This book is distributed under the terms of the Creative Commons Attribution-NonCommercial-NoDerivatives 4.0 International (CC BY-NC-ND 4.0) ( http://creativecommons.org/licenses/by-nc-nd/4.0/ ), which permits others to distribute the work, provided that the article is not altered or used commercially. You are not required to obtain permission to distribute this article, provided that you credit the author and journal.
NCBI Bookshelf. A service of the National Library of Medicine, National Institutes of Health.
StatPearls [Internet]. Treasure Island (FL): StatPearls Publishing; 2024 Jan-.
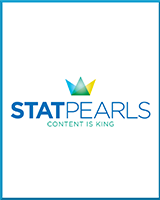
StatPearls [Internet].
Show detailsDefinition/Introduction
hThe basic understanding of radiation physics is important when dealing with radiation and explaining its associated health effects. Atoms are the fundamental building blocks of matter and are composed of protons, neutrons, and electrons. A chemical element holds the same number of protons, which corresponds to its atomic number. However, depending on the number of neutrons in the nucleus, the atomic mass of the element can change. This difference is what creates an isotope of an element. Electrons orbit the nucleus and are arranged in layers called shells. Removing one of the outer electrons from an atom emits energy, usually in the form of gamma rays. This phenomenon can is called ionizing radiation.[1] An important principle in ionizing radiation is the stability of isotopes and nuclides. When an isotope of an element has too few or too many neutrons in the nucleus, it becomes unstable. Nuclei are most stable when protons and neutrons add up to certain numbers, including 2, 8, 20, 82, and 126. Physics shows that odd numbers of neutrons and protons are less stable than even numbers. In a radioactive decay process, these radioactive and unstable nuclides can transform into a more stable nuclide that ultimately emits beta particles, alpha particles, or photons that include X-rays and gamma rays. The particles emitted are a form of radiation.[2]
Radioactive isotopes have unique characteristics determined by the type of particle, how much energy it emits, and the rate of decay. The different types of particles include alpha, beta, and photons. It is important to appreciate the different properties of each particle to understand the effect it has on the atoms with which it interacts. Depending on the mass, velocity, charge, and electron density of the target material, the extent of kinetic energy lost and the subsequent transfer of energy to certain mediums vary.[3]
Alpha particles consist of two protons and two neutrons. The emission of this particle results in a reduction of its atomic mass by four and a reduction of its atomic number by two. The emission of alpha particles usually results from the radioactive decay of heavy elements such as plutonium, uranium, and radium. Alpha particles have a positive charge of +2 and have a large ionizing power. However, due to their charge and large density, they typically lose energy rapidly over a short period and distance. The average distance that an alpha particle travels is about 3 to 5 cm, and it usually cannot penetrate clothes or skin. As a result of these characteristics, to cause any effect or damage, alpha particles must be emitted close to their target.[4][1][5]
Beta particles subdivide into negative and positive emissions. A negative beta emission has a single negative charge, and it increases the number of protons by one and decreases the number of neutrons by one. A positive beta emission contains a single positive charge, and it decreases the number of protons by one and increases the number of neutrons by one. In both cases, the atomic mass stays the same, but it forms a different element. Unlike alpha particles, beta particles can travel far and penetrate through water and tissue with high energy.[4][5]
When radioactive decay occurs in an element, residual energy produced from the transformation is typically stored within its nucleus and, therefore, ultimately excites the nucleus. Because high energy states cause instability, the nuclei release this energy to return to their baseline energy level. The energy that is released is typically in the form of gamma rays.[1] Gamma rays can also travel very far and can deposit or travel through substances such as iron, tissue, and even concrete. X-ray production occurs in a similar pattern, but instead of creating energy in the nucleus, energy generation occurs in the surrounding electrons. This process is called an internal conversion. When a nucleus is in an excited state, it ejects a gamma-ray. This gamma-ray interacts with one of the orbital electrons, typically in the innermost K layer. The gamma-ray is completely absorbed, and the atom is left with a void in the layer where the electron originally was. The vacant spot then becomes filled by an outer shell electron resulting in the production of an X-ray. X-rays can also be generated with the acceleration of an electron. This mechanism is demonstrated in the production of radiographic images utilizing X-rays.[4][5][6]
Radiation can be toxic when exposed in large amounts, and it is thus important to measure. The process of radioactive decay is random, and it is, therefore, difficult to predict when a particular atom will start to decay. However, it is possible to quantify the number of isotopes in a group that decays over a period of time. This rate of decay is called radioactivity, and it is proportional to the number of atoms of a certain radioactive material over its half-life. The half-life of an isotope is the time it takes for 50% of the nuclide in a group to decay. This rate is measured in Becquerels (Bq) in international units or Curies (Ci), which is used in the United States. By emitting particles, the radioactivity of an element can generate radiation. Radiation is clinically measured by the absorbed dose, which is equal to the amount of energy deposited in a specific region. It is calculated as the mean energy absorbed divided by the mass of the target material. In the practice of radiation oncology, the absorbed dose is measured as "gray" (Gy), with doses expressed in fractions over a time period.[7][1]
Issues of Concern
Radiation can be exposed internally or externally. Radiation exposure can occur internally when radionuclides enter the body through ingestion, inhalation, or the skin. When the nuclides are deposited into the skin, they undergo radioactive decay and emit radiation. External radiation exposure occurs via sources in the surrounding environment, such as nearby water, ground surfaces, air, or even x-ray tubes. In external radiation, radiation enters the body and penetrates the organs. Another example of this includes terrestrial and cosmic radiation, such as radiation from the sun. The sun continuously emits particles through space, eventually reaching the earth's surface and exposing humans to radiation. These natural exposures commonly occur in our daily life and are typically not destructive due to the body's ability to efficiently repair DNA damage. However, it is important to note that some tissues and organs are more sensitive to radiation than others. Rapidly dividing cells such as ones in the intestinal mucosa and bone marrow are the most vulnerable to radiation.[8]
Other issues of concern relate to geographical locations, house and work environments, and length of exposure. Mining for natural uranium is one example of the harmful effects of radiation. Uranium is known to have a very long half-life and emits powerful alpha particles when it decays. Uranium continues to decay, forming different elements throughout the process until it reaches a stable element of lead. During this decay process, unstable radioactive gas is produced, which can become suspended in the air; this causes a potential problem for miners due to the possibility of inspiring these particles. Alpha emissions contain a large amount of energy but can only travel short distances. When inhaled by miners, these particles can deposit into the lung tissue and cause deleterious effects directly to the alveoli cells. The same concept applies to any radiation that strikes human tissue.[3][4][8][5]
Cancer development is always an issue of concern when it comes to radiation exposure. In both internal and external radiation, ionizing radiation can damage DNA indirectly or directly. Direct damage typically occurs with high ionizing particles such as alpha or beta particles. These particles can interact and transfer their energy directly to the cell's DNA, causing single-stranded or double-stranded breaks or chromosomal damage. Radiation can also cause damage indirectly by the formation of free radicals produced through ionization of surrounding water. This mechanism is present in about two-thirds of all radiation-induced cell damage. It is always important to note the increased risk of cancer development due to DNA mutations that occur with ionizing radiation.[3][9]
Clinical Significance
The principles of radiation physics apply to the field of radiation oncology, and ionizing radiation can be useful in the treatment of many different types of diseases. Radiation therapy uses targeted, high-dose radiation to indirectly or directly kill or stop the growth of cancer cells. The same mechanism of DNA damage is apparent in radiation therapy. Because cancer cells have a high mitotic rate, radiation can be very effective in cancer treatment. To put this into perspective, 1-2 Gy of radiation typically results in 40 double-stranded breaks in DNA.[3] External beam radiation is a form of radiation therapy that uses ionizing radiation to target tumors from sources outside of the body. Similar to the production of radiographic X-ray imaging, an electron is accelerated using a linear accelerator machine, and this results in the generation of photons. This external beam passes through the body, and energy is deposited throughout the path of the beam, targeting the primary tumor. Due to the damaging effects of radiation and the proximity of the tumor to surrounding healthy organs, these beams can be manipulated to minimize their damaging effects.[7][4]
In 3D conformal radiation therapy, radiation can be given from many different directions so that the beam can converge on the target tumor, and it can better accommodate the size and shape of the tumor. With Intensity-modulated radiation therapy or IMRT, the dosage of the beams can be manipulated to decrease the dose to surrounding healthy tissue and increase the dose to the target tumor. Tumors are carefully mapped out using CT or MRI imaging, and the appropriate dose for the tumor, as well as the surrounding tissues, is proposed during planning.[1] These methods ultimately minimize the effect of radiation on the normal cells surrounding the tumor. Duration is another factor that can be manipulated depending on the type of disease and target organ. Radiation therapy can be fractionated so that a small dose of radiation can be given throughout a period of weeks, a single session, or a couple of days. For example, Stereotactic radiosurgery is a procedure usually done in one to five sessions, depending on the organ treated. It has utility in many conditions such as arteriovenous malformations, trigeminal neuralgia, acoustic neuromas, and cancers of the brain, liver, prostate, lung, breast, and spine.[7][1]
Cancer is also treatable using proton beam radiation, where protons are used instead of photons and electrons. Hydrogen atoms first split into positively charged protons and negatively charged electrons. The protons are then shot through a vacuum tube into a linear accelerator, where their energy further accumulates as they accelerate along with the delivery system. Once it reaches the desired energy level of about 100 million to 200 million electron volts, these protons can penetrate any depth within the body. These proton beams can be manipulated in shape and direction via magnets. To further customize and increase the precision of delivery, the opening of the proton accelerator can be changed to shape the beam as it exits the machine. When using photons or X-rays in radiation therapy, energy is deposited throughout the path of the beam. As a result, it affects tissue before and after it hits the tumor. Proton beam therapy is beneficial in that physicians can control where the beam deposits most of its energy. Protons travel through the body, releasing some energy throughout the path, but once they reach a certain point, called the Bragg peak, they deposit most of their energy. As a result, this type of radiation therapy focuses more energy on the actual tumor and spares the surrounding tissue.[1]
Brachytherapy is another radiation therapy where a clinician implants radioactive material temporarily or permanently near or inside the target object. It is a type of internal radiation and is used to deliver higher doses of radiation in a more specific and smaller area that cannot be possible with external beam radiation. Brachytherapy categorization depends on the applicator type, anatomical site, source type, the type of implant, or the dose rate. Interstitial implants are radioactive sources either incased in seeds or wires, and they are inserted in the tumor. These are useful for cancers such as prostate cancer. Implants using molds or plaques are typically used for superficial lesions where radioactive sources are directly placed over the skin. Radioactive sources placed inside a body cavity such as the vagina or cervix are called intracavitary implants. High dose rate implants are given at dose rates greater than 20 cGy/min and are typically given only for a few minutes.[10] Low dose rate implants remain inside the target organ or tumor for a few days or permanently left inside. The inverse square law is the fundamental principle behind brachytherapy. This law states that the energy received at a given distance from the source is inversely proportional to the square of the distance from the source, denoted by 1/r^2. In other words, the energy intensity increases as the distance from the source to the target lessen and vice versa. If the source is three times as far, it is one-ninth the intensity or exposure. Brachytherapy uses this law to allow the delivery of higher doses to adjacent areas.[11][12]
The precise delivery of radiation and its effectiveness in treating tumors has revolutionized the field of cancer treatment, and it has become part of the mainstay treatment for a multitude of cancers.
Nursing, Allied Health, and Interprofessional Team Interventions
The use of radiation should be performed with caution as it can cause detrimental effects when used for an extended period. The International Committee on Radiological Protection or ICRP has proposed three recommendations before usage of ionizing radiation. The principle of justification states that the use of radiation should always do more good than harm. The principle of optimization of protection highlights the importance of using the lowest possible dose of radiation for any case. The principle of application of dose limits indicates that the dose given should never exceed the recommended dose for an individual. The environmental protection agency or EPA and the CDC have also recommended ways to protect against excessive exposure to radiation through the principle of ALARA (As Low As Reasonably Achievable). The ALARA principle proposes three concepts that can be useful to best protect against radiation exposure. It recommends minimizing the amount of time exposed to radiation, increasing the distance from the radiation source, and shielding against radiation any time you are at risk of exposure. It emphasizes the importance of barriers such as standing behind walls during appropriate times and using personal protective equipment around radioactive sources.[13] These principles merit attention for all healthcare professionals dealing with radiation to help protect themselves and their patients. The usage of radiation should take place in a structured environment, and productive communication is a necessity. Healthcare workers dealing with radiation should talk with their radiation safety professionals and safety officers to discuss the use of personal protective equipment and the proper instrumentation to protect themselves from radiation best.[3] [Level I]
When planning radiation therapy for a patient, a great deal of communication and teamwork is part of the treatment plan. Due to the danger of using high-energy radiation beams for treatment, careful planning needs to occur to minimize radiation exposure to healthy areas in the body. A radiation oncologist works with dosimetrists and medical physicists to calculate and propose the best dose and treatment plan for the patient. It can take several days to create a treatment regimen, and the result is a personalized treatment for that specific disease or tumor. Dosimetrists contribution to the treatment process involves generating the dose calculations and dose distribution of the radiation beam. Once the radiation oncologist prescribes a dose to the defined tumor volume, the dosimetrists will design and determine the treatment field, technique, and patient set up to accurately deliver the prescribed dose while avoiding vital structures.[14] Medical physicists also contribute to radiation therapy treatment by applying their knowledge in physics into the practice of medicine. One of their primary duties is to accurately calculate the radiation output during treatments using external radiation beams or internal radiation sources. Medical physicists use extensive calculations to determine the best dosage and projection of radiation while assessing other physiologic variables such as blood flow and metabolic rates. While they play a vital role in diagnosing and treating patients, they also aid in quality assurance, radiation safety, and technical consultancy of their medical equipment and facility. For the treatment team to efficiently and accurately diagnose and treat patients, all equipment must undergo extensive testing. It is also essential to address the safety, handling, storage, and disposal of radioactive substances.[7][4][15]
References
- 1.
- Mehta SR, Suhag V, Semwal M, Sharma N. Radiotherapy: Basic Concepts and Recent Advances. Med J Armed Forces India. 2010 Apr;66(2):158-62. [PMC free article: PMC4920949] [PubMed: 27375326]
- 2.
- Lenaerts E, Coucke P. [Radiation physics for beginners]. Rev Med Liege. 2014;69 Suppl 1:13-5. [PubMed: 24822299]
- 3.
- Matthews EP. Radiation Physics, Biology, and Protection. Radiol Technol. 2019 May;90(5):471-485. [PubMed: 31088948]
- 4.
- Bortfeld T, Jeraj R. The physical basis and future of radiation therapy. Br J Radiol. 2011 Jun;84(1002):485-98. [PMC free article: PMC3473639] [PubMed: 21606068]
- 5.
- Donya M, Radford M, ElGuindy A, Firmin D, Yacoub MH. Radiation in medicine: Origins, risks and aspirations. Glob Cardiol Sci Pract. 2014;2014(4):437-48. [PMC free article: PMC4355517] [PubMed: 25780797]
- 6.
- Nakashima J, Duong H. StatPearls [Internet]. StatPearls Publishing; Treasure Island (FL): Jul 31, 2023. Radiology, Image Production and Evaluation. [PubMed: 31985938]
- 7.
- Tonnessen BH, Pounds L. Radiation physics. J Vasc Surg. 2011 Jan;53(1 Suppl):6S-8S. [PubMed: 20869192]
- 8.
- Willers H, Held KD. Introduction to clinical radiation biology. Hematol Oncol Clin North Am. 2006 Feb;20(1):1-24. [PubMed: 16580554]
- 9.
- Bolus NE. Basic review of radiation biology and terminology. J Nucl Med Technol. 2001 Jun;29(2):67-73; test 76-7. [PubMed: 11376098]
- 10.
- Mendez LC, Morton GC. High dose-rate brachytherapy in the treatment of prostate cancer. Transl Androl Urol. 2018 Jun;7(3):357-370. [PMC free article: PMC6043748] [PubMed: 30050796]
- 11.
- Chargari C, Deutsch E, Blanchard P, Gouy S, Martelli H, Guérin F, Dumas I, Bossi A, Morice P, Viswanathan AN, Haie-Meder C. Brachytherapy: An overview for clinicians. CA Cancer J Clin. 2019 Sep;69(5):386-401. [PubMed: 31361333]
- 12.
- Douk HS, Aghamiri MR, Ghorbani M, Farhood B, Bakhshandeh M, Hemmati HR. Accuracy evaluation of distance inverse square law in determining virtual electron source location in Siemens Primus linac. Rep Pract Oncol Radiother. 2018 Mar-Apr;23(2):105-113. [PMC free article: PMC5908270] [PubMed: 29681773]
- 13.
- Strauss KJ, Kaste SC. The ALARA (as low as reasonably achievable) concept in pediatric interventional and fluoroscopic imaging: striving to keep radiation doses as low as possible during fluoroscopy of pediatric patients--a white paper executive summary. Pediatr Radiol. 2006 Sep;36 Suppl 2(Suppl 2):110-2. [PMC free article: PMC2663649] [PubMed: 16862422]
- 14.
- Adams RD. The future of medical dosimetry. Med Dosim. 2015 Summer;40(2):159-165. [PubMed: 25861838]
- 15.
- Wang B, White G. The role of clinical medical physicists in the future: Quality, safety, technology implementation, and enhanced direct patient care. J Appl Clin Med Phys. 2019 Jun;20(6):4-6. [PMC free article: PMC6560227] [PubMed: 31099457]
Disclosure: Justyn Nakashima declares no relevant financial relationships with ineligible companies.
Disclosure: Hieu Duong declares no relevant financial relationships with ineligible companies.
- Evidence for a new nuclear 'magic number' from the level structure of 54Ca.[Nature. 2013]Evidence for a new nuclear 'magic number' from the level structure of 54Ca.Steppenbeck D, Takeuchi S, Aoi N, Doornenbal P, Matsushita M, Wang H, Baba H, Fukuda N, Go S, Honma M, et al. Nature. 2013 Oct 10; 502(7470):207-10.
- Review Physics and basic parameters of brachytherapy.[J Surg Oncol. 1997]Review Physics and basic parameters of brachytherapy.Lee EJ, Weinhous MS. J Surg Oncol. 1997 Jun; 65(2):143-50.
- Photonuclear dose calculations for high-energy photon beams from Siemens and Varian linacs.[Med Phys. 2003]Photonuclear dose calculations for high-energy photon beams from Siemens and Varian linacs.Chibani O, Ma CM. Med Phys. 2003 Aug; 30(8):1990-2000.
- [Wilhelm Conrad Röntgen and the discovery of X-rays].[Bull Acad Natl Med. 1996][Wilhelm Conrad Röntgen and the discovery of X-rays].Tubiana M. Bull Acad Natl Med. 1996 Jan; 180(1):97-108.
- Review Short Refresher of Radiobiology.[Nuclear and Radiological Emerg...]Review Short Refresher of Radiobiology.Averyn VS. Nuclear and Radiological Emergencies in Animal Production Systems, Preparedness, Response and Recovery. 2021
- Radiation Physics - StatPearlsRadiation Physics - StatPearls
Your browsing activity is empty.
Activity recording is turned off.
See more...