avoid the problem of having oxygen and hydrogen present simultaneously.
Fermentative Hydrogen Production
Fermentative hydrogen production, in which glucose is converted to carbon dioxide and hydrogen, could be a viable energy opportunity, but it has yet to be explored in detail. One advantage of using fermentation to produce hydrogen is that this process is technically easier to manage compared to processes that rely on light reactions. However, the hydrogen yield of fermentation is strictly limited. Only one third of the electrons shuttled through fermentation end with hydrogen; the rest of the potential energy is lost in the form of acetate or other fermentation products.
Hydrogen Production through the Iron Cycle
Many species of bacteria, including Rhodobacter SW2, Chlorobium species, and Rhodopseudomonas palustris, are capable of using iron as an electron donor for photosynthesis. In theory, it should be possible to direct these electrons to hydrogen production, although this has not yet been demonstrated.
Some bacteria that carry out iron oxidation and photosynthesis do not couple them to growth. If iron processing by this route were harnessed for hydrogen production purposes, a second organism could conceivably be put to work in converting iron back to its reduced state. In theory, this partnership would complete the iron cycle, although reduced iron would likely have to be replenished from time to time. This mode of energy production has the advantage of a practically unlimited supply of easily accessible iron. In addition, although difficulties would likely arise in precipitating and removing oxidized iron from the system, citrate and other iron chelators could accelerate the process. Providing an appropriate source of reductant for the second organism for regeneration of the reduced iron might also limit the overall process.
Hydrogen from Carbon Storage Compounds
Cyanobacteria accumulate stores of carbon in daylight and can be induced to use these compounds as a source of energy and electrons for fixing nitrogen and producing hydrogen in the dark. This temporal separation allows an oxygen-producing process (photosynthesis) to coexist in the same cell with an oxygen-sensitive anaerobic process (nitrogen fixation). It may be possible to harvest hydrogen produced by this route but the current lack of knowledge about how microbes produce, store, and mobilize their carbon reserves limits the possibilities of exploiting this system for energy production. More research into these topics is needed. The strategies cyanobacteria use to protect nitrogenases from oxygen should also be more thoroughly investigated; no single strategy used by any organism is well understood, and the diversity of strategies has not been explored.
Hydrogen from Microbial Mats
Microbial mats that rely on the cycling of sulfur are known to develop in coastal areas. Studies indicate that these mats release hydrogen—possibly as an outlet for electrons when carbon dioxide is limiting.3 Alternatively, hydrogen release may be a way of coping with fluctuations in solar energy inputs. It may be possible to harvest hydrogen from microbial mats, but current lack of understanding of this system prevents progress. Further research is needed to determine whether the amounts of hydrogen produced by these mats are significant and whether a reductionist version of a microbial mat (with a limited number of well-understood species) can be productive.
METHANE
Methane is the end product of the anaerobic food chain—a sequence carried out by communities of fermentative bacteria, acid-producing intermediary organisms, and finally, methanogenic archaea. Methane-producing communities are very stable and resilient, but they are also complex and largely undefined. New species that engage in some aspect of methane production are frequently identified, but their functional and performance properties do not often differ substantially from that of known organisms.
Current commercial-scale methods of methane production yield from 50% to 100% conversion of substrate to methane on a mass basis, depending on the feedstock. Carbohydrate-rich substrates yield higher amounts of methane than feedstocks with high concentrations of lignocellulose or nitrogenous materials. The input feedstock also determines the composition of the gas product; carbohydrate substrates yield roughly 50% methane and 50% carbon dioxide, while more reduced (oxygen-poor) feedstocks yield higher proportions of methane.
Advantages
Methane has a number of advantages over other fuels. First and foremost, methane production is a relatively simple process that has been used for centuries to convert biomass into a useable energy product. As a result, methane production is very predictable, many of the factors that affect product yield are well known and easily controlled, and there is an existing infrastructure for using the fuel product.
Methane production is also very adaptable to small scale manufacturing situations, which could alleviate distribution requirements. For larger scale systems, existing transportation infrastructure could be used for distributing the product gas. Methane production can be carried out using many different input streams, carrying the majority of the “electron freight” into methane independent of the chemistry of the substrate. Methane is also relatively easy to harvest since it separates readily from the liquid phase. Infrastructure needs in methane production are low, and it is a productive way of disposing of biological wastes like manure.
Methane-producing communities are very stable and resilient, but they are also complex and largely undefined. Methane production is a relatively simple process that has been used for centuries to convert biomass into a useable energy product.
Disadvantages and Technical Difficulties
On the downside, methane production is a much-explored area of energy production, so there may not be much room to improve the yield and efficiency of this process. Current protocols are marked by their low productivity, although this could possibly be improved by using biofilm reactors or other newly engineered systems. Also, methane, like other gases, is relatively difficult to distribute as compared with liquid fuels.
A few technical difficulties continue to inhibit the successful use of methane as a biofuel. For example, biomass digestion for methane production cannot handle lignin, a component of plant cell walls. These materials must be made more accessible to the primary hydrolyzers in methane generation in order to make the process more efficient. Methane-producing microbial communities also operate only within a narrow pH range, so the acidity of methane-producing bioreactors must be carefully managed to avoid souring the digester.
Research Needs
There are numerous outstanding research needs with respect to developing methane as a biofuel, ranging from optimizing the productivity of the communities that carry out methanogenesis to exploring the possibility of converting methane into liquid biofuels. Methanogenesis is a community-based process, and there are two possible philosophies to optimizing this community: the reductionist approach and the ecological approach. It is unclear which philosophy would achieve the greatest success in improving methane yield.
In a reductionist approach to optimizing methanogenesis, researchers would generate a community de novo, that is, from scratch, compiling members from a limited number of known species. This could potentially create a community that is simpler and more tractable than the undefined consortia that are currently used to carry out methanogenesis. The “bottlenecks,” or rate-limiting steps, of methanogenesis would need to be identified in order to create an efficient community. For example, if syntrophy (the “trading” of substrates between species) is rate-limiting, then care needs to be taken to ensure that the species engaging in syntrophy are present and compatible. Sequencing the metagenome of a methanogenic community could help enable researchers to build better communities by providing new insights into the composition and functions of efficient natural communities.
An ecological approach is also possible in optimizing methano-genesis. The logic here is simple. Communities that carry out methanogenesis have evolved over millions of years, and in the interest of efficiency, it may be best to leave them intact. To make the most of these communities, it is necessary to understand the physical parameters that control methanogenesis. Research is needed to understand the basis of robustness in complex methanogenic communities. Currently, many of the networks and interactions in this process are not fully understood, but they may be crucial to methanogenesis.
New methods are needed for monitoring, stabilizing, and stimulating the catalytic activity of those microbes that are known to be critical to the productivity of methane-producing bioreactors.
Several issues with respect to the substrates for methano-genesis also need to be addressed. The rate-limiting factors in the utilization of acetate, a major substrate for methanogens, need to be determined in order to improve the growth rate of these organisms. Also, methanogenic communities have difficulties converting nitrogen-rich materials to methane because ammonia inhibits methanogens. The substrate ranges of methanogenic communities, and of methanogens in particular, need to be broadened in the interest of more complete biomass utilization.
Solubilization and degradation of lignocellulose are limiting factors in the conversion of this material to methane, and ways to accelerate these steps need to be explored. The rumens of ruminant mammals and the hindguts of termites are good model systems for efficient methane production from lignocellulose. However, it will be difficult to engineer improvements in these factors in undefined communities. In the absence of complete conversion of lignocellulose to methane, it may be possible to use the surplus, undigested material as feedstock for ethanol/alcohol production.
In the future, landfills could be constructed to optimize waste treatment and methane production, but it is unknown what changes could be expected in the microbial communities over time or what could limit their activity at different stages in development. Finally, the possibility of converting methane to liquid fuels needs to be explored. It may be possible to use methane oxidizing organisms, or their enzymes, to convert methane to methanol on a commercial scale, for example. In addition, new processes such as the in situ conversion of coal and hydrocarbons to methane, which may provide avenues for natural gas production while avoiding costs associated with removing the substrates from the ground, should be investigated. This technology should only be pursued if CO2 sequestration processes are functioning efficiently. Then engineering strategies to make substrates like coal more available and digestible may be encouraged. A deeper understanding of the global regulatory networks, metabolic pathways, and syntrophic relationships of methanogens and their communities is critical to these explorations.
Methanol
The possibility of using microorganisms to convert methane to methanol on a scale suitable for commercial use should be researched more fully. Methanol, a nonexplosive liquid, is relatively easy to transport, and can either be burned as a fuel or used in fuel cells. However, methanol has a lower energy density than gasoline and other alcohols, and a microorganism has yet to be found that can produce methanol at significant concentrations, as it is toxic to microbial cells. As a result, there are significant technical difficulties in using microorganisms to produce methanol for use as a fuel. Methanol may be produced from methane using microbial monooxygenase enzymes, but it is not known how best to stop the reactions so that methane is converted into methanol and not converted all the way to formaldehyde. It may be possible to combine metabolic pathways to achieve optimal methane conversion. Alternatively, it may be more feasible to engineer a catalyst to carry out this conversion. The possibility of using anaerobic methane oxidation to manufacture methanol has not been studied and merits further research.
Butanol, Longer Chain Alcohols, and Ketones
In addition to ethanol, hydrogen, and methane, many anaerobic microorganisms produce other high energy compounds, including butanol, long-chain alcohols, and ketones, that could either be used as fuels or as substrates for the manufacture of fuels. These substances are liquids, so they are potentially very easy to handle and transport. Butanol could be particularly useful; it has a higher octane rating than gasoline, making it a valuable fuel for any internal combustion engine made for burning gasoline. Because it is less hygroscopic, butanol can be shipped through the existing common-carrier pipelines, unlike ethanol.
The products of the fermentative production of butanol by solvent-producing species of the bacterium Clostridium are six parts butanol, three parts acetone, and one part ethanol. Unfortunately, the production of butanol by fermentation is self-limiting because of the toxicity of the products to cells. Technological advancements that have enabled the removal of products as they are generated, combined with increases in microbial tolerance of the products, have resulted in increases in productivity and yield.
The discovery or development of new solvent-producing microbes with greater tolerance for solvents is recommended. Engineering an organism with more or better membrane-associated solvent efflux pumps, which keep solvents out of the cells, could increase solvent yield. It would also be desirable to entice the fermenter to switch from making acids to making solvents earlier in the process. The mechanism for this switch is not known, but the concentration of undissociated acid plays a role, as does the pH and the proton motive force.
Solvent production in Clostridium is sporulation-related, but the ability to sporulate may drain cellular resources away from the work of solvent-making. Removing sporulation-related genes from solvent-producing species may improve production rates. Alternatively, it could be possible to introduce solvent-production genes (which are all on a plasmid and are therefore highly mobile) into another organism, like yeast, that is easily managed and does not sporulate.
A microbial fuel cell is a system that makes use of this ability by feeding the microorganisms a constant supply of biomass and bypassing most of their biosynthetic processes in order to continuously generate electricity.
Microbial Fuel Cells
A number of bacterial species are able to couple the oxidation of organic matter to the transfer of electrons to an electrode, thereby producing electricity. A microbial fuel cell is a system that makes use of this ability by feeding the microorganisms a constant supply of biomass and bypassing most of their biosynthetic processes in order to continuously generate electricity. Biomass substrates useful for fermentations can also be used in microbial fuel cells.
The arrangement of a microbial fuel cell is fairly simple: two chambers, one anaerobic and the other aerobic, are separated by a proton exchange membrane. Biomass fuel is fed to the bacteria on the anaerobic side. The bacteria then extract electrons from the biomass and deposit them on an anode that connects the two chambers, making a usable form of energy. The bacteria also extract protons from the biomass, allowing them to diffuse across the membrane to the cathode side. A catalyst on the aerobic cathode side combines the electrons, protons, and oxygen, making water. A primary goal of these systems is to minimize biosynthesis, which is a drain on the fuel cell reactants, in order to maximize electron flow.
The electrons that the bacteria extract from the substrate need a way to get to the electrode. They can either be shuttled to the electrode by chemical mediators like phenazine derivatives, or, if the strain is capable, directly from the cell through conductive appendages or through interconnected intercellular circuits.
The study of microbial fuel cells is in its infancy in comparison to other microbial energy technologies, and researchers still have a lot to learn about how to generate electrons. Efficiencies approaching 80% in shuttling electrons across the anode have been reported,4 but observations of greater than 95% efficiency are occurring.5 The most inefficient step of the process is the microbial interaction with the anode, on which the electrons from biomass are deposited. The rate of electron transfer to oxygen on the cathode side is also slow. The discovery of nanowires, electron conducting protein structures extending from the outer membrane of some bacterial cells, holds the promise for big improvements in anode performance.
Microbial fuel cells will likely be put to best use in situations in which energy needs are small but batteries are unsatisfactory. They may be used to power oceanographic instruments, for example, which are currently limited by battery power. Sediment fuel cells that exploit organic matter (or Fe(II), sulfide, or other electron source) and microbial communities in ocean sediments could potentially provide power for devices like these for many years.
A number of concepts hold promise for propelling microbial fuel cell technology forward. It may be possible to couple microbial redox systems with nanotechnology devices like nanoscale wires or nanotubes for electron transport or wires designed using biomimetics to imitate microbial pili, for example. Microbially produced electron shuttles, including phenazines, might also improve efficiency. Another possibility lies in combining phototrophs and chemoorganotrophs in multiple thin-layer photo-bioreactors. In such an arrangement, phototrophic microorganisms would make low molecular weight organic compounds to feed chemoorganotrophs continuously in the fuel cell.
Advantages
Microbial fuel cells have certain advantages over other microbial fuel technologies. They are reasonably efficient, and their ability to produce electricity continuously removes the constraint of seasonal variation in daylight intensity and photoperiod, factors that limit the productivity of hydrogen-generating photobioreactors. Microbial fuel cells are also adaptable to use in remote locations.
Disadvantages and Technical Difficulties
The disadvantages of microbial fuel cells is the slow rate that biomass is converted to electricity. Engineering advancements to increase the surface area of fuel cell electrodes is required because current scales directly with available surface area. Other practical difficulties include low current density, the scalability of the system, electrochemical resistances such as the rate of proton diffusion to the cathode, and increasing the rate of electron transfer between the microbes and the anode.
Research Needs
Microbial fuel cell research needs can be divided into microbio-logical issues and electrochemical issues. Crucial microbiological research topics include:
- Investigations into whether fuel cell efficiency can be improved through directed evolution of electricity-generating microorganisms. Bacteria have never before had any fitness incentive to generate electricity specifically, so applying selective pressure in the lab may well achieve success in improving fuel cell yield.
- Research into why some bacteria, such as Escherichia coli, can sustain continuous growth in a fuel cell while others, like Shewanella species, halt growth.
- Research into using other, new organisms as biocatalysts. The ability to transfer electrons to an anode is probably very broadly distributed in the bacterial world. Particular emphasis should focus on discovering:
- Microbes with improved performance characteristics like longer nanowires to conduct electrons and better electron shuttling properties in general,
- Thermophilic (heat-loving) and psychrophilic (cold-loving) organisms that can be used as biocatalysts, and
- Diverse microbial communities that form functional biofilms on the anode in the presence of substrate that increase the efficiency of electron delivery.
Needed electrochemical research includes:
- Research into specific redox pairs that are amenable to fuel cell applications,
- Investigations into the electrochemical mechanisms that drive fuel cells. Currently, neither the mechanism of conveying electrons to the electrode nor the mechanism of electron conductance along nanowires are completely understood.
- Research into the regulation of electron transfer network synthesis and how it may be controlled or engineered into other microbes.

(Energy Information Administration/Annual Energy Outlook 2006)
BIODIESEL FUELS
It may be possible to use lipid-accumulating microorganisms like yeast or algae to produce oil for making biodiesel (a fuel made from transesterified vegetable oil). One advantage of this type of fuel production is the ease with which oil can be harvested from the system. However, emissions from biodiesel-powered vehicles are worse, in terms of their impacts on human and environmental health, than emissions from petrodiesel-powered vehicles. Hence, emission control equipment must be reconfigured in order to use biodiesel in a conventional diesel vehicle.
The possibility that high lipid-content microbes could be used as a resource for biodiesel production should be more fully explored. It is possible that the lipid content of these organisms could be manipulated to enhance yield.
Microbe-Assisted Biomass Generation
Microorganisms can benefit the cultivation of biomass for energy conversion in a variety of ways; they can help manage plant pests and diseases, provide nutrients to plants, and maintain balances of carbon, nitrogen, phosphorus, and sulfur in the soil.
Fertilizer production and application constitutes 29% of all agricultural energy use, hence, fertilizing bioenergy crops could potentially be energetically costly. Plant species that associate with nitrogen-fixing bacteria have lower fertilizer needs, so employing them as bioenergy crops could cut down on the energy expended on their growth. Microbes associated with plant roots are also capable of stimulating uptake of essential nutrients that are in low abundance in soils.
Research Needs
A number of research topics in this area deserve consideration, including efforts to improve the yield of crops currently in use and the development of new bioenergy crops.
Attempts should be made to establish symbioses between nitrogen-fixing bacteria and bioenergy crops. Efforts should also be made to cultivate more species of nitrogen-fixing bacteria in order to have a more diverse “library” of strains from which to draw prospective symbionts.
More tools are needed for rapid monitoring and analysis of soil and rhizosphere (root zone) microbial communities.
Research is needed to investigate the efficiency of solar capture required for biomass generation.
The oceans could be a vast source of biomass for microbial energy production. For example, kelp is roughly 30% mannose, a product that can easily be fermented into methane and turned into energy. More research is needed to explore the possibilities the oceans offer for energy production.
Use of Microbes to Facilitate Processing of Other Energy Sources or to Minimize The Environmental Impact of Currently Used Sources
Microbes can potentially serve many functions in energy production. In addition to their direct roles as fuel producers, microorganisms could also be involved in the ecology of energy by lessening the environmental impacts of carbon-based energy generation or by converting the waste products of production into more useful or manageable commodities.
Carbon sequestration is one area in which microorganisms could make important contributions to sustainable energy production. Coccolithophores (a type of marine phytoplankton), for example, could be farmed in plots down gradient of coal or natural gas combustion to sequester carbon dioxide as calcium or magnesium carbonate. Microbial carbonic anhydrase could also be used to convert carbon dioxide to bicarbonate; however, more emphasis on elucidating basic microbial CO2 removal mechanisms by a variety of different organisms and enzyme systems is needed.
Microorganisms could be put to work in enhancing the efficiency of coal and petroleum operations by boosting oil recovery from the subsurface or by converting unrecoverable oil to natural gas in situ. If solubilized, coal could be a suitable substrate for anaerobic digestion, enabling the creation of useful energy products from this plentiful resource. These technologies must be accompanied by simultaneous development of efficient carbon sequestration procedures.
The residue from ethanol fermentation, which might otherwise go to waste, could be used as a substrate for many different microbial processes, including methane production. Microbial communities are inhibited by ethanol fermentation residue, so methanogenic cultures that are adapted to inhibitory compounds will need to be developed.
Research is also needed to uncover the limitations on methanogenesis in situ when extracting petroleum from the subsurface using microorganisms. Understanding the biochemistry of hydrocarbon degradation is critical to these extraction efforts. More work is needed in this area.
Lignin is a major component of waste from the production of biofuels, and new genes and microorganisms that can make use of this material to generate fuels and other useful products are needed. Termite guts may be a good model system for exploring novel routes of lignocellulose degradation. However, thermal conversion of lignin may be a more practical route than biodegradation, given lignin's relatively high heat of combustion.
OVERARCHING RESEARCH ISSUES
In addition to the specific research needs detailed in Section C, there are a number of general research themes that, if explored, can benefit the development of all modes of microbial energy production. These topics include bioprospecting, research on microbial communities, enzymology, the biology of non-growing cells, modeling, genomics, nanotechnology, techniques, and others.
BIOPROSPECTING
The scientific community needs a larger inventory of cultivated microorganisms from which to draw for microbial energy development. Bioprospecting can alleviate this shortfall. Also called “biodiversity prospecting,” bioprospecting seeks to catalog and capture the breadth of the world's biological diversity in order to put novel organisms and genes to work in energy production, industrial applications, medicine, or other areas.
Although metagenomics and new methods of identifying genes are important, and many of the functions desirable in energy production may be mined from metagenome samples (genomic DNA harvested from communities of microbes rather than single-species cultures), isolation of organisms with novel metabolisms and capabilities is still critical for the discovery of new and desirable metabolic functions. Hence, after carrying out metagenomic studies, the challenge becomes drawing out the microbes that encode the functions of interest from the environment. It is imperative to develop new methods for isolating microorganisms that have specific, desired functions.
The scientific community needs a larger inventory of cultivated microorganisms from which to draw for microbial energy development. Bioprospecting can alleviate this shortfall.
Careful design and implementation of multiple trait searching strategies that are guided by technical insights are recommended. This effort could be patterned after the successful efforts of the pharmaceutical industry that routinely carries out bioprospecting. The desired traits must be fully defined in an interdisciplinary effort to ensure that the isolates will meet energy conversion needs.
Biomining strategies that target microbes, microbial communities, and enzymes that can enhance the conversion of biomass to usable sugars should be implemented. The conversion of biomass to sugars is key to the creation of many end-product energy carriers, and lignocellulostic biomass is the most abundant resource of carbohydrates for these processes. However, despite the fact that this biomass is readily turned over in nature, few microbes that carry out the conversion of lignocellulose to sugars have been identified, suggesting that there is an untapped natural resource of microbes that can improve current technologies for the conversion of biomass into renewable fuels and chemicals. Metagenome sequencing of environments in which plant biomass is degraded at a high rate is recommended.
Advances are needed in our ability to cultivate heretofore unculturable microorganisms. It may be advisable to use a 96-well plate format for screening large numbers of organisms for desirable traits like electrical activity. In addition to finding new organisms, bioprospecting should also be used to isolate desirable traits that can then be engineered into “platform organisms,” those well studied that are amenable to manipulation.

(Energy Information Administration/Annual Energy Outlook 2006)
MICROBIAL COMMUNITIES
Many of the microbial processes critical to energy production are carried out by complex communities of organisms. There is a need to better understand the microbial community interactions that make these conversions possible. Better understanding of microbial community structure, robustness, networks, home-ostasis, and cell-to-cell signaling is also needed. A more thorough knowledge in all these areas will facilitate better modeling of microbial energy systems.
There is a need to study the fundamental mechanisms of energy distribution within microbial consortia, communities, and mats. One technique to do that is to apply high resolution techniques to determine the ATP contents of community members. Confocal microscopy, reporter genes, and adenylate charge ratio measurements would also be helpful in these studies.
Natural ecosystems containing complex microbial assemblages may contain organisms of potential interest in energy production. Studies of in situ metabolic activities and their regulation are warranted in order to optimize metabolic activities in vitro.
Tremendous advances have been made in imaging technologies that could allow a closer examination of biofilms and other complex communities to improve our control and use of these systems for energy transformation.
ENZYMOLOGY
A better understanding of the basic enzymology of microorganisms is necessary to move forward more quickly with microbial energy conversion. The actions of enzymes and enzyme complexes within the context of the whole cell, how they are regulated, where they are placed, what they interact with—all these topics need to be explored.
Nitrogenase is a good example of a poorly understood, but crucial, enzyme. There are still gaps in what is known about how the nitrogenase cofactor is assembled, why the enzyme needs ATP to function, which proteins are associated with the nitrogenase complex, and how the reductant gets carried to the enzyme. The enzymes of Photosystem II (PSII) also require further research. The exact placement of PSII in the cell membrane is critical to its function, and understanding how it is assembled and placed in the membrane could enable researchers to improve the system to direct the flow of light into energy-bearing products like hydrogen.
Research is needed to determine how best to apply metagenomic and directed evolution techniques to identify new, better enzymes for energy production and carbon sequestration. Annotation of putative gene products is distressingly poor. Improved approaches for determining the enzymatic function of predicted proteins would be most beneficial in the identification of alternative or improved metabolic pathways.
The bioinorganic chemistry of key catalytic enzymes should be studied in order to enable the design of bio-inspired synthetic catalysts. In addition, more knowledge of the enzymology of factors that limit potential efficient CO2 removal (i.e., via RubisCO and other primary catalysts) is needed to help mitigate the effects of CO2 production during cellulosic fermentations.
BIOLOGY OF NON-GROWING CELLS
One of the fundamental questions in microbial energy transformation is this: how can resting cells be induced to make energy products and not cell mass? In light of this challenge, there is a need to study and understand the behavior of these “metabolizing but non-growing” cells, including their maintenance energy requirements, the biology of starvation, the circumstances that bring about slow growth, and how to maintain non-growing cells at high densities. It is desirable to be able to turn growth-decoupled systems “on” and “off” rapidly.
MODELING AND MODEL ORGANISMS
Better modeling tools are needed to facilitate progress in microbial energy conversion. Models of metabolic dynamics, including concentrations of reductants and regulation of electron flow need to be improved. Experts in mechanistic and functional models should be included in model development in order to ensure that the metrics for each particular system are properly defined. Global techno-economic models of microbial energy transformation systems, which seek simultaneously to describe the resource flows into and out of a system as well as its economics, are needed and should be made publicly available on the internet.
Modeling methods that are user friendly for microbiologists and models that can enhance the dialog between chemical engineers and microbiologists are also needed.
It is important to continue the study of model organisms, as an understanding of model systems will advance not only the fundamental knowledge of microorganisms, but also the accuracy of genome and metagenome sequence annotation. It may be advisable to assemble a small collection of microorganisms that could serve as models for use in development of improved strains for energy conversion.
Broad investment in a range of strategies for microbial fuel production is recommended. It is in the interest of all parties to develop a diverse energy portfolio.
GENOMICS
The tools of genomics, proteomics, and metabolomics should be applied to determine how microbial cells carry out the functions of interest in microbial energy conversion, what limits these functions now, and what is likely to limit them in the future. It is also important to determine how these global processes are integrated with, and may be predicted from, genome sequences.
It is important to continue sequencing the complete genomes of microbes used in energy transformation, particularly since whole genome sequencing is now reasonably priced and the potential payoffs of sequencing are high.
The idea of using synthetic genomics to create entirely new organisms to carry out the desirable functions in microbial energy conversion has tremendous potential.
The metagenome sequences of targeted communities, including those found in the termite hindgut, rumen, microbial mats, and shipworm guts, could be highly useful in understanding and potentially manipulating biomass degradation for energy conversion.
NANOTECHNOLOGY
Nanotechnology could be a useful approach in designing bioreactors to improve product tolerance and in optically interrogating bioreactor membranes to evaluate stress. Micro- and nano-fluidics will eventually be useful for DNA fingerprinting linked with temporal and spatial analysis to examine system dynamics.
Nanotechnology tools, such as micromanipulation and fluidics, are likely to be useful in moving, capturing, and analyzing microbial cells in microbial energy systems. These capabilities would be useful in separating microbes with a DNA tag from the rest of the community, for example.
TECHNIQUES
The success of microbial energy conversion is largely dependent on the development of new and improved techniques for examining and optimizing microbial systems.
New and better methods are needed for measuring physiological processes on the microscale. Measuring the activities of single cells remains a technical challenge for microbiology. Singe cell measurements could enable insights into the stochasticity of microbial energy systems and the activity of unculturable microorganisms.
Improved ways of visualizing electron flow in real time are needed.
A better rubric for examining energy conversion efficiency is necessary to meaningfully compare different systems of microbial energy transformation and to evaluate their comparative advantages. For example, a system that currently operates at 0.1% conversion efficiency has more room for improvement and could merit more resource investment than a process that operates at 50% efficiency, but there is no widely accepted standard method for calculating efficiency in order to make such a comparison. The field also requires standardized methods for quantitatively evaluating the energy output of these systems.
Research in microbial energy conversion should make better use of the techniques of high-throughput technology in order to identify new microbes, enzymes, and products that can be put to use in energy transformation. High-throughput tools would also be useful for carrying out directed evolution of known enzymes in order to improve their catalytic efficiencies.
Rapid functional screens are needed to identify desirable microbial traits that can be used in energy conversion.
ADDITIONAL RESEARCH RECOMMENDATIONS
Other recommendations for moving forward with research in microbial energy conversions include:
- The development of pilot plants for microbial energy fuel production. Pilot plants are necessary but largely unavailable for small companies and universities to use for testing of their particular processes. The canyon between fundamental research and commercialization is massive and bridges are needed.
- Study the fundamentals of electron flow in microbes used in energy transformations. Electron flow is important for any organism that would produce electricity or a reduced chemical product. We also need an improved understanding of how different metabolic modules interact with each other for the production of energy fuels.
- Examine the communities of hydrogen-producing microbial mats. Microbial mats produce a tremendous amount of hydrogen, and we need an understanding of these communities and why they allow hydrogen to escape.
- Study microbial strategies that impact energy conversions but are not directly involved in the process. For example, we need a better understanding of the systems bacteria use to protect oxygen sensitive nitrogenases, hydrogenases, and methyl transferases.
- Apply systems biology in metabolic flux analysis to identify bottlenecks in energy-relevant microbial processes.
- Develop genetic exchange systems for new microbes to facilitate the construction of second-generation biocatalysts for the production of renewable chemicals and fuels.
CURRENT FUNDING MODELS AND MICROBIAL ENERGY RESEARCH
Recently, there has been a shift away from investigator-initiated grants for microbial energy research. This change, combined with a new emphasis on top-down grant program management and peer evaluation systems that reward “safe science,” has reduced innovation and subverted the funding of basic research in favor of applied programs. Although a large unified effort, like that used in the Manhattan Project, which emphasized sharing results and coordinating research approaches to develop nuclear technologies in the 1940s, could help advance microbial energy conversion, a combination of individual investigator-initiated research and multi-investigator interdisciplinary research is a more balanced approach. The emphasis of the current funding paradigm should be shifted toward investigator-initiated projects while maintaining some investment in interdisciplinary research anchored by concrete goals.
Research and development in microbial energy conversion call on the skills of individuals from multiple disciplines, not just microbiology. Educators must emphasize multidisciplinary training at both the undergraduate and graduate levels in order to meet the demands of this field of research.
Broad investment in a range of strategies for microbial fuel production is recommended. In the short term, emphasis can be placed on improving mature technologies, but this investment should not come at the expense of investing in basic research to bolster other, less-proven forms of microbial energy conversion. It is in the interest of all parties to develop a diverse energy portfolio.
Discontinuous funding strategies, which can change a researcher's funding from year to year, make planning research in microbial energy technologies problematic.
Governmental support is essential for funding small scale demonstration-stage energy systems that can eventually lead to full-size demonstrations. Standard research grants are available from several agencies for funding of fundamental research in microbial energy conversion, and proven technologies can attract funding from industry. However, funding for demonstration-stage projects in between these two stages is scarce.
EDUCATION, TRAINING, INTERDISCIPLINARY COOPERATION, AND COMMUNICATION
More emphasis needs to be placed on multidisciplinary education and training and on cooperation between disciplines in order to make the most of microbial fuel production technologies and to meet the research needs of the future.
EDUCATION AND TRAINING NEEDS
Research and development in microbial energy conversion call on the skills of individuals from multiple disciplines, not just microbiology. Educators must emphasize multidisciplinary training at both the undergraduate and graduate levels in order to meet the demands of this field of research. Some specific suggestions for improving the training of students destined for careers in microbial fuel production include:
- Requiring graduate students to complete rotations in different disciplines,
- Requiring internships or international study,
- Funding post-doctoral researchers who engage in multidisciplinary research,
- Funding sabbaticals for research professionals to study in a related field, and
- Developing university institutes in which researchers and students from many fields can have a common forum for discussing and sharing research in microbial energy conversion.
Educators must emphasize multidisciplinary training at both the undergraduate and graduate levels in order to meet the demands of this field of research.
Recent advances in genetic sequencing have made genomic data easy to generate, but analyzing those data remains a challenge. There is a need to train microbial physiologists who can interpret genomic data for use in the study of microbial energy conversion and elsewhere. University-level training in microbial physiology needs to be revamped and reenergized to meet this need. These training programs should include material that can facilitate collaborative efforts between physiologists and professionals in other disciplines (engineering, for example) to help solve the problems of energy transformation.
INTERDISCIPLINARY COOPERATION AND MULTIDISCIPLINARY RESEARCH
The study of microbial fuel production is multidisciplinary by nature: isolating and optimizing the microorganisms, designing and assembling the bioreactor or fuel cell, processing the biomass fuel, separating the products—these activities call on the skills of many different experts. Hence, strategies are needed to promote and advance cooperation between microbiologists, engineers, modelers, bioinformaticists, inorganic chemists, materials scientists, plant molecular biologists, plant breeders, and other experts involved in microbial energy research. Particular emphasis should be placed on assembling collaborations to make best use of genomics information and to develop innovative algorithms to apply to the specific needs of microbial energy conversion.
Unfortunately, mechanisms for funding joint research proposals in microbial energy are few and far between. It is important to create additional grant initiatives to fund these types of projects. Also, funding agencies often take a conservative approach in backing research by favoring projects that can muster a great deal of preliminary data. Relaxing the burden of proof required to secure funding would work to the advantage of fields like microbial energy conversion, which operate at the boundaries of the traditional departmental disciplines. Interdisciplinary training grants would also be helpful.
In carrying out multidisciplinary research, it is beneficial to assemble as many members of the research group as possible in close proximity, preferably within the same building. The development of core facilities is one mechanism for bringing scientists with diverse expertise together. One example of a successful interdisciplinary collaboration is the University of Southern California (USC) Interdisciplinary Microbial Fuel Cell group, which includes scientists from the USC departments of Earth Sciences (microbiology), Biology (molecular and evolutionary biology), Chemistry (membrane chemistry), and Engineering (modeling and electrochemistry) and houses them all in the same building. The group also has collaborations with scientists from other universities, including faculty from Penn State, Pacific Northwest National Labs, and the Korean Institute of Science and Technology.
THE ROLE OF PROFESSIONAL SOCIETIES IN MICROBIAL ENERGY RESEARCH
Professional societies should take the lead role in communicating research findings and opportunities in microbial fuel production in an accessible way to the public, legislators, and policy makers.
Professional societies must also take responsibility for educating their members about funding opportunities in microbial energy conversion available from private organizations.
RECOMMENDATIONS
- Cellulose and lignocellulose are the preferred substrates for producing liquid transportation fuels, of which ethanol is the most commonly considered example. Generating fuels from these materials is still difficult and costly. A number of challenges need to be met in order to make the conversion of cellulose and lignocellulose to transportation fuels more cost-competitive, including:
- Optimizing different biomass pretreatment processes for different substrates,
- Engineering more efficient enzymes for lignocellulose and hemicellulose degradation,
- Developing more efficient fermenting organisms, and
- Reducing the cost of producing cellulose- and lignocellu-lose-degrading enzymes.
- The design of hydrogen-producing bioreactors must be improved in order to more effectively manage hydrogen removal, oxygen exclusion, and, in the case of photobioreactors, to capture light energy more efficiently.
- Methane production may be optimized by fine-tuning methanogenic microbial communities. In a reductionist approach to optimizing methanogenesis, researchers would generate a community de novo, compiling members from a limited number of known species. This could potentially create a community that is simpler and more tractable than the undefined consortia that are currently used to carry out methanogenesis. In an ecological approach, the network of physical parameters that control methanogenesis in a natural microbial community could be adjusted and optimized.
- The ability to transfer electrons to an anode in a microbial fuel cell is probably very broadly distributed in the bacterial world. Research in microbial fuel cells should focus on discovering:
- microbes with characteristics hypothesized to improve performance like longer nanowires to conduct electrons and better electron shuttling properties in general,
- thermophilic (heat-loving) and psychrophilic (cold-loving) organisms that can be used as biocatalysts under alternative environmental conditions, and
- Diverse microbial communities that form biofilms on the anode in the presence of substrate.
The scientific community needs a larger inventory of cultivated microorganisms from which to draw for energy conversion development. New and unusual organisms for manufacturing fuels and for use in fuel cells can be discovered using bioprospecting techniques. Particular emphasis should be placed on finding microbes, microbial communities, and enzymes that can enhance the conversion of lignocellulosic biomass to usable sugars.
Many of the microbial processes critical to energy conversion are carried out by complex communities of organisms, and there is a need to better understand the community interactions that make these transformations possible. Better understanding of microbial community structure, robustness, networks, homeostasis, and cell-to-cell signaling is also needed.
A better understanding of the basic enzymology of microorganisms is needed in order to move forward more quickly with microbial energy production. Research should focus on the actions of enzymes and enzyme complexes within the context of the whole cell, how they're regulated, where they're placed, and what they interact with.
Better modeling tools are needed to facilitate progress in microbial energy transformations. Models of metabolic dynamics, including levels of reductants and regulation of electron flow need to be improved. Global techno-economic models of microbial energy conversion systems, which seek to simultaneously describe the resource flows into and out of a system as well as its economics, are needed and should be made publicly available on the internet.
More emphasis needs to be placed on multidisciplinary education and training and on cooperation between disciplines in order to make the most of microbial energy conversion technologies and to meet the research needs of the future.
Footnotes
- 1
Holtzapple, MT, RR Davison, MK Ross, S Albrett-Lee, M Nagwani, CM Lee, C Lee, S Adelson, W Kaar, D Gaskin, H Shirage, NS Chang, VS Chang, and ME Loescher. 1999. Biomass conversions to mixed alcohol fuels using the MixAlco process. Appl. Biochem. Biotechnol. Spring 77-79:609-31.
- 2
Gosse, M. C. Flickinger. 2006. “Investigation of Latex Micro-photobioreactor for Hydrogen Production using Non-Growing Rhodopseudomonas palustris.” 28th Symposium on Biotechnology for Fuels and Chemicals, Nashville, TN
- 3
Hoehler, TM, BM Bebout and DJ Des Marai. 2001. The role of microbial mats in the production of reduced gases on the early Earth Nature 412, 324-327.
- 4
Rabaey, K, N Boon, SD Siciliano, M Verhaege and W Verstraete. 2004. Biofuel cells select for microbial consortia that sef-mediate electron transfer. Appl. Environ. Microbiol. 70:5373-5382.
- 5
Lovley, D, personal communication.
- NLM CatalogRelated NLM Catalog Entries
- Review Upflow anaerobic sludge blanket reactor--a review.[Indian J Environ Health. 2001]Review Upflow anaerobic sludge blanket reactor--a review.Bal AS, Dhagat NN. Indian J Environ Health. 2001 Apr; 43(2):1-82.
- Solar fuels via artificial photosynthesis.[Acc Chem Res. 2009]Solar fuels via artificial photosynthesis.Gust D, Moore TA, Moore AL. Acc Chem Res. 2009 Dec 21; 42(12):1890-8.
- Combustion in the future: The importance of chemistry.[Proc Combust Inst. 2020]Combustion in the future: The importance of chemistry.Kohse-Höinghaus K. Proc Combust Inst. 2020 Sep 25; . Epub 2020 Sep 25.
- Recent advances in sensitized mesoscopic solar cells.[Acc Chem Res. 2009]Recent advances in sensitized mesoscopic solar cells.Grätzel M. Acc Chem Res. 2009 Nov 17; 42(11):1788-98.
- Review The Uncharted Microbial World: Microbes and Their Activities in the Environment: This report is based on a colloquium, sponsored by the American Academy of Microbiology, convened February 9-11, 2007, in Seattle, Washington[ 2008]Review The Uncharted Microbial World: Microbes and Their Activities in the Environment: This report is based on a colloquium, sponsored by the American Academy of Microbiology, convened February 9-11, 2007, in Seattle, Washington. 2008
- Microbial Energy ConversionMicrobial Energy Conversion
- Specific Protein Links for Conserved Domains (Select 213019) (614)Protein
Your browsing activity is empty.
Activity recording is turned off.
See more...