NCBI Bookshelf. A service of the National Library of Medicine, National Institutes of Health.
Feingold KR, Anawalt B, Blackman MR, et al., editors. Endotext [Internet]. South Dartmouth (MA): MDText.com, Inc.; 2000-.
ABSTRACT
Thyroid hormones are essential for normal brain development. They influence neurogenesis, neuronal and glial cell differentiation and migration, synaptogenesis, and myelination. Thyroid hormone deficiency may severely affect the brain during fetal and postnatal development, causing retarded maturation, intellectual deficits, and neurological impairment. Neural cells express the thyroid hormone nuclear receptors THRA and THRB, which mediate most actions of T3, the active hormone. Brain T3 derives in part from the circulation, and part from type-2 deiodinase-mediated 5’-deiodination of T4 in glial cells. Type 3 deiodinase inactivates T4 and T3 by 5-deiodination in neurons. Membrane transporters facilitate the passage of T4 and T3 across the brain barriers. The main transporters are the monocarboxylate transporter 8 (MCT8) and the organic anion transporter polypeptide 1C1 (OATP1C1). MCT8 facilitates T4 and T3 transport whereas OATP1C1 transports T4 but not T3. T3 regulates the expression of a large number of genes in the brain, mostly during developmental stages, but also in the adult. Rodent models of disease have provided most of our knowledge on thyroid hormone action in the brain. However, species-specific differences in brain maturation and organization make it difficult sometimes to extrapolate the data obtained in rodent models to the human. This review will present a summary of the main concepts developed from rodent studies, with a focus on the human brain.
For complete coverage of all related areas of Endocrinology, please visit our on-line FREE web-text, WWW.ENDOTEXT.ORG.
INTRODUCTION
Thyroid hormones are crucial for brain development, and influence brain function throughout life. In adults, hypothyroidism causes lethargy, hyporeflexia, and poor motor coordination (1,2), is associated with bipolar affective disorders, depression, or loss of cognitive functions (3,4). Subclinical hypothyroidism is often associated with memory impairment. Conversely, hyperthyroidism causes hyperreflexia, irritability, and anxiety among other symptoms (5). Hypo- or hyperthyroidism can lead to mood disorders, dementia, confusion, and personality changes. Most of these disorders are usually reversible with proper treatment, indicating that adult-onset thyroid hormone alterations affect neural function but do not leave permanent structural defects.
The actions of thyroid hormones during mammalian brain maturation are qualitatively different. They influence many developmental processes, usually during limited time windows. They are required for the timely synchronization of independent events and facilitate the transition between fetal and postnatal stages (6,7), similarly to promoting amphibian metamorphosis (8,9). Thyroid hormone deficiency during critical transition periods may lead to irreversible brain damage, the consequences of which depend on the severity and duration of the deficiency, and most importantly its time of onset (10-14).
Until recently, the rat was the most widely used animal model in the study of thyroid physiology and the actions of thyroid hormones in the brain. However, for more than 20 years, the mouse is the preferred animal model, and the use of knockout and knockin mice has facilitated our understanding. However, it is important to be aware of species specificities which make it difficult to extrapolate the results to the human situation. The timing of development in relation to birth among mammals presents substantial differences, even if the sequence of events might be similar (15-17). The web resource www.translatingtime.org (18) provides tools to compare neurodevelopmental time across species. As an approximation, the newborn rat may be compared with a second-trimester human fetus, and the maturation of a newborn human cerebral cortex to that of a 12-13-day old rat pup (19). For integrative reviews on molecular and evolutionary aspects of the cerebral cortex and cerebellar development and the effects of thyroid hormones see (20-23).
Whenever possible, this chapter focuses on observations in humans. Gene and protein notations follow the HUGO nomenclature (http://www.genenames.org): for human genes, names are in italics and in capital letters, protein names are written in non-italic capital letters irrespective of species.
STRUCTURAL DEFECTS CAUSED BY THYROID HORMONE DEFICIENCY
As an approach to understanding how thyroid hormones influence brain development, the changes caused by thyroid hormone deprivation are informative. In rats, perinatal hypothyroidism causes reduced myelination and diverse structural defects (for a classical review on this topic see (24). A reduction of the neuropil causes increased cell density in the cerebral cortex (25,26). Reduction in total cell numbers of regions with significant neurogenesis during the postnatal period, such as the olfactory bulb and the granular layers of the hippocampus and cerebellum (26-28). Transient structures show retarded disappearance. An important example is the subplate, a transient structure of the cortex involved in the organization of thalamic afferents to the cortex (29). In the cerebellum, regression of the external granular, or germinal layer, is retarded by a few days (30). The Cajal-Retzius cells, formed early during cortical development and involved in the regulation of the inside-out migration of neurons have delayed appearance (Fig. 1 left panel) (31). The GABAergic interneurons have altered distribution and connectivity, and the parvalbumin subclass is reduced in number (32-35). The maturation of several types of neurons is compromised, with stunted dendritic and/or axonal growth and maturation, for example cholinergic cells (36), cerebellar Purkinje cells (Fig. 1 central panel) (37,38), and cortex layer V pyramidal cells (39,40). Changes in dendritic spine number are also observed in the cortex and hippocampus after adult-onset hypothyroidism and are reversible with thyroxine treatment (41,42). Hypothyroidism also causes delayed and poor deposition of myelin (43-46) whereas hyperthyroidism accelerates myelination (47). After prolonged neonatal hypothyroidism, the number of myelinated axons in adult rats is abnormally low in hypothyroid animals although most of the myelinated axons appear to have a normal thickness of the myelin sheath (Fig. 1 right panel).

Fig. 1.
Examples of the effects of hypothyroidism on developmental timing, cell differentiation, and cell migration. Left panel: In the cortical plate, hypothyroidism of fetal onset reduced the number of Cajal-Retzius cells, Rln mRNA (reelin), and reelin protein at P0. Normal amounts of reelin were present in the cortex at P5, indicating that thyroid hormones control the timing of Rln expression (from (31)). Central panel: Neonatal hypothyroidism causes arrested Purkinje cell differentiation (upper panels), and delayed disappearance of the external (germinal) granular layer due to delayed migration of granular cells. The right panel shows a permanent myelin deficit in hypothyroidism. Myelin staining of the anterior commissure of adult rats with fetal/neonatal-onset hypothyroidism and their respective euthyroid controls shows a thinner and paler commissure in the hypothyroid rats. Electron microscope imaging reveals that a higher proportion of axons of hypothyroid rats were of lower diameter and unmyelinated (from (48)).
Less information is available on how hypothyroidism affects the structure of the human brain. Post mortem examination in two cases of deficient thyroid hormone transport to the brain caused by MCT8 mutations revealed anatomical changes compatible with thyroid hormone deficiency (49) (Fig. 2): delayed maturation of the neocortex and cerebellum, delayed myelination, altered neuronal differentiation with lower expression of neurofilaments, and reduced synaptogenesis with reduced synaptophysin expression. Specific cellular changes included a reduced number of Cajal-Retzius cells and parvalbumin interneurons in the cortex. Cerebellar Purkinje cells had a normal morphology in contrast to the usual finding in hypothyroid rodents. These findings indicate that it is possible to extrapolate, but with caution, the brain lesions observed in hypothyroid rodents to humans.

Fig. 2.
MCT8 mutations cause anatomical changes compatible with cerebral hypothyroidism already during fetal stages. The left upper panels show staining of the cortex with neurofilament (brown color) in a control fetus (a) and a fetus with a MCT8 mutation (b). The normal cortex shows staining of Cajal-Retzius cells, which were absent in the MCT8 fetus. The left lower panels show staining of the cerebellum with anti-myelin basic protein. The normal fetus (c) shows immunoreactivity, which is not present in the MCT8 fetus (d). The right panels correspond to an 11-years old boy with MCT8 mutation (panels f, h, k l, n) and control of the same age (panels e, g, i, j, m). Panels e, f: cross-section of the pyramidal track stained with neurofilament; in the MCT8-mutated patient the axonal diameter is smaller than in the control. Panels g, h: staining of the cortex with anti-parvalbumin antibody shows that parvalbumin interneurons are present in the normal cortex but not in the MCT8 cortex. Panels i-l: cerebellar staining for myelin basic protein (i, j) and myelin (k, l). Panels m. n: cerebellar staining for synaptophysin showing the presence of synaptic boutons around the body of a Purkinje cell in the control (m) but not in the patient (n). Data from (49).
THYROID HORMONES IN THE DEVELOPING BRAIN
Maternal and Fetal Thyroid Hormones
The relative roles of the maternal and fetal thyroid hormones on brain development is a most important question. Fetal brain thyroid hormones depend on the transplacental passage of the maternal hormones and the onset of fetal thyroid function. In rodents, the onset of active fetal thyroid secretion occurs at E17.5, but thyroid hormones are present earlier in the embryos, a few days after implantation. This hormone is of maternal origin, as T4 and T3 cross the placenta (50-55). In normal rats at term, the maternal T4 accounts for about 17.5% of the total extra-thyroidal fetal thyroxine pool (56). However, in the fetal brain the concentrations of T4 and T3 are low before the onset of fetal thyroid function, and rapidly increase several-fold from E18 to E21 (57).
In humans, Vulsma et al. (58) demonstrated the transplacental passage of T4 by showing that in neonates with thyroid agenesis or a total organification defect, T4 was present in cord serum at 30-50% of the normal concentration. The only possible origin of this T4 was maternal. Transfer of T4 from the mother to the fetus protects the fetal brain in congenital hypothyroidism, preventing neurological damage before birth, and making it possible that early postnatal treatment is effective (59). The importance of maternal hormones becomes apparent in situations where protection does not take place. This occurs in the combined maternal and fetal thyroid failure, as in feto-maternal PIT-1 deficiency (60), or presence of high titers of thyroid stimulation blocking antibodies (61). In these cases, mothers and neonates had extremely low thyroid hormones. The infants suffered profound developmental delays, permanent sensorineural deafness, and irreversible neuromotor impairment.
STUDIES IN RODENTS
If thyroid hormones from the mother offer protection to the brain in cases of fetal thyroid failure, one could then ask about the relevance of maternal thyroid hormones for brain development in the presence of a normal fetal thyroid gland, or before the gland becomes functional. If maternal thyroid hormones contribute to fetal brain development, then alterations of maternal thyroid physiology might have consequences on the fetal brain. A relevant question is whether the isolated failure of the mother’s thyroid gland causes thyroid hormone deficiency in the fetal brain. Thyroidectomy in pregnant rats, a situation of maternal hypothyroidism with intact fetal thyroid, causes a reduction in the total extra-thyroidal T4 and T3 of the fetuses and developmental delays, but at E21 there was no reduction of T4 and T3 in the brain compared to fetuses from control dams (62). In these experiments, fetal thyroid activity compensated for any possible reduction of brain thyroid hormones caused by maternal thyroidectomy. More recent studies (63), confirmed that thyroidectomy of the dams did not alter the concentrations of T4 and T3 in the fetal cerebral cortex at E21, whereas dams treated with methyl-mercapto-imidazole (methimazole), which causes maternal and fetal hypothyroidism, had greatly reduced brain T4 and T3 concentrations. Microarray analysis of the fetal cerebral cortex successfully identified many genes with altered expression in the combined maternal and fetal hypothyroidism methimazole-induced model but with unchanged fetal expression in the offspring of thyroidectomized mothers. The conclusion emanating from the comparison of these two models is that fetal brain gene expression in the rat at term is under predominant control of the fetal thyroid gland.
In contrast to these studies, the offspring from rat dams thyroidectomized at day 16 of pregnancy showed altered cortical lamination and other structural defects when analyzed at P40 (29). The bulk microarray studies of the cortex might have failed to identify subtle, layer-specific changes of gene expression requiring time to transduce into structural alterations. Other studies support the effect of maternal thyroid hormones before the onset of fetal thyroid function. Transient maternal hypothyroidism of pregnant rats, from E12 to E15, caused the displacement of cells in the neocortex and hippocampus of the offspring, associated with audiogenic seizures when analyzed at 40 days of age, (64). Moderate thyroid hormone deficiency during pregnancy caused neuronal ectopias in the corpus callosum of the progeny (65). Thus, although the fetal thyroid gland exerts the main control on fetal brain development, there is experimental support for subtle actions of maternal thyroid hormone before the onset of fetal thyroid function. These actions do not have yet a correlate in terms of defined molecular events, and the possibility remains that they reflect indirect effects of systemic hypothyroidism rather than direct actions of the hormones on neural targets (66).
STUDIES IN HUMANS
Early studies on the human fetal thyroid gland development showed that colloid formation, iodide concentration, and synthesis of thyroglobulin and T4 could be demonstrated by the 11th week of gestation (67,68). Recent gene expression studies showed that the genes encoding thyroid transcription factors, thyroglobulin, thyroid peroxidase, and the TSH receptor are expressed already by week 7, and that the sodium-iodide symporter is strongly upregulated by the 10th week (69). From the 11th week, serum total and free T4 and T3 increase with time (Fig 3) (70,71), and T4 reaches maternal concentrations by the 36th week (72). The increased T4 and T3 observed in Fig. 3 are clearly due to fetal thyroid gland activity, and it is uncertain to what extent the maternal hormones contribute to the fetal hormone pool in the presence of a normally active fetal thyroid gland. Supporting the contribution of maternal hormones in a situation of normality, its interruption might explain the relative hypothyroxinemia of premature babies (73). Early in gestation, T4 is present in low amounts in the coelomic fluid from the 5th-6th gestational weeks, and the amniotic fluid contains T4 and T3 from the 10th-12th week (74,75).
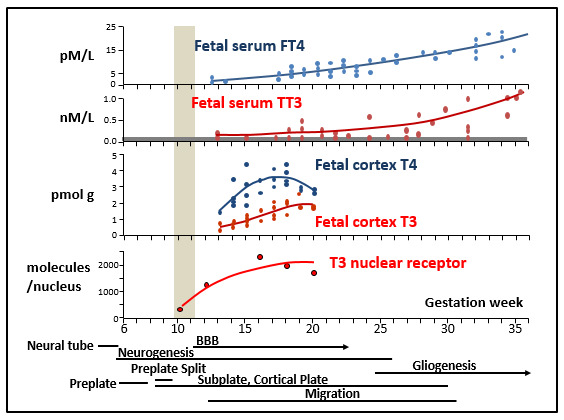
Fig. 3.
Developmental events in the human fetal cortex and changes of thyroid hormone concentrations and nuclear receptor. The lower part of the figure shows the approximate timing of developmental events, the formation of the neural tube; the start of neurogenesis on GW5-5; the formation of the preplate, i.e. the formation of the first neurons, the Cajal-Retzius cells in the marginal zone, and the subplate cells; the preplate split, when the first projection neurons migrate and start filling the space between the Cajal-Retzius and the subplate cells, leading to the formation of the cortical plate; the appearance of tight junctions between vascular endothelial cells, and the blood-brain barrier (BBB), neuronal migration, and gliogenesis with the appearance of astroglial cells and oligodendrocytes. The vertical gray band marks the onset of thyroid gland function. Data on thyroid hormone concentrations in serum are from (71). Data on thyroid hormone concentrations in the cortex are from (76). Data on T3 nuclear receptor are from (77).
The recognition of the transplacental passage of thyroid hormones, described above, raised the question of the role of maternal T4 on fetal brain development (78). The implication is that maternal T4 exerts actions on the fetal brain before onset of the fetal thyroid gland, and even complements the fetal hormones after midgestation (79). Reports are indicating adverse effects of hypothyroxinemia on cognition and behavior of the progeny (80-82), or no effects (83). The issue is unsettled, mainly due to disparities in methodological approaches and the presence of compounding factors. Among these, the definition of hypothyroxinemia, the presence or not of hypothyroidism, iodine deficiency as the main cause of hypothyroxinemia (84), environmental pollutants, and indirect effects of hypothyroxinemia through complications of pregnancy (85-88). Clinical trials involving treatment of hypothyroxinemic pregnant women with T4 gave no clear answers yet (89-91).
Transport of Thyroid Hormones into the Brain
Other chapters of this book describe in detail the issue of thyroid hormone transport (92). This section contains only a few specificities concerning transport in the brain. The crucial role of transporters in the brain is to facilitate that T4 and T3 from the circulation cross the brain barriers (93-97). There are two main barriers in the mature brain: the blood-brain barrier (BBB) and the blood-cerebrospinal-spinal fluid barrier (98,99). The presence of tight junctions between endothelial vascular cells, or choroid plexus epithelial cells form barriers that hamper paracellular transport. Crossing these barriers requires transporters at the opposite sides of the plasma membrane facilitating the influx and efflux of the transported solutes. The gradient concentration of the free solutes determines the flux direction. The BBB surface is 5000-fold larger than that of the blood-CSF barrier (100). The larger exchange surface and the short distance of individual neurons from microvessels (8-20 µm (98)), makes the BBB the most relevant site of transport from the blood to the brain parenchyma.
Tight junctions between vascular endothelial cells, i.e., the BBB proper, are present in rodents by E15-E16 (101,102), and in humans by GW12 (99) (Fig. 3).

Fig. 4.
Thyroid hormone transport and metabolism in the rodent brain. MCT8 in the blood-brain barrier facilitates the transport of circulating T4 and T3 to the brain parenchyma and OATP1C1 the transfer of T4. T4 in the astrocytes is converted to T3 by DIO2, providing additional T3 to nearby neural cells. DIO3 in neurons degrades T3 to T2 and T4 to rT3. Modified with permission from (103).
Further maturation and consolidation require the presence of pericytes and astrocytes in the vascular unit (104). Lopez-Espindola et al. (96) found immunoreactive MCT8 at GW12 in the human fetal brain endothelial cells, possibly highlighting the importance of MCT8-mediated transport at a time when the BBB starts limiting the entry of solutes to the brain. MCT8 was also present in other barriers such as the blood-cerebrospinal fluid barrier, the transient outer cerebrospinal fluid-brain barrier, and the ependymocytes. These findings suggest the presence of alternative routes by which T4 and T3 could reach the brain parenchyma in the fetus.
There is a fundamental difference between rodents and humans in the transport of thyroid hormones through the BBB. The rodent BBB contains MCT8 and OATP1C1 (Fig. 4). MCT8 transports T4 and T3 whereas OATP1C1 transports T4, reverse T3 (rT3), and T4 sulfate. In humans, as in other primates, the BBB contains MCT8 but lacks OATP1C1 (93,105). One explanation why MCT8 mutations cause profound neurological impairment in patients but not in mice is that T4 transport through OATP1C1 compensates similar mutations in mice by making T4 available as a DIO2 substrate. There is experimental support for this mechanism as follows: i. MCT8-deficient mice lack neurological impairment (106), with only slight alterations of brain gene expression (107), contrary to what would be expected; ii. DIO2 activity and T3 generation increase in the brain of MCT8-deficient mice (108,109); and iii. To achieve cerebral hypothyroidism in mice, a compound deficiency of MCT8 and DIO2, or MCT8 and OATP1C1 is needed, i.e., a knockout of Slc16a2 and Dio2 or Slc16a2 and Slco1c1 (66,107,110,111).
Role of Deiodinases
The deiodinases present in the cerebrum are DIO2 and DIO3 (112). The adult mouse cerebellum also contains significant DIO1 activity (113). Different cells express DIO2 and DIO3 in the brain. Glial cells and some interneurons express DIO2, and excitatory neurons express DIO3 (114-118). The glial cells expressing DIO2 include radial glial cells, astrocytes, and tanycytes (Fig. 5) (114,116,118). Dio2 expression occurs in most brain areas, and increase in hypothyroidism (Fig. 5). Dio3 shows a restricted high expression at P0 in discrete nuclei, related to sexual differentiation of the brain, but the physiological relevance of this expression has not been studied (Fig. 5 (119)).

Fig. 5.
Dio2 and Dio3 expression by in situ hybridization. Panels A, E, Dio2: in euthyroid rats (panel A) Dio2 is distributed all over the brain especially in the upper layers of the cortex, the hippocampus, the thalamic ventromedial nucleus, with increased expression in hypothyroid rats (panel B). The median eminence, infundibulum, and walls of the 3rd ventricle show high expression in tanycytes, a specialized type of glial cells (panel C). Dio2 increases in hypothyroidism also in this region (panel D). In the rest of the brain, Dio2 is expressed in the astrocytes as shown in panel E after GFAP counterstaining of the in situ hybridization. Panels F-H; Dio3 expression in the mouse at P0: Selective expression in the accumbens nucleus and the anterior pole of the bed nucleus of stria terminalis (Acb/BST, panel F), BST, median and medial preoptic nuclei (MnPO and MPO, panel G) and amygdala (panel H). Panels A, B, and E (114,115). Panels C and D are adapted from (116). Panels F,G,H are from (119).
Astrocytes, which exceed by ten-fold the number of neurons supply T3 to other neural cells by DIO2 deiodination of T4 (Fig. 3) (114,115,120). In rodents, the majority of astrocytes arise during the first postnatal week, and DIO2 expression and activity increase accordingly. Tanycytes are specialized glial cells lining the walls of the 3rd ventricle and the cause for the high hypothalamic DIO2 activity (121). Tanycytes in the median eminence form a blood-hypothalamus barrier, modulated in response to metabolic factors, which control the access of blood-borne substances to the arcuate nucleus (122). These cells are linked to the central control of feeding, body weight, and energy balance, and may act as stem cells to produce hypothalamic neurons (123). The hypothalamic paraventricular nucleus does not express DIO2, and T3 generated in astrocytes or tanycytes might control TRH production in this nucleus (114).
It is estimated that in DIO2-expressing tissues, such as the brain, brown adipose tissue, and pituitary, 50% or more of T3 derives from local T4 deiodination (124-127). In the adult rat brain, as much as 80% of nuclear-bound T3 is formed locally from T4 (128). Through DIO2 and DIO3 expression, the concentrations of T3 meet the local requirements of the particular developmental stage independently of fluctuations of circulating T3. Some discrete examples are the roles of Dio3 (129) and Dio2 (130) in the mouse cochlea with a peak of DIO2 activity on P7 before the onset of hearing, and the sequential expression of Dio3 and Dio2 in the control of retinal cone specification (131).
The balance between DIO2 and DIO3 activity during development is critical to ensure adequate amounts of T3 in neural tissue. Extremely high levels of Dio3 expression and activity are present in the uterine implantation site, and later on in the uterine epithelium, preventing that inadequate amounts of T4 and T3 reach the embryos at early stages (132). By the end of pregnancy in the rat, DIO2 activity increases markedly in the fetal brain, in parallel with a 10-fold increase of T4 and 28-fold increase of T3 (133,134). The increase in DIO2 activity occurs after the start of gliogenesis and generation of astrocytes by E17 (135).
The fetal brain produces most T3 locally from circulating T4. Circulating T3 has poor access to the fetal brain. In a classical experiment, Morreale de Escobar and coworkers (136) showed that generation of T3 from T4 provides a means to regulate the concentrations of T3 within narrow limits (Fig. 6).

Fig. 6.
T3 concentrations in the fetal brain after infusion of pregnant rats with increasing doses of T4 or T3. MMI-treated pregnant rats were infused with T4 or T3 using osmotic pumps from gestational day 15 through 21. The doses shown correspond to the starting dose on E15 and remained constant during the infusion period. Infusion of T4, but not T3, increased T3 in the brain. Data from (136).
In this experiment, they infused increasing doses of T4 or T3 to hypothyroid pregnant rats and then measured the resulting concentrations of T3 in the hypothyroid fetal brain. After T4 infusion to the mother, the fetal brain T3 concentration increased, reaching a normal concentration with a relatively low dose of T4. In contrast, it was difficult to increase T3 in the fetal brain after T3 infusion only over a wide dose range. DIO2-dependent generation of T3 in the fetal brain from maternal T4 also occurs in euthyroid pregnant mice. Administration of T3 to the mothers increased T3 in the fetal brain, not by direct transfer, but by stimulating Dio2 gene expression and DIO2 activity (137).
The reason why the fetal rodent brain is not permeable to T3 is unknown. One possibility would be lack of expression of the T4 and T3 transporter MCT8, but the fetal brain expresses high concentrations of MCT8, even larger than in the postnatal period (138). One possible mechanism would be selective T3 degradation by DIO3 after crossing the brain barriers. Different cellular transport routes for T4 and T3 could be involved in the selectivity of this mechanism. Knocking out Dio3 increases the sensitivity of the fetal and postnatal mouse brain to thyroid hormones (139-141).
STUDIES IN HUMANS
In the developing human cerebral cortex, DIO2 mRNA and activity are present already by GW7-GW8 (142). The relative expression of DIO2 and DIO3 is an important determinant of the T3 concentrations as development proceeds and has a strong regional component, as shown by Kester et al. (76) (Fig. 7).

Fig. 7.
T3 concentrations in the fetal cortex and cerebellum respectively at different gestation weeks. Data extracted from (76).
These authors measured T4 and T3 concentrations and deiodinase activities in several brain regions from GW12-GW20 fetuses. The cerebral cortex had a progressive increase in T3 concentrations (Fig. 3), which correlated with an increased concentration of T4 and DIO2 activity. The parallel increase of T4 and DIO2 activity indicated an increased DIO2 transcription since T4 inhibits DIO2 activity. DIO3 activity was very low, near the limit of detection. The cerebellum showed a completely different picture: T3 was very low, and D3 activity was the highest among all regions. The difference between the cortex and the cerebellum (Fig. 7) supports the notion that the relative expression of DIO2 and DIO3 regulate the tissue response to thyroid hormones during development.
These changes occur in parallel to the accumulation of the nuclear receptor (77), indicating an increased sensitivity of the cortex to thyroid hormones from GW12 onwards (Fig. 3). This occurs after the preplate split, i.e., when the first migrating neurons start the formation of the cortical plate overlapping the period of neurogenesis and cell migration. Gliogenesis and the generation of astrocytes, the main cells expressing DIO2, occur around GW25 in the cortex (143).
The cells expressing DIO2 before astrocytes are formed are the radial glial cells (96,118), especially the outer radial glial cells (Fig. 8). This is most surprising because the outer radial glial cells, the universal stem cells of the cortex, are especially abundant in the cortex of primates, including humans. They are responsible for the enlargement of the subventricular zone and cortex expansion in these species (144). Furthermore, there is tightly correlated coexpression of DIO2 and the T4 transporter SLCO1C1 (OATP1C1) (Fig. 9) (118), indicating that the outer radial glia is the origin of the T3 formed locally in the human cortex at midgestation.

Fig. 8.
A scheme representing a cross-section of the human fetal cerebral cortex at 18 -19 weeks of gestation. MZ, marginal zone; CP, cortical plate; IZ, intermediate zone; OSVZ, outer subventricular zone; ISVZ, inner subventricular zone; VZ, ventricular zone. In red, the ventricular or apical radial glia, with their bodies located in the ventricular zone, extending an apical process to the ventricular Surface and a basal process to the Surface of the brain. In green, the outer radial glia, lacking the apical process. Migrating neurons arriving at the cortical plate along the radial glial processes. In yellow, intermediate progenitors in the internal subventricular zone. Drawing reproduced with permission from (144).
The locally produced T3 could be involved in neurogenesis or could act on the newly formed neurons, which express THRA (145), or interneurons expressing THRB (118) to regulate their migration or terminal differentiation. T4 could reach the radial glia from the circulation. Alternatively, T4 could reach the apical processes of the ventricular radial glia by crossing the neuroepithelium from the inner CSF, or their basal processes from the outer CSF in the subarachnoid space (146). After the onset of gliogenesis, astrocytes express DIO2 (96,118). In contrast to the specific expression of SLCO1C1/OATP1C1, SLC16A2/MCT8 is widely expressed in most cell types (Fig. 9).

Fig. 9.
Single-cell transcriptome profiling of DIO2, SLCO1C1, SLC16A2, THRA, and THRB expression (data from reference 118). In color are bulk cell clusters obtained using Uniform Manifold Approximation and Projection plots from the single-cell RNA sequencing datasets of human fetuses at gestational weeks 17–18 by Polioudakis et al. (147). Endo: endothelial cells, DIV: proliferating cells, ExM-U: excitatory neurons upper cortex enriched, InCGE: interneurons from the caudal ganglionic eminence, InMGE: interneurons from the medial ganglionic eminence, IP: intermediate progenitors, Mg: microglia, Neu: excitatory projection neurons, OPC: oligodendrocyte precursor cells, oRG: outer radial glial cells, Per: pericytes, vRG: ventricular radial glial cells. Blue dots represent single cells. DIO2 and SLCO1C1 coexpress in the outer radial glial cells. DIO2 is also expressed in some interneurons.
THYROID HORMONE RECEPTORS IN THE BRAIN
Flamant and coworkers have reviewed the relative roles of the receptor isoforms on developing and adult brain, using mouse genetic models (148,149). Two different genes THRA and THRB encode two receptor types, alpha (THRA1) and beta (THRB1 and THRB2), respectively. THRA also encodes THRA2, which does not bind T3 and is not a T3 receptor.
The cellular and regional complexity of the brain and its asynchronous maturation requires a previous understanding of the ontogeny and patterns of expression of the receptors to understand their physiological role. The low number of receptor molecules per nucleus, and the relative lack of specificity of receptor antibodies have precluded their use to analyze receptor expression by conventional immunohistochemistry. Analysis of the regional and cellular expression requires in situ hybridization histochemistry. This technique provides data on receptor mRNA expression that may not reflect accurately the concentration of the receptor protein (150,151).
Studies on receptor protein concentration used T3 binding assays in nuclei isolated from whole brain or brain regions, with a sensitivity of about 100-200 molecules/nucleus. Binding assays detect indistinctly the THRA1 and THRB subtypes, but discrimination between THRA1 and THRB may be possible to some extent using a combination of T3 binding and immunoprecipitation, or by competitive binding assays using the acetic acid derivative triac. THRA1 has the same affinity for triac as for T3, and THRB up to ten-fold higher affinity for triac than for T3 (152). Receptor expression studies have also used knock-in mice in which the receptor protein was tagged with green fluorescent protein (GFP) (145), or with hemagglutinin epitopes (151).
There are discrepancies between the cellular abundance of receptor-encoded mRNAs and the corresponding receptor proteins (150). At the mRNA level, all receptor isoforms are present in the brain. Apart from the highly abundant, non-receptor encoding, Thra2 transcript, the predominant receptor isoform is Thra1, widely distributed in the CNS from E14 to adulthood (151,153-155). From E19 to P0, Thra1 is present in the outer part of the cerebral cortex and hippocampal CA1 field. During the late fetal stage, Thra1 is present in the piriform cortex, superior colliculus, pyramidal cell layer of the hippocampus, and the granular layer of the dentate gyrus. In adult rodents, Thra1 expression is prominent in the cerebral cortex, cerebellum, hippocampus, striatum, and olfactory bulb. Knock-in mice studies with green fluorescent protein-tagged THRA1 (145) showed that most neurons express the THRA1-GFP as they become postmitotic in tanycytes of the third ventricle and very low expression in astrocytes. In the cerebellum, the conjugated protein was present in migrating granule cells of the molecular layer and mature granule cells of the internal granule cell layer but not in the proliferating cells of the external germinal layer (also known as the external granular layer). Juvenile, but not adult Purkinje cells expressed THRA1-GFP. In the study by Minakhina et al. (151) the hemagglutinin-tagged THRA1 protein was the predominant receptor subtype in the mouse brain, and THRA2 concentration was 5-10-fold higher.
In cultured cells T3 receptors are present in neurons, astrocytes, oligodendrocytes, sensory neurons, Schwann cells, and microglia (156-160). Primary cultures of mouse astrocytes, which respond transcriptionally to T3, express Thra and Thrb (7). Receptor-specific actions can be demonstrated on some genes such as Dio3, which is specific for THRA1 (161-164). Some cells express specific receptor isoforms, for example, the mouse retina expresses Thra1 widely whereas immature photoreceptor cells selectively express Thrb1 (165,166).
Thrb1 expression during rodent development is low during the fetal period and increases during the postnatal period and through adulthood. Between E17 and E20, only low levels are present in the brain, especially in the hippocampal pyramidal layer. On P0, an increase occurs in the accumbens, striatum, and hippocampus. From around P7 Thrb1 appears in the cerebral cortex. The patterns of expression of Thra1 and Thrb1 overlap (Fig. 10), but in some cells, one of the isoforms is predominant.

Fig. 10.
T3 receptor mRNA expression in the mouse brain, by in situ hybridization with Thra1 and Thrb1-specific probes. In the cerebrum (left panels) there is an overlapping distribution of both receptor subtypes, with some differences in the hippocampus, amygdala, and hypothalamus. In the cerebellum (right panels) Thra1 is expressed in the granular layer (left upper panel), whereas Thrb1 is expressed in the Purkinje cell layer.
As an example, in the cerebellum, differentiated granular cells express Thra1 while Purkinje cells express Thrb1. Thrb2, which is abundant in the pituitary, is also expressed in the retina and the hypothalamus (166,167). During fetal stages, low levels of Thrb2 are present in the striatum (153). The web resource of the Allan Brain Institute (https://celltypes.brain-map.org/rnaseq/mouse_ctx-hip_smart-seq) provides a tool to explore the specific distribution of Thra1 and Thrb during mouse development.
Ontogeny of Thyroid Hormone Receptors in the Developing Brain
T3 binding assays detect the T3 receptor for the first time in the rat brain at E13.5-E14.5 and then increase, reaching stable concentrations from E17 to P0 (168). In close agreement, studies in knock-in mice expressing a conjugated TRα1-green fluorescent protein detect the receptor for the first time at E13.5 in the cortical plate (145). This date marks the onset of brain responsiveness to thyroid hormones (141). The receptor increases at birth and the highest concentrations are reached at P6 (169,170), but receptor occupancy is maximal at P15 (171).
In the human brain, the receptor protein quantified by nuclear binding assays, and receptor mRNAs by PCR, are detected during the first trimester (77,142,172). The receptor protein, with a binding profile typical of THRB, is present at low levels in the fetus around GW10 and increases in concentration 10-fold up to GW18-GW18 (77) (Fig. 3), during neurogenesis and neuron migration. At these stages, subsets of CALB2 (calretinin) and SST (somatostatin) interneurons selectively express THRB (Fig. 9) (118), but its contribution to the total receptor protein measured by binding assays is unknown.
Occupancy of receptors with the ligand in the brain antecedes the occupancy in other tissues. In the human brain, the T3 ligand is present from GW10, at concentrations enough to result in about 25% occupancy of receptor (77,173), and when no T3 is detected in other organs. As stated above, this is due to the early presence of DIO2 activity (76). A similar situation occurs in fetal lambs at gestational day 100. The brain had a 74% receptor occupancy compared with 10% in the liver and lung (174). In rats total receptor occupancy by the hormone increases in parallel with the postnatal increase in plasma and cytosol total and free T3, and reach a maximum of 50-60% at postnatal day 15 (171).
THYROID HORMONE ACTION ON BRAIN GENE EXPRESSION
Aporeceptor and Holoreceptor
Thyroid hormones regulate the expression of many genes in the rodent brain, mainly during the postnatal period, but the brain is also sensitive in the fetus and in adult animals (6,7,107,175-182). T3 interacting with the nuclear receptors, i.e., the holoreceptor, has a modulatory role on the receptor transcriptional activity, upregulating or downregulating gene expression. In the absence of the hormone, the aporeceptor has intrinsic transcriptional activity, relevant in events such as amphibian metamorphosis (183), PC12 cell differentiation (184) or development of the inner ear in mice (185). To some extent, the hypothyroid phenotype is the consequence of aporeceptor activity (30,186). Similarly, mutations of receptors that abolish T3 binding cause developmental abnormalities (166,187,188) (see the chapter on the syndromes of resistance to thyroid hormones).
Non-genomic Actions
It is unclear to what extent non-genomic actions might also mediate effects of T4 and T3 in the brain (189-191). Interaction of T4 with integrin αvβ3 might have a role in the expansion of progenitors in the neocortex (192). Proposed non-genomic actions of T3 include the maturation and plasticity of hippocampal pyramidal neurons (193), and the regulation of the onset and duration of the sensitive period of imprinting in chicks (194).
For many genes, the role of thyroid hormones during development is to regulate the timing of gene expression with a strong regional specificity, for example on the myelin genes (46) (see also Fig. 1). In the absence of DIO3, developmental expression of some T3 target genes is accelerated (141). The response of many target genes is region-dependent and shows partial overlap in different brain areas (9). Even within the same area, the same gene may be responsive only in a fraction of cells, as shown by Nrgn (RC3/neurogranin (175)). Transcription of thyroid hormone target genes is likely the result of the combinatorial activity of transcription factors, which include the T3 receptor whose relative weight may depend on the specific region and developmental period. It also reflects the diversity existing within apparently homogeneous cell classes.
Thyroid Hormone-Responsive Elements
Early studies showed the presence of thyroid hormone-responsive elements in the promoter or intronic regions of thyroid hormone-dependent brain genes. To name a few, the myelin basic protein (195); the Purkinje cell-specific gene PCP2 (196); the calmodulin-binding and PKC substrate RC3 (197); the prostaglandin D2 synthetase (198,199); the transcription factor Hairless (200); the neuronal cell adhesion molecule NCAM (201); and the early response gene NGFI-A (202). More recently, Chromatin immunoprecipitation assays identified receptor binding sites in the developing mouse cerebellum (203) or cerebellar cell lines expressing THRA1 or THRB1 (162). Other targets are regulated at the levels of mRNA stability (acetylcholinesterase), protein translation (MAP2 (204)) or mRNA splicing (TAU (205)). Regulation of splicing might be due to a primary action on the transcription of splicing regulators (206).
Genes Responsive to Thyroid Hormones in the Fetal and Postnatal Rodent Brain
Thyroid hormone regulation of several genes occurs in the rodent fetal brain in vivo (207-209). In the fetal cerebral cortex at the end of gestation, thyroid hormone controls the expression of genes involved in the biogenesis of the cytoskeleton, cell migration, and branching of neurites. In some studies, a large percentage of the thyroid hormone-dependent genes were related to Camk4 signaling pathways (63,210). Camk4 is regulated by T3 in cultured primary neurons (211,212), but is not in the postnatal rat brain in vivo (213). Results of extensive analysis by RNA-Seq of primary embryonal cerebrocortical cultures disclosed many thyroid hormone targets with roles during cortical development (6,20). The responsiveness of the fetal brain to thyroid hormones increases in Dio3 knockout mice (139,141).
Many of the thyroid hormone-regulated genes identified during the postnatal period in the rodent brain are sensitive to the hormone only during a time window that spans the first 2-3 weeks after birth. Many of these genes are not dependent on thyroid hormone in the fetal or in the adult brain, possibly due to a DIO3-related mechanism (141). Chatonnet et al. (9), performed an analysis of the published data on thyroid hormone action on gene expression in the brain and cultured cells and arrived at a list of at least 37 genes consistently found in different studies as targets of the T3 receptors. Some of them are candidates for transcriptional regulation because they contain a thyroid hormone-responsive element. These genes include Adamtsl4, Dbp, Fos, Hr, Kcna1, Klf9, Scd1, Stat5a, and Txnip. Additional genes found regulated transcriptionally by T3 in several independent studies are Shh, Hr, Dbp, Gbp3, and Nrgn.
In our studies, employing RNA-Seq of primary cerebrocortical cells (6) we found that T3 up-or down-regulated up to 7.7% of expressed genes 24 hours after treatment. T3 was active in the presence of cycloheximide on about 30% of these genes indicating an effect on transcription. No response to T3 occurred in similar cells from Thra and Thrb knockout mice, as expected for receptor-mediated actions. Additionally, a large proportion of the T3-responsive genes in the presence of cycloheximide contained T3-responsive elements (162). The estimation is that T3 regulates around 2.5% of all expressed cellular genes and at least 1% at the transcriptional level through a T3 responsive element.
Brain deprivation of thyroid hormones caused by systemic hypothyroidism causes larger changes of gene expression than compound inactivation of the MCT8 and OATP1C1 transporters, or inactivation of MCT8 and DIO2. In the MCT8 plus OATP1C1 deficiency, the transport of T4 and T3 is severely compromised, resulting in isolated brain hypothyroidism with low tissue T4 and T3 (66). In the MCT8 plus DIO2 deficiency, the transport of T3 and the generation of local T3 from T4 are blocked, leading also to brain hypothyroidism buy with normal T4 and low T3. Contrary to what would be expected, the changes in gene expression are not equivalent in these two models of brain hypothyroidism, which are also different from systemic hypothyroidism. The latter causes altered expression of about the double number of genes in the cerebral cortex and the striatum than the cerebral hypothyroidism of the double knockouts. One reason is that systemic hypothyroidism causes many effects on gene expression that are secondary to other actions of T3 elsewhere in the body. When transcriptionally-regulated genes were compared, similar profiles were found in the three conditions. In systemic hypothyroidism, among the transcriptional targets of T3, the most affected downregulated genes in the cortex were Kcnj10, Hr, Ky, Cyp11a1, Nefm, Npt, Stac2, Hcrtr1, Shh, Prlr, Sema7a, and upregulated, Aldh1a3, Adamts18, Ntf3, Mc4r, Dio2, Trhr. In the striatum, the most affected downregulated genes were Prlr, Cyp11a1, Cnr1, Sema7a, Gls2, Shh, Igsf9, Enpp2, Gdf10, Arg2, Nefm, and upregulated, Cyp26b1, Syt10, Trhr, Mc4r, Dio2, Gabra5.
Interactions with Glucocorticoids and Retinoids
The interactions of thyroid hormones with other hormonal systems (214) might be relevant to their actions on neural cells. In primary cerebrocortical cultures, one of the most significant processes regulated by T3 was the control of G-protein-coupled receptor activity (164), estimated to be important in the transition from fetal type to adult-type gene expression. A detailed analysis of these interactions is beyond the scope of this chapter, and only one example is mentioned related to the crosstalk with glucocorticoids and retinoid signaling pathways. T3 controls the expression of several enzymes involved in retinoic acid (RA) metabolism: the RA synthesizing enzymes ALDH1A1 and ALDH1A3, and the degrading enzyme CYP26B1 (9,163). The final effect on RA concentrations depends on the relative expression of each of the enzymes, which have developmental and regional variations, and on glucocorticoid signaling. Aldh1a1 is upregulated by T3, preferentially through TRα1, and glucocorticoids potentiated the effect of T3. Additionally, T3 controls the expression of Nr3c1, the glucocorticoid receptor. Aldh1a3 is downregulated by T3 in primary cells and increases in expression in hypothyroidism as indicated in the previous paragraph. On the other hand, Cyp26b1 is upregulated by T3 in primary cells and is downregulated in the hypothyroid cortex but upregulated in the hypothyroid striatum. By increasing ALDH1A1, especially in the presence of glucocorticoids, T3 will increment RA concentrations, whereas acting on ALDH1A3 and CYP26B1, T3 will reduce RA concentrations. A recent study on Dio3 knockout mice has found evidence for these interactions (141).
Actions on Gene Expression in the Adult Brain
In adult subjects, thyroid hormones influence mood and behavior, and thyroid dysfunction affects neurotransmitter systems (215) often leading to psychiatric disorders (216). High doses of T4 improve mood in bipolar depression (217,218). In the adult rat striatum, administration of a large single T3 dose leads to up-regulation of 149 genes and down-regulation of 88 genes (177). Physiological doses of T3 given for several days to hypothyroid animals led to up-regulation of 18 genes, and down-regulation of just one gene. Therefore, acute large doses of thyroid hormone cause large changes in gene expression, with more modest changes with lower doses. Some of the regulated genes are related to circadian rhythms and wakefulness, with one of them (Dbp or D-site binding protein) proposed as a candidate gene in bipolar disorders (219), and likely to be regulated directly by TRα1 (176). Many other genes were involved in striatal physiology as components of several signaling pathways. Fig. 11 shows a putative model of T3 action on the adult striatum.

Fig. 11.
Regulation of gene expression by thyroid hormones in the adult striatum. Signaling pathways are schematically represented and the main groups of regulated genes are shown in numerals. 1: G-protein coupled receptor signaling (Cnr1, Rgs9, Rasd2, Rasgrp1). 2: Ca2+/calmodulin pathway (Nrgn); MAPK pathways (Map2k3, Fos). 4: Early genes (Nr4a1, Arc, Dusp1, Egr1, Homer). 5: Ion channels (Scn4b). Abbreviations: VDCC, voltage-dependent sodium channels, NMDA, N-methyl-D-aspartate, D1 and D2, dopamine receptors 1 and 2. From (177).
ACTIONS OF THYROID HORMONES ON SPECIFIC DEVELOPMENTAL EVENTS
There are several possible approaches for studying the role of thyroid hormones on brain development. One way would be to analyze the effects of hypothyroidism and thyroid hormone treatment on the development of the cerebral cortex, the striatum, the cerebellum, the hippocampus, and other regions. Another way is to analyze common events occurring in the different brain regions, such as neurogenesis, cell migration, and differentiation, among others. In this review, we have opted for this second approach, with a focus on the T3 regulation of genes involved in these processes. Reviews on the cerebral cortex, and the cerebellum are available (20,21,23).
Neurogenesis
Generation of neurons, or neurogenesis, starts in humans around the fifth gestational week (GW5, Fig. 3), and in mice around embryonic day 10 (E10). The bulk of neurogenesis takes place until GW25 or E16/17 respectively, partially overlapping gliogenesis. The granular cell layers of the hippocampus, olfactory bulb, and cerebellum continue accumulating neurons postnatally, a reason why they are especially sensitive to thyroid function. At the onset of neurogenesis, the neuroepithelial cells sequentially express Pax6, Neurog1/2, and NeuroD, undergoing glutamatergic identity, and under the influence of FGF10 undergo a fast transition to radial glia (20,220). These cells remain attached by an apical process to the ventricular surface and elongate as the embryonic brain epithelium thickens, adopting a bipolar morphology (Fig. 8). In the cortex, the elongated basal process reaches the pia providing a scaffold for cell migration (221,222). In addition to this structural and supportive function, the radial glia is the universal cortical stem cell that generates all neurons and glia, directly or through intermediate precursors. (220). In primates, enlargement of the cortex is due to the accumulation of a population of radial glial cells that lose the apical process and accumulate in the outer part of an enlarged subventricular zone (Fig. 8). Human-specific changes occur with further enlargement of the cortex over the great apes (223).
The role of thyroid hormones on proliferation and differentiation of neural precursors in the embryonic neurogenic areas has been shown during tadpole premetamorphosis (224) and concerning the effects of maternal thyroid hormones (192,225,226). NeuroD, mentioned above, is sensitive to thyroid hormones in the cerebellum (227), and THRA1 mutations cause abnormal proliferation and adhesion of human cortical progenitors (228). Iodine deficiency in rats affects hippocampal radial glial cells (229). The first neurons originating in the cortex, the Cajal-Retzius cells, and the subplate cells, are sensitive to thyroid hormones in rodents and in humans (31,49,210).
Limited neurogenesis also occurs in the adult brain, related in humans to neuropsychiatric conditions, cognitive deficits, and depression. Adult neurogenesis takes place in two structures: the subventricular zone, located underneath the surface of the lateral ventricles, and the subgranular zone, adjacent to the granular layer of the hippocampal dentate gyrus. The subventricular zone generates olfactory bulb interneurons in adult rodents, and in humans provides new interneurons to the adjacent striatum (230). The subgranular zone generates dentate gyrus granular neurons in adult rodents and humans (231). Hypothyroidism and thyroid hormones influence neurogenesis in the rodent subventricular zone and subgranular zone (224,225,232-241).
Neural Cell Differentiation
Thyroid hormone controls the expression of many genes with roles on terminal cell differentiation, such as cell cycle regulators, cytoskeletal proteins, neurotrophins, and neurotrophin receptors, and extracellular matrix proteins. Among the cell cycle regulators, E2F1, p53, cyclins, and cyclin-dependent kinase inhibitors are regulated by thyroid hormone in cell culture (242-244).
Neural cell shape is determined by the cytoskeleton, which consists of microtubules (tubulin), microfilaments (actin), and intermediate filaments, specific for neurons (neurofilaments), glia (glial fibrillary acidic protein), or maturing cells (vimentin, nestin). Tubulins α1 and α2 are downregulated by thyroid hormone, and tubulin β4 is upregulated (245,246). Microtubule-associated proteins (MAPs) are also under thyroid hormone control at a posttranscriptional level. For example, thyroid hormone regulates Map2 protein distribution in the Purkinje cell dendritic tree (204), and conversion of immature forms of the microtubule-associated protein TAU (MAPT) to mature forms by alternative splicing of the MAPT mRNA (205). The neurofilament genes Nefh, and Nefm are also under thyroid hormone control in the fetal and postnatal cerebral cortex (63,107).
Astrocytes (247,248) cerebellar Golgi epithelial cells (249), and microglia (160) are thyroid hormone targets. Thyroid hormones influence the in vivo expression of astroglial genes encoding tenascin C, laminin, and L1 adhesion molecule, with effects on neuronal migration and differentiation, and axonal fasciculation (250-252) . In vitro, β-adrenergic receptor antagonists block the effect of T3 on astrocyte differentiation (253). In another studies T3 upregulates Arrb1 (arrestin beta1), facilitating endocytosis of β2 adrenergic receptor (ADRB2) and ERK activation (254).
The control of neurotrophin expression might mediate some of the effects of thyroid hormone on differentiation and survival. Interactions between thyroid hormone and NGF are important for the growth and maintenance of cholinergic neurons in the basal forebrain (36). Unliganded THRA1 in PC12 cells expressing Thra1 blocks NGF-induced differentiation and the blockade is released by T3 (184), suggesting a possible mechanism for the control of differentiation timing. Changes in NGF, NTRK, P75NTR, BDNF, and NTF3 after hypothyroidism have been described (255-257).
Thyroid hormones influence myelination directly through effects on oligodendrocyte differentiation (258,259), and may also have indirect effects by stimulating axonal maturation, which is impaired in hypothyroidism (Fig. 1 right panel (260,261)). A lower axon diameter prevents reaching the critical size to become myelinated (262). Low axon diameter also occurred in a patient with MCT8 mutation (Fig. 3 (49)), which might contribute to the hypomyelination of these patients.
As thyroid hormones are required for terminal differentiation of oligodendroglial cells, they influence the expression of practically all myelin protein genes, but only during the myelination period. In the rat, this period extends from about the end of the first postnatal week up to the end of the first month, with a strong regional component in parallel with the wave of myelination (46,263). The control of myelin gene expression is transient, and in hypothyroidism, there is a developmental delay, but the effect on the myelin content is permanent (Fig. 1).
Early studies showed that T3 inhibits proliferation and promotes differentiation of oligodendrocyte precursor cells (OPC) (264,265) through repression of the E2F1 transcription factor (266). Transcriptomic analysis identified the universal T3-target gene Klf9 (Krüppel-like factor 9) as a mediator of the effect of T3 on OPC differentiation (267). The transcriptional repressors NCoR and HDAC repress the oligodendrocyte differentiation pathway. T3 relieves this repression and induces Sox10, needed for the maintenance of the differentiated state (268). Oligodendrocyte precursor cells express Thra1 and Thrb (269-271), but Thra1 is the predominant receptor gene expressed in the newly formed and myelinating oligodendrocytes.
Neural Cell Migration
Thyroid hormone also influences neuronal migration in the cerebral cortex, hippocampus, and cerebellum. Thyroid hormone deficiency during cortical development leads to less than the normal definition of cortical layers (260,272,273). Thyroid hormone influences the maturation of the radial glia, the path along which radial migration occurs in the cerebral cortex and the hippocampus (229).
An important cellular target of thyroid hormone is the Cajal-Retzius cell. These cells are located in the marginal zone, the future cortex layer 1, and are required for proper migration of neurons in the cerebral cortex and the hippocampus, cortical lamination, and establishment of synaptic connections (274-276). These cells secrete reelin (RLN), an extracellular matrix protein under thyroid hormone control (31). RLN function is essential for the inside-out pattern of cerebral cortex development. The protein disabled (DAB), a component of the RLN signaling pathway is also under thyroid hormone control. The Rln and Dab1 genes are not regulated by T3 at the transcriptional level, but other genes expressed in Cajal-Retzius cells are transcriptional targets of T3 in primary neurons: these include Rgs4, Npnt, Ephb6, Clstn2, and Dnmt3a (6,20). Cajal-Retzius cells, therefore, appear to be important and selective cellular targets of thyroid hormones during development. Their number is low in perinatal hypothyroidism in rat pups (277), and in a human embryo with mutated MCT8 (49).
There are many extracellular matrix proteins regulated by thyroid hormones, with actions on cell migration and neuronal differentiation, synaptic plasticity, etc. The extracellular matrix proteins are heterogeneous, comprising laminin, fibronectin, collagen, neurotrophic factors, adhesion molecules, hyaluronan proteins, proteoglycans, and other components. As already mentioned, thyroid hormones regulate negatively tenascin C, laminin, and L1 (250-252), and the adhesion molecule NCAM (201,278). In addition to these proteins, regulated by T3 in vivo, T3 regulates many other genes of this heterogeneous group in cultured primary cerebrocortical cells (6) twenty-five of them at the transcriptional level. Among these, seven have thyroid hormone receptor responsive elements: Adamts2, Lingo3, Mfap3l, Bmp1, Megf10, Nav2, and Crim1. The function of these genes and the significance of their regulation has been discussed (20).
In the rodent cerebellum (279,280) thyroid hormones are involved in the late phase of granular cell migration from the external germinal layer (EGL) to the internal granular layer (IGL). This process takes place postnatally in rodents ending by P20 with the complete disappearance of the EGL. A characteristic feature of the hypothyroid cerebellum is a delay in the migration of granule cells and the persistence of the EGL beyond P20 (Fig. 1) (281). It is unclear how the absence of thyroid hormone interferes with granule cell migration. Cell migration in Thra1 knock-out mice proceeds normally, and there is no effect of hypothyroidism (30). This suggests that the migration defect in hypothyroid mice represents a non-physiological action of the unliganded receptor. On the other hand, these cells express Thra1 during the migration period (P7-P19) and not before (145), which might indicate an action of the receptor on granule cell maturation during migration. Several groups have described T3-regulated genes involved in different processes (9,163,282).
CONCLUSIONS
Thyroid hormone actions in the brain are extremely complex with a continuously changing landscape as development proceeds. Brain maturation involves continuous changes in cell composition, i.e., the target organ of thyroid hormone is under constant change. This is reflected in the changing regulated gene network. Genes that are transcriptional targets of T3 at a certain developmental time may be refractory at another time. The importance of thyroid hormone for the brain requires tightly controlled mechanisms of thyroid hormone delivery to the brain and cellular interactions in the metabolism of thyroid hormones, with crucial roles of DIO2 and DIO3 regulating the cellular concentration of T3.
Disruption of these mechanisms results in syndromes of profound neurological impairment. The challenge is to understand in detail the mechanisms of action of thyroid hormones at different stages of development in the human brain, and not merely extrapolating from rodent models, for a better understanding of thyroid hormone action defects.
REFERENCES
- 1.
- Joffe RT, Sokolov STH. Thyroid hormones, the brain, and affective disorders. Crit Rev Neurobiol. 1994;8:45–63. [PubMed: 8124730]
- 2.
- Laureno R. Neurologic manifestations of thyroid disease. The Endocrinologist. 1996;6:467–473.
- 3.
- Ganguli M, Burmeister LA, Seaberg EC, Belle S, DeKosky ST. Association between dementia and elevated TSH: a community-based study. Biol Psychiatr. 1996;40:714–725. [PubMed: 8894063]
- 4.
- Joffe RT, Pearce EN, Hennessey JV, Ryan JJ, Stern RA. Subclinical hypothyroidism, mood, and cognition in older adults: a review. Int J Geriatr Psychiatry. 2013;28:111–118. [PMC free article: PMC3488161] [PubMed: 22410877]
- 5.
- Craske MG, Stein MB. Anxiety. Lancet. 2016;388:3048–3059. [PubMed: 27349358]
- 6.
- Gil-Ibanez P, Garcia-Garcia F, Dopazo J, Bernal J, Morte B. Global Transcriptome Analysis of Primary Cerebrocortical Cells: Identification of Genes Regulated by Triiodothyronine in Specific Cell Types. Cereb Cortex. 2017;27:706–717. [PubMed: 26534908]
- 7.
- Morte B, Gil-Ibanez P, Bernal J. Regulation of Gene Expression by Thyroid Hormone in Primary Astrocytes: Factors Influencing the Genomic Response. Endocrinology. 2018;159:2083–2092. [PubMed: 29617759]
- 8.
- Laudet V. The origins and evolution of vertebrate metamorphosis. Curr Biol. 2011;21:R726–R737. [PubMed: 21959163]
- 9.
- Chatonnet F, Flamant F, Morte B. A temporary compendium of thyroid hormone target genes in brain. Biochim Biophys Acta. 2015;1849:122–129. [PubMed: 24882357]
- 10.
- Anderson GW, Schoonover CM, Jones SA. Control of thyroid hormone action in the developing rat brain. Thyroid. 2003;13:1039–1056. [PubMed: 14651788]
- 11.
- Bernal J. Thyroid hormones and brain development. Vitam Horm. 2005;71:95–122. [PubMed: 16112266]
- 12.
- Bernal J. Thyroid hormone receptors in brain development and function. Nat Clin Pract Endocrinol Metab. 2007;3:249–259. [PubMed: 17315033]
- 13.
- Koibuchi N, Iwasaki T. Regulation of brain development by thyroid hormone and its modulation by environmental chemicals. Endocr J. 2006;53:295–303. [PubMed: 16702774]
- 14.
- Morreale de Escobar G, Obregon MJ, Escobar del Rey F. Role of thyroid hormone during early brain development. Eur J Endocrinol. 2004;151 Suppl 3:U25–37. [PubMed: 15554884]
- 15.
- Fisher DA, Polk DH. Maturation of thyroid hormone actions. In: Delange F, Fisher DA, Glinoer D, eds. Research in congenital hypothyroidism. New York: Plenum Press; 1988:61-77.
- 16.
- Holt AB, Renfree MB, Chek DB. Comparative aspects of brain growth: a critical evaluation of mammalian species used in brain growth research with emphasis on the Tammar wallaby. In: Hetzel BS, Smith RM, eds. Fetal brain disorders. Recent approaches to the problem of mental deficiency. Amsterdam: Elsevier/North Holland; 1981:17-43.
- 17.
- Morreale de Escobar G, Escobar del Rey F, Ruiz-Marcos A. Thyroid hormone and the developing brain. In: Dussault JH, Walker P, eds. Congenital Hypothyroidism. New York: Marcel Decker, Inc.; 1983:85-126.
- 18.
- Workman AD, Charvet CJ, Clancy B, Darlington RB, Finlay BL. Modeling transformations of neurodevelopmental sequences across mammalian species. J Neurosci. 2013;33:7368–7383. [PMC free article: PMC3928428] [PubMed: 23616543]
- 19.
- Romijn HJ, Hofman MA, Gramsbergen A. At what age is the developing cerebral cortex of the rat comparable to that of the full-term newborn human baby? Early Hum Dev. 1991;26:61–67. [PubMed: 1914989]
- 20.
- Bernal J. Thyroid hormone regulated genes in cerebral cortex development. J Endocrinol. 2017;232:R83–R97. [PubMed: 27852726]
- 21.
- Leto K, Arancillo M, Becker EB, Buffo A, Chiang C, Ding B, Dobyns WB, Dusart I, Haldipur P, Hatten ME, Hoshino M, Joyner AL, Kano M, Kilpatrick DL, Koibuchi N, Marino S, Martinez S, Millen KJ, Millner TO, Miyata T, Parmigiani E, Schilling K, Sekerkova G, Sillitoe RV, Sotelo C, Uesaka N, Wefers A, Wingate RJ, Hawkes R. Consensus Paper: Cerebellar Development. Cerebellum. 2016;15:789–828. [PMC free article: PMC4846577] [PubMed: 26439486]
- 22.
- Chatonnet F, Picou F, Fauquier T, Flamant F. Thyroid hormone action in cerebellum and cerebral cortex development. J Thyroid Res. 2011;2011:145762. [PMC free article: PMC3134109] [PubMed: 21765985]
- 23.
- Berbel P, Navarro D, Roman GC. An evo-devo approach to thyroid hormones in cerebral and cerebellar cortical development: etiological implications for autism. Front Endocrinol (Lausanne). 2014;5:146. [PMC free article: PMC4158880] [PubMed: 25250016]
- 24.
- Legrand J. Effects of thyroid hormones on Central Nervous System. In: Yanai J, ed. Neurobehavioral Teratology. Amsterdam: Elsevier Science Publishers; 1984:331-363.
- 25.
- Eayrs JT. Developmental relationships between brain and thyroid. In: Michael RP, ed. Endocrinol. Hum. Behav. London: Oxford University Press; 1968:239-255.
- 26.
- Madeira MD, Paula-Barbosa M, Cadete-Leite A, Tavares MA. Unbiased estimate of hippocampal granule cell numbers in hypothyroid and in sex-age-matched control rats. J Hirnforsch. 1988;29:643–650. [PubMed: 3235823]
- 27.
- Madeira MD, Cadete-Leite A, Andrade JP, Paula-Barbosa MM. Effects of hypothyroidism upon the granular layer of the dentate gyrus in male and female adult rats: a morphometric study. J Comp Neurol. 1991;314:171–186. [PubMed: 1797872]
- 28.
- Madeira MD, Pereira A, Cadete-Leite A, Paula-Barbosa MM. Estimates of volumes and pyramidal cell numbers in the prelimbic subarea of the prefrontal cortex in experimental hypothyroid rats. J Anat. 1990;171:41–56. [PMC free article: PMC1257126] [PubMed: 2081709]
- 29.
- Berbel P, Navarro D, Auso E, Varea E, Rodriguez AE, Ballesta JJ, Salinas M, Flores E, Faura CC, de Escobar GM. Role of late maternal thyroid hormones in cerebral cortex development: an experimental model for human prematurity. Cereb Cortex. 2010;20:1462–1475. [PMC free article: PMC2871377] [PubMed: 19812240]
- 30.
- Morte B, Manzano J, Scanlan T, Vennstrom B, Bernal J. Deletion of the thyroid hormone receptor alpha 1 prevents the structural alterations of the cerebellum induced by hypothyroidism. Proc Natl Acad Sci U S A. 2002;99:3985–3989. [PMC free article: PMC122635] [PubMed: 11891331]
- 31.
- Alvarez-Dolado M, Ruiz M, del Rio JA, Alcantara S, Burgaya F, Sheldon M, Nakajima K, Bernal J, Howell BW, Curran T, Soriano E, Muñoz A. Thyroid hormone regulates reelin and dab1 expression during brain development. J Neurosci. 1999;19:6979–6973. [PMC free article: PMC6782883] [PubMed: 10436054]
- 32.
- Berbel P, Marco P, Cerezo JR, DeFelipe J. Distribution of parvalbumin immunoreactivity in the neocortex of hypothyroid adult rats. Neurosci Lett. 1996;204:65–68. [PubMed: 8929979]
- 33.
- Gilbert ME, Sui L, Walker MJ, Anderson W, Thomas S, Smoller SN, Schon JP, Phani S, Goodman JH. Thyroid hormone insufficiency during brain development reduces parvalbumin immunoreactivity and inhibitory function in the hippocampus. Endocrinology. 2007;148:92–102. [PubMed: 17008398]
- 34.
- Manzano J, Cuadrado M, Morte B, Bernal J. Influence of thyroid hormone and thyroid hormone receptors in the generation of cerebellar GABAergic interneurons from precursor cells. Endocrinology. 2007 [PubMed: 17761765]
- 35.
- Richard S, Guyot R, Rey-Millet M, Prieux M, Markossian S, Aubert D, Flamant F. A Pivotal Genetic Program Controlled by Thyroid Hormone during the Maturation of GABAergic Neurons. iScience. 2020;23:100899. [PMC free article: PMC7037980] [PubMed: 32092701]
- 36.
- Gould E, Butcher LL. Developing cholinergic basal forebrain neurons are sensitive to thyroid hormone. J Neurosci. 1989;9:3347–3358. [PMC free article: PMC6569663] [PubMed: 2795167]
- 37.
- Dusart I, Flamant F. Profound morphological and functional changes of rodent Purkinje cells between the first and the second postnatal weeks: a metamorphosis? Front Neuroanat. 2012;6:11. [PMC free article: PMC3324107] [PubMed: 22514522]
- 38.
- Legrand J. Analyse de l'action morphogénétic des hormones thyroïdiennes sur le cervelet du jeune rat. Arch Anat Microsc Morphol Exp. 1967;56:205–244. [PubMed: 5622523]
- 39.
- Ruiz-Marcos A, Sanchez-Toscano F, Obregón MJ, Escobar del Rey F, Morreale de Escobar G. Reversible morphological alterations of cortical neurons in juvenile and adult hypothyroidism in the rat. Brain Res. 1980;185:91–102. [PubMed: 7353183]
- 40.
- Ruiz-Marcos A, Sanchez-Toscano F, Obregón MJ, Escobar del Rey F, Morreale de Escobar G. Thyroxine treatment and recovery of hypothyroidism-induced pyramidal cell damage. Brain Res. 1982;239:559–574. [PubMed: 7093702]
- 41.
- Gould E, Allan MD, McEwen BS. Dendritic spine density of adult hippocampal pyramidal cells is sensitive to thyroid hormone. Brain Res. 1990;525:327–329. [PubMed: 2253032]
- 42.
- Ruiz-Marcos A, Cartagena P, García A, Escobar del Rey F, Morreale de Escobar G. Rapid effects of adult-onset hypothyroidism on dendritic spines of pyramidal cells of the rat cerebral cortex. Exp Brain Res. 1988;73:583–588. [PubMed: 3224667]
- 43.
- Balazs R, Brooksbank BWL, Davison AN, Eayrs JT, Wilson DA. The effect of neonatal thyroidectomy on myelination in the rat brain. Brain Res. 1969;15:219–232. [PubMed: 5807766]
- 44.
- Malone MJ, Rosman NP, Szoke M, Davis D. Myelination of brain in experimental hypothyroidism. An electron-microscopic and biochemical study of purified myelin isolates. J Neurol Sci. 1975;26:1–11. [PubMed: 1159453]
- 45.
- Noguchi T, Sugisaki T. Hypomyelination in the cerebrum of the congenitally hypothyroid mouse (hyt). J Neurochem. 1984;42:891–893. [PubMed: 6198475]
- 46.
- Rodriguez-Pena A, Ibarrola N, Iniguez MA, Munoz A, Bernal J. Neonatal hypothyroidism affects the timely expression of myelin- associated glycoprotein in the rat brain. J Clin Invest. 1993;91:812–818. [PMC free article: PMC288032] [PubMed: 7680668]
- 47.
- Adamo AM, Aloise PA, Soto EF, Pasquini JM. Neonatal Hyperthyroidism in the Rat Produces an Increase in the Activity of Microperoxisomal Marker Enzymes Coincident with Biochemical Signs of Accelerated Myelination. J Neurosci Res. 1990;25:353–359. [PubMed: 2325160]
- 48.
- Guadaño-Ferraz A, Escobar del Rey F, Morreale de Escobar G, Innocenti GM, Berbel P. The development of the anterior commissure in normal and hypothyroid rats. Dev Brain Res. 1994;81:293–308. [PubMed: 7813049]
- 49.
- Lopez-Espindola D, Morales-Bastos C, Grijota-Martinez C, Liao XH, Lev D, Sugo E, Verge CF, Refetoff S, Bernal J, Guadano-Ferraz A. Mutations of the thyroid hormone transporter MCT8 cause prenatal brain damage and persistent hypomyelination. J Clin Endocrinol Metab. 2014;99:E2799–2804. [PMC free article: PMC4255116] [PubMed: 25222753]
- 50.
- Morreale de Escobar G, Obregón MJ, Escobar del Rey F. Fetal and maternal thyroid hormones. Horm Res. 1987;26:12–27. [PubMed: 3297961]
- 51.
- Morreale de Escobar G, Pastor R, Obregón MJ, Escobar del Rey F. Effects of maternal hypothyroidism on the weight and thyroid hormone content of rat embryonic tissues. Endocrinology. 1985;117:1890–1901. [PubMed: 4042969]
- 52.
- Nataf B, Sfez M. Debut du fonctionnement de la thyroide foetale du rat. Soc Biol. 1961;156:1235–1238.
- 53.
- Obregón MJ, Mallol J, Pastor R, Morreale de Escobar G, Escobar del Rey G. L-thyroxine and 3,3',5-triiodo-L-thyronine in rat embryos before onset of fetal thyroid function. Endocrinology. 1984;114:305–307. [PubMed: 6690276]
- 54.
- Porterfield SP, Hendrich CE. Tissue iodothyronine levels in fetuses of control and hypothyroid rats at 13 and 16 days gestation. Endocrinology. 1992;131:195–200. [PubMed: 1611997]
- 55.
- Woods RJ, Sinha AK, Ekins RP. Uptake and metabolism of thyroid hormones by the rat foetus early in pregnancy. Clin Sci. 1984;67:359–363. [PubMed: 6467839]
- 56.
- Morreale de Escobar G, Calvo R, Obregón MJ, Escobar del Rey F. Contribution of maternal thyroxine to fetal thyroxine pools in normal rats near term. Endocrinology. 1990;126:2765–2767. [PubMed: 2328706]
- 57.
- Obregón MJ, Ruiz de Oña C, Calvo R, Escobar del Rey F, Morreale de Escobar G. Outer ring iodothyronine deiodinases and thyroid hormone economy: responses to iodine deficiency in the rat fetus and neonate. Endocrinology. 1991;129:2663–2673. [PubMed: 1935795]
- 58.
- Vulsma T, Gons MH, de Vijlder J. Maternal-fetal transfer of thyroxine in congenital hypothyroidism due to a total organification defect or thyroid dysgenesis. N Eng J Med. 1989;321:13–16. [PubMed: 2733742]
- 59.
- van Trotsenburg P, Stoupa A, Leger J, Rohrer T, Peters C, Fugazzola L, Cassio A, Heinrichs C, Beauloye V, Pohlenz J, Rodien P, Coutant R, Szinnai G, Murray P, Bartes B, Luton D, Salerno M, de Sanctis L, Vigone M, Krude H, Persani L, Polak M. Congenital Hypothyroidism: A 2020-2021 Consensus Guidelines Update-An ENDO-European Reference Network Initiative Endorsed by the European Society for Pediatric Endocrinology and the European Society for Endocrinology. Thyroid. 2021;31:387–419. [PMC free article: PMC8001676] [PubMed: 33272083]
- 60.
- de Zegher F, Pernasetti F, Vanhole C, Devlieger H, Van den Berghe G, Martial JA. The prenatal role of thyroid hormone evidenced by fetomaternal Pit-1 deficiency. J Clin Endocrinol Metab. 1995;80:3127–3130. [PubMed: 7593413]
- 61.
- Yasuda T, Ohnishi H, Wataki K, Minagawa M, Minamitami K, Niimi H. Outcome of a baby born from a mother with acquired juvenile hypothyroidism having undetectable thyroid hormone concentrations. J Clin Endocrinol Metabol. 1999;84:2630–2632. [PubMed: 10443651]
- 62.
- Morreale de Escobar G, Pastor R, Obregon MJ, Escobar del Rey F. Effects of maternal hypothyroidism on the weight and thyroid hormone content of rat embryonic tissues, before and after onset of fetal thyroid function. Endocrinology. 1985;117:1890–1900. [PubMed: 4042969]
- 63.
- Morte B, Diez D, Auso E, Belinchon MM, Gil-Ibanez P, Grijota-Martinez C, Navarro D, de Escobar GM, Berbel P, Bernal J. Thyroid hormone regulation of gene expression in the developing rat fetal cerebral cortex: prominent role of the Ca2+/calmodulin-dependent protein kinase IV pathway. Endocrinology. 2010;151:810–820. [PubMed: 20056827]
- 64.
- Ausó E, Lavado-Autric R, Cuevas E, Del Rey FE, Morreale De Escobar G, Berbel P. A moderate and transient deficiency of maternal thyroid function at the beginning of fetal neocorticogenesis alters neuronal migration. Endocrinology. 2004;145:4037–4047. [PubMed: 15087434]
- 65.
- Goodman JH, Gilbert ME. Modest thyroid hormone insufficiency during development induces a cellular malformation in the corpus callosum: a model of cortical dysplasia. Endocrinology. 2007;148:2593–2597. [PubMed: 17317780]
- 66.
- Morte B, Gil-Ibanez P, Heuer H, Bernal J. Brain Gene Expression in Systemic Hypothyroidism and Mouse Models of MCT8 Deficiency: The Mct8-Oatp1c1-Dio2 Triad. Thyroid. 2021;31:985–993. [PubMed: 33307956]
- 67.
- Shepard TH. Onset of function of the human fetal thyroid: biochemical and autoradiographic studies from organ culture. J Clin Endocrinol Metabol. 1967;27:945. [PubMed: 5339119]
- 68.
- Olin P, Vecchio G, Ekholm R, Almqvist S. Human fetal thyroglobulin: characterization and in vitro biosynthesis studies. Endocrinology. 1970;86:1041–1048. [PubMed: 5435246]
- 69.
- Szinnai G, Lacroix L, Carre A, Guimiot F, Talbot M, Martinovic J, Delezoide AL, Vekemans M, Michiels S, Caillou B, Schlumberger M, Bidart JM, Polak M. Sodium/iodide symporter (NIS) gene expression is the limiting step for the onset of thyroid function in the human fetus. J Clin Endocrinol Metab. 2007;92:70–76. [PubMed: 17077129]
- 70.
- Greenberg AH, Czernichow P, Reba RC, Tyson J, Blizzard RM. Observations on the maturation of thyroid function in early fetal life. J Clin Invest. 1970;49:1790–1803. [PMC free article: PMC322668] [PubMed: 4989616]
- 71.
- Thorpe-Beeston JG, Nicolaides KH, Felton CV, Butler J, McGregor AM. Maturation of the secretion of thyroid hormone and thyroid stimulating hormone in the fetus. N Eng J Med. 1991;324:532–536. [PubMed: 1899469]
- 72.
- Thorpe-Beeston JG, Nicolaides KH. Fetal thyroid function. Fetal Diagn Ther. 1993;8:60–72. [PubMed: 8452651]
- 73.
- Morreale de Escobar G, Ares S. The hypothyroxinemia of prematurity. J Clin Endocrinol Metabol. 1998;83:713–715. [PubMed: 9467601]
- 74.
- Contempré B, Jauniaux E, Calvo R, Jurkovic D, Campbell S, Morreale de Escobar G. Detection of thyroid hormones in human embryonic cavities during the first trimester of pregnancy. J Clin Endocrinol Metabol. 1993;77:1719–1722. [PubMed: 8263162]
- 75.
- Calvo RM, Jauniaux E, Gulbis B, Asuncion M, Gervy C, Contempre B, Morreale de Escobar G. Fetal tissues are exposed to biologically relevant free thyroxine concentrations during early phases of development. J Clin Endocrinol Metab. 2002;87:1768–1777. [PubMed: 11932315]
- 76.
- Kester MH, Martinez de Mena R, Obregon MJ, Marinkovic D, Howatson A, Visser TJ, Hume R, Morreale de Escobar G. Iodothyronine levels in the human developing brain: major regulatory roles of iodothyronine deiodinases in different areas. J Clin Endocrinol Metab. 2004;89:3117–3128. [PubMed: 15240580]
- 77.
- Bernal J, Pekonen F. Ontogenesis of the nuclear 3,5,3'-triiodothyronine receptor in the human fetal brain. Endocrinology. 1984;114:677–679. [PubMed: 6317365]
- 78.
- Morreale de Escobar G, Obregón MJ, Escobar del Rey F. Is neuropsychological development related to maternal hypothyroidism, or to maternal hypothyroxinemia? J Clin Endocrinol Metabol. 2000;85:3975–3987. [PubMed: 11095417]
- 79.
- de Escobar GM, Obregon MJ, del Rey FE. Maternal thyroid hormones early in pregnancy and fetal brain development. Best Pract Res Clin Endocrinol Metab. 2004;18:225–248. [PubMed: 15157838]
- 80.
- Berbel P, Mestre JL, Santamaria A, Palazon I, Franco A, Graells M, Gonzalez-Torga A, de Escobar GM. Delayed neurobehavioral development in children born to pregnant women with mild hypothyroxinemia during the first month of gestation: the importance of early iodine supplementation. Thyroid. 2009;19:511–519. [PubMed: 19348584]
- 81.
- Henrichs J, Bongers-Schokking JJ, Schenk JJ, Ghassabian A, Schmidt HG, Visser TJ, Hooijkaas H, de Muinck Keizer-Schrama SM, Hofman A, Jaddoe VV, Visser W, Steegers EA, Verhulst FC, de Rijke YB, Tiemeier H. Maternal thyroid function during early pregnancy and cognitive functioning in early childhood: the generation R study. J Clin Endocrinol Metab. 2010;95:4227–4234. [PubMed: 20534757]
- 82.
- Ghassabian A, El Marroun H, Peeters RP, Jaddoe VW, Hofman A, Verhulst FC, Tiemeier H, White T. Downstream effects of maternal hypothyroxinemia in early pregnancy: nonverbal IQ and brain morphology in school-age children. J Clin Endocrinol Metab. 2014;99:2383–2390. [PubMed: 24684462]
- 83.
- Momotani N, Iwama S, Momotani K. Neurodevelopment in children born to hypothyroid mothers restored to normal thyroxine (T(4)) concentration by late pregnancy in Japan: no apparent influence of maternal T(4) deficiency. J Clin Endocrinol Metab. 2011;97:1104–1108. [PubMed: 22319040]
- 84.
- Glinoer D. The regulation of thyroid function in pregnancy: pathways of endocrine adaptation from physiology to pathology. Endocr Rev. 1997;18:401–433. [PubMed: 9183570]
- 85.
- Henrichs J, Ghassabian A, Peeters RP, Tiemeier H. Maternal hypothyroxinemia and effects on cognitive functioning in childhood: how and why? Clin Endocrinol (Oxf). 2013;79:152–162. [PubMed: 23600900]
- 86.
- Ramezani Tehrani F, Nazarpour S, Behboudi-Gandevani S. Isolated maternal hypothyroxinemia and adverse pregnancy outcomes: A systematic review. J Gynecol Obstet Hum Reprod. 2021;50:102057. [PubMed: 33401029]
- 87.
- Thompson W, Russell G, Baragwanath G, Matthews J, Vaidya B, Thompson-Coon J. Maternal thyroid hormone insufficiency during pregnancy and risk of neurodevelopmental disorders in offspring: A systematic review and meta-analysis. Clin Endocrinol (Oxf). 2018;88:575–584. [PMC free article: PMC5888183] [PubMed: 29325223]
- 88.
- Dosiou C, Medici M. MANAGEMENT OF ENDOCRINE DISEASE: Isolated maternal hypothyroxinemia during pregnancy: knowns and unknowns. Eur J Endocrinol. 2017;176:R21–R38. [PubMed: 27528503]
- 89.
- Casey BM, Thom EA, Peaceman AM, Varner MW, Sorokin Y, Hirtz DG, Reddy UM, Wapner RJ, Thorp JM Jr, Saade G, Tita AT, Rouse DJ, Sibai B, Iams JD, Mercer BM, Tolosa J, Caritis SN, VanDorsten JP. Eunice Kennedy Shriver National Institute of Child H, Human Development Maternal-Fetal Medicine Units N. Treatment of Subclinical Hypothyroidism or Hypothyroxinemia in Pregnancy. N Engl J Med. 2017;376:815–825. [PMC free article: PMC5605129] [PubMed: 28249134]
- 90.
- Lazarus JH, Bestwick JP, Channon S, Paradice R, Maina A, Rees R, Chiusano E, John R, Guaraldo V, George LM, Perona M, Dall'Amico D, Parkes AB, Joomun M, Wald NJ. Antenatal thyroid screening and childhood cognitive function. N Engl J Med. 2012;366:493–501. [PMC free article: PMC3814060] [PubMed: 22316443]
- 91.
- Cooper DS, Pearce EN. Subclinical Hypothyroidism and Hypothyroxinemia in Pregnancy - Still No Answers. N Engl J Med. 2017;376:876–877. [PubMed: 28249140]
- 92.
- Visser WE, Friesema EC, Visser TJ. Minireview: thyroid hormone transporters: the knowns and the unknowns. Mol Endocrinol. 2011;25:1–14. [PMC free article: PMC5417302] [PubMed: 20660303]
- 93.
- Roberts LM, Woodford K, Zhou M, Black DS, Haggerty JE, Tate EH, Grindstaff KK, Mengesha W, Raman C, Zerangue N. Expression of the thyroid hormone transporters monocarboxylate transporter-8 (SLC16A2) and organic ion transporter-14 (SLCO1C1) at the blood-brain barrier. Endocrinology. 2008;149:6251–6261. [PubMed: 18687783]
- 94.
- Ceballos A, Belinchon MM, Sanchez-Mendoza E, Grijota-Martinez C, Dumitrescu AM, Refetoff S, Morte B, Bernal J. Importance of Monocarboxylate transporter 8 (Mct8) for the blood-brain barrier dependent availability of 3,5,3’-Triiodo-L-Thyronine (T3). Endocrinology. 2009. In Press. [PMC free article: PMC2671898] [PubMed: 19147674]
- 95.
- Bernal J, Guadano-Ferraz A, Morte B. Thyroid hormone transporters-functions and clinical implications. Nat Rev Endocrinol. 2015;11:406–417. [PubMed: 25942657]
- 96.
- Lopez-Espindola D, Garcia-Aldea A, Gomez de la Riva I, Rodriguez-Garcia AM, Salvatore D, Visser TJ, Bernal J, Guadano-Ferraz A. Thyroid hormone availability in the human fetal brain: novel entry pathways and role of radial glia. Brain Struct Funct. 2019;224:2103–2119. [PubMed: 31165302]
- 97.
- Vatine GD, Al-Ahmad A, Barriga BK, Svendsen S, Salim A, Garcia L, Garcia VJ, Ho R, Yucer N, Qian T, Lim RG, Wu J, Thompson LM, Spivia WR, Chen Z, Van Eyk J, Palecek SP, Refetoff S, Shusta EV, Svendsen CN. Modeling Psychomotor Retardation using iPSCs from MCT8-Deficient Patients Indicates a Prominent Role for the Blood-Brain Barrier. Cell Stem Cell. 2017;20:831–843 e835. [PMC free article: PMC6659720] [PubMed: 28526555]
- 98.
- Abbott NJ, Ronnback L, Hansson E. Astrocyte-endothelial interactions at the blood-brain barrier. Nat Rev Neurosci. 2006;7:41–53. [PubMed: 16371949]
- 99.
- Abbott NJ, Patabendige AA, Dolman DE, Yusof SR, Begley DJ. Structure and function of the blood-brain barrier. Neurobiol Dis. 2010;37:13–25. [PubMed: 19664713]
- 100.
- Pardridge WM. Brain metabolism: a perspective from the blood-brain barrier. Physiol Rev. 1983;63:1481–1535. [PubMed: 6361813]
- 101.
- Ben-Zvi A, Lacoste B, Kur E, Andreone BJ, Mayshar Y, Yan H, Gu C. Mfsd2a is critical for the formation and function of the blood-brain barrier. Nature. 2014;509:507–511. [PMC free article: PMC4134871] [PubMed: 24828040]
- 102.
- Haddad-Tovolli R, Dragano NRV, Ramalho AFS, Velloso LA. Development and Function of the Blood-Brain Barrier in the Context of Metabolic Control. Front Neurosci. 2017;11:224. [PMC free article: PMC5399017] [PubMed: 28484368]
- 103.
- Rinholm JE, Bergersen LH. Neuroscience: The wrap that feeds neurons. Nature. 2012;487:435–436. [PubMed: 22836992]
- 104.
- Zhao Z, Nelson AR, Betsholtz C, Zlokovic BV. Establishment and Dysfunction of the Blood-Brain Barrier. Cell. 2015;163:1064–1078. [PMC free article: PMC4655822] [PubMed: 26590417]
- 105.
- Ito K, Uchida Y, Ohtsuki S, Aizawa S, Kawakami H, Katsukura Y, Kamiie J, Terasaki T. Quantitative membrane protein expression at the blood-brain barrier of adult and younger cynomolgus monkeys. J Pharm Sci. 2011;100:3939–3950. [PubMed: 21254069]
- 106.
- Wirth EK, Roth S, Blechschmidt C, Holter SM, Becker L, Racz I, Zimmer A, Klopstock T, Gailus-Durner V, Fuchs H, Wurst W, Naumann T, Brauer A, de Angelis MH, Kohrle J, Gruters A, Schweizer U. Neuronal 3',3,5-triiodothyronine (T3) uptake and behavioral phenotype of mice deficient in Mct8, the neuronal T3 transporter mutated in Allan-Herndon-Dudley syndrome. J Neurosci. 2009;29:9439–9449. [PMC free article: PMC6666526] [PubMed: 19641107]
- 107.
- Morte B, Ceballos A, Diez D, Grijota-Martinez C, Dumitrescu AM, Di Cosmo C, Galton VA, Refetoff S, Bernal J. Thyroid hormone-regulated mouse cerebral cortex genes are differentially dependent on the source of the hormone: a study in monocarboxylate transporter-8- and deiodinase-2-deficient mice. Endocrinology. 2010;151:2381–2387. [PMC free article: PMC2869252] [PubMed: 20211971]
- 108.
- Dumitrescu AM, Liao XH, Weiss RE, Millen K, Refetoff S. Tissue-specific thyroid hormone deprivation and excess in monocarboxylate transporter (mct) 8-deficient mice. Endocrinology. 2006;147:4036–4043. [PubMed: 16709608]
- 109.
- Trajkovic M, Visser TJ, Mittag J, Horn S, Lukas J, Darras VM, Raivich G, Bauer K, Heuer H. Abnormal thyroid hormone metabolism in mice lacking the monocarboxylate transporter 8. J Clin Invest. 2007;117:627–635. [PMC free article: PMC1797602] [PubMed: 17318265]
- 110.
- Liao XH, Di Cosmo C, Dumitrescu AM, Hernandez A, Van Sande J, St Germain DL, Weiss RE, Galton VA, Refetoff S. Distinct roles of deiodinases on the phenotype of Mct8 defect: a comparison of eight different mouse genotypes. Endocrinology. 2011;152:1180–1191. [PMC free article: PMC3040057] [PubMed: 21285310]
- 111.
- Mayerl S, Muller J, Bauer R, Richert S, Kassmann CM, Darras VM, Buder K, Boelen A, Visser TJ, Heuer H. Transporters MCT8 and OATP1C1 maintain murine brain thyroid hormone homeostasis. J Clin Invest. 2014;124:1987–1999. [PMC free article: PMC4001533] [PubMed: 24691440]
- 112.
- Gereben B, Zavacki AM, Ribich S, Kim BW, Huang SA, Simonides WS, Zeold A, Bianco AC. Cellular and molecular basis of deiodinase-regulated thyroid hormone signaling. Endocr Rev. 2008;29:898–938. [PMC free article: PMC2647704] [PubMed: 18815314]
- 113.
- Barez-Lopez S, Bosch-Garcia D, Gomez-Andres D, Pulido-Valdeolivas I, Montero-Pedrazuela A, Obregon MJ, Guadano-Ferraz A. Abnormal motor phenotype at adult stages in mice lacking type 2 deiodinase. PLoS One. 2014;9:e103857. [PMC free article: PMC4118963] [PubMed: 25083788]
- 114.
- Guadaño-Ferraz A, Obregón MJ, St-Germain D, Bernal J. The type 2 iodothyronine deiodinase is expressed primarily in glial cells in the neonatal rat brain. Proc Natl Acad Sci USA. 1997;94:10391–10396. [PMC free article: PMC23373] [PubMed: 9294221]
- 115.
- Guadaño-Ferraz A, Escámez MJ, Rausell E, Bernal J. Expression of type 2 iodothyronine deiodinase in hypothyroid rat brain indicates an important role of thyroid hormone in the development of specific primary sensory systems. J Neurosci. 1999;19:3430–3439. [PMC free article: PMC6782260] [PubMed: 10212303]
- 116.
- Tu HM, Kim SW, Salvatore D, Bartha T, Legradi G, Larsen PR, Lechan RM. Regional distribution of type 2 thyroxine deiodinase messenger ribonucleic acid in rat hypothalamus and pituitary and its regulation by thyroid hormone. Endocrinology. 1997;138:3359–3368. [PubMed: 9231788]
- 117.
- Tu HM, Legradi G, Bartha T, Salvatore D, Lechan RM, Larsen PR. Regional expression of the type 3 iodothyronine deiodinase messenger ribonucleic acid in the rat central nervous system and its regulation by thyroid hormone. Endocrinology. 1999;140:784–790. [PubMed: 9927306]
- 118.
- Diez D, Morte B, Bernal J. Single-cell transcriptome profiling of thyroid hormone effectors in the human fetal neocortex: expression of SLCO1C1, DIO2, and THRB in specific cell types. Thyroid. 2021 [PubMed: 34114484]
- 119.
- Escámez MJ, Guadaño-Ferraz A, Cuadrado A, Bernal J. Type 3 iodothyronine deiodinase is selectively expressed in areas related to sexual differentiation in the newborn rat brain. Endocrinology. 1999;140:5443–5446. [PubMed: 10537178]
- 120.
- Freitas BC, Gereben B, Castillo M, Kallo I, Zeold A, Egri P, Liposits Z, Zavacki AM, Maciel RM, Jo S, Singru P, Sanchez E, Lechan RM, Bianco AC. Paracrine signaling by glial cell-derived triiodothyronine activates neuronal gene expression in the rodent brain and human cells. J Clin Invest. 2010;120:2206–2217. [PMC free article: PMC2877954] [PubMed: 20458138]
- 121.
- Riskind PN, Kolodny JM, Larsen PR. The regional distribution of type II 5'-monodeiodinase in euthyroid and hypothyroid rats. Brain Res. 1987;420:194–198. [PubMed: 3676753]
- 122.
- Langlet F, Levin BE, Luquet S, Mazzone M, Messina A, Dunn-Meynell AA, Balland E, Lacombe A, Mazur D, Carmeliet P, Bouret SG, Prevot V, Dehouck B. Tanycytic VEGF-A boosts blood-hypothalamus barrier plasticity and access of metabolic signals to the arcuate nucleus in response to fasting. Cell Metab. 2013;17:607–617. [PMC free article: PMC3695242] [PubMed: 23562080]
- 123.
- Bolborea M, Dale N. Hypothalamic tanycytes: potential roles in the control of feeding and energy balance. Trends Neurosci. 2013;36:91–100. [PMC free article: PMC3605593] [PubMed: 23332797]
- 124.
- Larsen PR, Silva JR, Kaplan MM. Relationships between circulating and intracellular thyroid hormones. Physiological and clinical implications. Endocr Rev. 1981;2:87–102. [PubMed: 6271545]
- 125.
- van Doorn J, Roelfsema F, van der Heide D. Contribution from local conversion of thyroxine to 3,5,3'-triiodothyronine to intracellular 3,5,3'-triiodothyronine in several organs in hypothyroid rats at isotope equilibrium. Acta Endocrinol (Copenh). 1982;101:386–396. [PubMed: 7148336]
- 126.
- Galton VA, Wood ET, St Germain EA, Withrow CA, Aldrich G, St Germain GM, Clark AS, St Germain DL. Thyroid hormone homeostasis and action in the type 2 deiodinase-deficient rodent brain during development. Endocrinology. 2007;148:3080–3088. [PubMed: 17332058]
- 127.
- Morte B, Bernal J. Thyroid hormone action: astrocyte-neuron communication. Front Endocrinol (Lausanne). 2014;5:82. [PMC free article: PMC4038973] [PubMed: 24910631]
- 128.
- Crantz FR, Silva JE, Larsen PR. An analysis of the sources and quantity of 3,5,3'-triiodothyronine specifically bound to nuclear receptors in rat cerebral cortex and cerebellum. Endocrinology. 1982;110:367–375. [PubMed: 6276132]
- 129.
- Ng L, Hernandez A, He W, Ren T, Srinivas M, Ma M, Galton VA, St Germain DL, Forrest D. A protective role for type 3 deiodinase, a thyroid hormone-inactivating enzyme, in cochlear development and auditory function. Endocrinology. 2009;150:1952–1960. [PMC free article: PMC2659284] [PubMed: 19095741]
- 130.
- Campos-Barros A, Amma LL, Faris JS, Shailam R, Kelley MW, Forrest D. Type 2 iodothyronine deiodinase expression in the cochlea before the onset of hearing. Proc Natl Acad Sci USA. 2000;97:1287–1292. [PMC free article: PMC15599] [PubMed: 10655523]
- 131.
- Eldred KC, Hadyniak SE, Hussey KA, Brenerman B, Zhang PW, Chamling X, Sluch VM, Welsbie DS, Hattar S, Taylor J, Wahlin K, Zack DJ, Johnston RJ Jr. Thyroid hormone signaling specifies cone subtypes in human retinal organoids. Science. 2018:362. [PMC free article: PMC6249681] [PubMed: 30309916]
- 132.
- Galton VA, Martínez E, Hernández A, St. Germain EA, Bates JM, St.Germain DL. Pregnant rat uterus expresses high levels of the type 3 iodothyronine deiodinase. J Clin Invest. 1999;103:979–987. [PMC free article: PMC408265] [PubMed: 10194470]
- 133.
- Bates JM, St.Germain DL, Galton VA. Expression profiles of the three iodothyronine deiodinases D1, D2 and D3, in the developing rat. Endocrinology. 1999;140:844–851. [PubMed: 9927314]
- 134.
- Ruiz de Oña C, Obregón MJ, Escobar del Rey F, Morreale de Escobar G. Developmental changes in rat brain 5'-deiodinase and thyroid hormones during the fetal period: the effects of fetal hypothyroidism and maternal thyroid hormones. Pediatr Res. 1988;24:588–594. [PubMed: 3205610]
- 135.
- Zuchero JB, Barres BA. Glia in mammalian development and disease. Development. 2015;142:3805–3809. [PMC free article: PMC4712885] [PubMed: 26577203]
- 136.
- Calvo R, Obregón MJ, Ruiz de Oña C, Escobar del Rey F, Morreale de Escobar G. Congenital hypothyroidism, as studied in rats. Crucial role of maternal thyroxine but not of 3,5,3'-triiodothyronine in the protection of the fetal brain. J Clin Invest. 1990;86:889–899. [PMC free article: PMC296808] [PubMed: 2394838]
- 137.
- Barez-Lopez S, Obregon MJ, Bernal J, Guadano-Ferraz A. Thyroid Hormone Economy in the Perinatal Mouse Brain: Implications for Cerebral Cortex Development. Cereb Cortex. 2018;28:1783–1793. [PubMed: 28407057]
- 138.
- Grijota-Martinez C, Diez D, Morreale de Escobar G, Bernal J, Morte B. Lack of action of exogenously administered T3 on the fetal rat brain despite expression of the monocarboxylate transporter 8. Endocrinology. 2011;152:1713–1721. [PubMed: 21303950]
- 139.
- Hernandez A, Morte B, Belinchon MM, Ceballos A, Bernal J. Critical role of types 2 and 3 deiodinases in the negative regulation of gene expression by T(3)in the mouse cerebral cortex. Endocrinology. 2012;153:2919–2928. [PMC free article: PMC3359606] [PubMed: 22523155]
- 140.
- Hernandez A, Quignodon L, Martinez ME, Flamant F, St Germain DL. Type 3 deiodinase deficiency causes spatial and temporal alterations in brain T3 signaling that are dissociated from serum thyroid hormone levels. Endocrinology. 2010;151:5550–5558. [PMC free article: PMC2954712] [PubMed: 20719855]
- 141.
- Martinez ME, Hernandez A. The Type 3 Deiodinase Is a Critical Modulator of Thyroid Hormone Sensitivity in the Fetal Brain. Front Neurosci. 2021;15:703730. [PMC free article: PMC8265566] [PubMed: 34248495]
- 142.
- Chan S, Kachilele S, McCabe CJ, Tannahill LA, Boelaert K, Gittoes NJ, Visser TJ, Franklyn JA, Kilby MD. Early expression of thyroid hormone deiodinases and receptors in human fetal cerebral cortex. Brain Res Dev Brain Res. 2002;138:109–116. [PubMed: 12354639]
- 143.
- Zhong S, Zhang S, Fan X, Wu Q, Yan L, Dong J, Zhang H, Li L, Sun L, Pan N, Xu X, Tang F, Zhang J, Qiao J, Wang X. A single-cell RNA-seq survey of the developmental landscape of the human prefrontal cortex. Nature. 2018;555:524–528. [PubMed: 29539641]
- 144.
- Johnson MB, Wang PP, Atabay KD, Murphy EA, Doan RN, Hecht JL, Walsh CA. Single-cell analysis reveals transcriptional heterogeneity of neural progenitors in human cortex. Nat Neurosci. 2015;18:637–646. [PMC free article: PMC5568903] [PubMed: 25734491]
- 145.
- Wallis K, Dudazy S, van Hogerlinden M, Nordstrom K, Mittag J, Vennstrom B. The thyroid hormone receptor alpha1 protein is expressed in embryonic postmitotic neurons and persists in most adult neurons. Mol Endocrinol. 2010;24:1904–1916. [PMC free article: PMC5417394] [PubMed: 20739404]
- 146.
- Saunders NR, Dziegielewska KM, Mollgard K, Habgood MD. Physiology and molecular biology of barrier mechanisms in the fetal and neonatal brain. J Physiol. 2018;596:5723–5756. [PMC free article: PMC6265560] [PubMed: 29774535]
- 147.
- Polioudakis D, de la Torre-Ubieta L, Langerman J, Elkins AG, Shi X, Stein JL, Vuong CK, Nichterwitz S, Gevorgian M, Opland CK, Lu D, Connell W, Ruzzo EK, Lowe JK, Hadzic T, Hinz FI, Sabri S, Lowry WE, Gerstein MB, Plath K, Geschwind DH. A Single-Cell Transcriptomic Atlas of Human Neocortical Development during Mid-gestation. Neuron. 2019;103:785–801 e788. [PMC free article: PMC6831089] [PubMed: 31303374]
- 148.
- Flamant F, Gauthier K, Richard S. Genetic Investigation of Thyroid Hormone Receptor Function in the Developing and Adult Brain. Curr Top Dev Biol. 2017;125:303–335. [PubMed: 28527576]
- 149.
- Flamant F, Gauthier K. Thyroid hormone receptors: the challenge of elucidating isotype-specific functions and cell-specific response. Biochim Biophys Acta. 2013;1830:3900–3907. [PubMed: 22704954]
- 150.
- Ercan-Fang S, Schwartz HL, Oppenheimer JH. Isoform specific 3,5,3'-triiodothyronine receptor binding capacity and messenger ribonucleic acid content in rat adenohypophysis: Effect of thyroidal state and comparison with extrapituitary tissues. Endocrinology. 1996;137:3228–3233. [PubMed: 8754744]
- 151.
- Minakhina S, Bansal S, Zhang A, Brotherton M, Janodia R, De Oliveira V, Tadepalli S, Wondisford FE. A Direct Comparison of Thyroid Hormone Receptor Protein Levels in Mice Provides Unexpected Insights into Thyroid Hormone Action. Thyroid. 2020;30:1193–1204. [PMC free article: PMC7415890] [PubMed: 32122258]
- 152.
- Messier N, Langlois MF. Triac regulation of transcription is T(3) receptor isoform- and response element-specific. Mol Cell Endocrinol. 2000;165:57–66. [PubMed: 10940484]
- 153.
- Bradley DJ, Towle HC, Young WS. Spatial and temporal expression of a- and b- thyroid hormone receptor mRNAs, including the b2-subtype, in the developing mammalian nervous system. J Neurosci. 1992;12:2288–2302. [PMC free article: PMC6575910] [PubMed: 1607941]
- 154.
- Bradley DJ, Young WS III, Weinberger C. Differential expression of alpha and beta thyroid hormone receptor genes in rat brain and pituitary. Proc Natl Acad Sci USA. 1989;86:72507254. [PMC free article: PMC298035] [PubMed: 2780568]
- 155.
- Mellström B, Naranjo JR, Santos A, González AM, Bernal J. Independent expression of the a and b c-erbA genes in developing rat brain. Mol Endocrinol. 1991;5:1339–1350. [PubMed: 1663215]
- 156.
- Luo M, Faure R, Dussault JH. Ontogenesis of nuclear T3 receptors in primary cultured astrocytes and neurons. Brain Res. 1986;381:275–280. [PubMed: 3756504]
- 157.
- Yusta B, Besnard F, Ortiz-Caro J, Pascual A, Aranda A, Sarlieve L. Evidence for the presence of nuclear 3,5,3'-triiodothyronine receptors in secondary cultures of pure rat oligodendrocytes. Endocrinology. 1988;122:2278–2284. [PubMed: 3359982]
- 158.
- Glauser L, Barakat-Walter I. Differential distribution of thyroid hormone receptor isoform in rat dorsal root ganglia and sciatic nerve in vivo and in vitro. J Neuroendocrinol. 1997;9:217–227. [PubMed: 9089473]
- 159.
- Barakat-Walter I, Droz B. Nuclear and cytoplasmic triiodothyronine binding sites in primary sensory neurons and Schwann cells: radioautographic study during development. J Neuroendocrinol. 1995;7:127–136. [PubMed: 7767325]
- 160.
- Lima FR, Gervais A, Colin C, Izembart M, Neto VM, Mallat M. Regulation of microglial development: a novel role for thyroid hormone. J Neurosci. 2001;21:2028–2038. [PMC free article: PMC6762591] [PubMed: 11245686]
- 161.
- Barca-Mayo O, Liao XH, Alonso M, Di Cosmo C, Hernandez A, Refetoff S, Weiss RE. Thyroid hormone receptor alpha and regulation of type 3 deiodinase. Mol Endocrinol. 2011;25:575–583. [PMC free article: PMC3063087] [PubMed: 21292823]
- 162.
- Chatonnet F, Guyot R, Benoit G, Flamant F. Genome-wide analysis of thyroid hormone receptors shared and specific functions in neural cells. Proc Natl Acad Sci U S A. 2013;110:E766–775. [PMC free article: PMC3581916] [PubMed: 23382204]
- 163.
- Gil-Ibanez P, Bernal J, Morte B. Thyroid hormone regulation of gene expression in primary cerebrocortical cells: role of thyroid hormone receptor subtypes and interactions with retinoic acid and glucocorticoids. PLoS One. 2014;9:e91692. [PMC free article: PMC3950245] [PubMed: 24618783]
- 164.
- Gil-Ibanez P, Morte B, Bernal J. Role of thyroid hormone receptor subtypes alpha and beta on gene expression in the cerebral cortex and striatum of postnatal mice. Endocrinology. 2013;154:1940–1947. [PubMed: 23493375]
- 165.
- Ng L, Hurley JB, Dierks B, Srinivas M, Salto C, Vennstrom B, Reh TA, Forrest D. A thyroid hormone receptor that is required for the development of green cone photoreceptors. Nat Genet. 2001;27:94–98. [PubMed: 11138006]
- 166.
- Jones I, Srinivas M, Ng L, Forrest D. The thyroid hormone receptor beta gene: structure and functions in the brain and sensory systems. Thyroid. 2003;13:1057–1068. [PubMed: 14651789]
- 167.
- Cook CB, Kakucska I, Lechan RM, Koenig RJ. Expression of thyroid hormone receptor beta 2 in rat hypothalamus. Endocrinology. 1992;130:1077–1079. [PubMed: 1733708]
- 168.
- Pérez-Castillo A, Bernal J, Ferreiro B, Pans T. The early ontogenesis of thyroid hormone receptor in the rat fetus. Endocrinology. 1985;117:2457–2461. [PubMed: 2998737]
- 169.
- Schwartz HL, Oppenheimer JH. Ontogenesis of 3,5,3'-triiodothyronine receptors in neonatal rat brain, dissociation between receptor concentration and stimulation of oxygen consumption by 3,5,3'-triiodothyronine. Endocrinology. 1978;103:943–948. [PubMed: 217661]
- 170.
- Strait KA, Schwartz HL, Perezcastillo A, Oppenheimer JH. Relationship of C-erbA Messenger RNA Content to Tissue Triiodothyronine Nuclear Binding Capacity and Function in Developing and Adult Rats. J BiolChem. 1990;265:10514–10521. [PubMed: 2162351]
- 171.
- Ferreiro B, Pastor R, Bernal J. T3 receptor occupancy and T3 levels in plasma and cytosol during rat brain development. Acta Endocrinol (Copenh). 1990;123:95–99. [PubMed: 2389629]
- 172.
- Iskaros J, Pickard M, Evans I, Sinha A, Hardiman P, Ekins R. Thyroid hormone receptor gene expression in first trimester human fetal brain. J Clin Endocrinol Metabol. 2000;85:2620–2623. [PubMed: 10902817]
- 173.
- Ferreiro B, Bernal J, Goodyer CG, Branchard CL. Estimation of nuclear thyroid hormone receptor saturation in human fetal brain and lung during early gestation. J Clin Endocrinol Metabol. 1988;67:853–856. [PubMed: 3417852]
- 174.
- Ferreiro B, Bernal J, Morreale de Escobar G, Potter BJ. Preferential saturation of brain 3,5,3'-triiodothyronine receptor during development in fetal lambs. Endocrinology. 1988;122:438–443. [PubMed: 3338407]
- 175.
- Iñiguez MA, De Lecea L, Guadaño-Ferraz A, Morte B, Gerendasy D, Sutcliffe JG, Bernal J. Cell-specific effects of thyroid hormone on RC3/neurogranin expression in rat brain. Endocrinology. 1996;137:1032–1041. [PubMed: 8603571]
- 176.
- Chatonnet F, Guyot R, Picou F, Bondesson M, Flamant F. Genome-wide search reveals the existence of a limited number of thyroid hormone receptor alpha target genes in cerebellar neurons. PLoS One. 2012;7:e30703. [PMC free article: PMC3346809] [PubMed: 22586439]
- 177.
- Diez D, Grijota-Martinez C, Agretti P, De Marco G, Tonacchera M, Pinchera A, de Escobar GM, Bernal J, Morte B. Thyroid hormone action in the adult brain: gene expression profiling of the effects of single and multiple doses of triiodo-L-thyronine in the rat striatum. Endocrinology. 2008;149:3989–4000. [PubMed: 18467437]
- 178.
- Dong H, Wade M, Williams A, Lee A, Douglas GR, Yauk C. Molecular insight into the effects of hypothyroidism on the developing cerebellum. Biochem Biophys Res Commun. 2005;330:1182–1193. [PubMed: 15823568]
- 179.
- Martel J, Cayrou C, Puymirat J. Identification of new thyroid hormone-regulated genes in rat brain neuronal cultures. Neuroreport. 2002;13:1849–1851. [PubMed: 12395077]
- 180.
- Miller LD, McPhie P, Suzuki H, Kato Y, Liu ET, Cheng SY. Multi-tissue gene-expression analysis in a mouse model of thyroid hormone resistance. Genome Biol. 2004;5:R31. [PMC free article: PMC416467] [PubMed: 15128445]
- 181.
- Poguet AL, Legrand C, Feng X, Yen PM, Meltzer P, Samarut J, Flamant F. Microarray analysis of knockout mice identifies cyclin D2 as a possible mediator for the action of thyroid hormone during the postnatal development of the cerebellum. Dev Biol. 2003;254:188–199. [PubMed: 12591240]
- 182.
- Quignodon L, Grijota-Martinez C, Compe E, Guyot R, Allioli N, Laperriere D, Walker R, Meltzer P, Mader S, Samarut J, Flamant F. A combined approach identifies a limited number of new thyroid hormone target genes in post-natal mouse cerebellum. J Mol Endocrinol. 2007;39:17–28. [PubMed: 17601882]
- 183.
- Sato Y, Buchholz DR, Paul BD, Shi YB. A role of unliganded thyroid hormone receptor in postembryonic development in Xenopus laevis. Mech Dev. 2007;124:476–488. [PMC free article: PMC1973152] [PubMed: 17482434]
- 184.
- Munoz A, Wrighton C, Seliger B, Bernal J, Beug H. Thyroid hormone receptor/c-erbA: control of commitment and differentiation in the neuronal/chromaffin progenitor line PC12. J Cell Biol. 1993;121:423–438. [PMC free article: PMC2200090] [PubMed: 8385673]
- 185.
- Winter H, Braig C, Zimmermann U, Engel J, Rohbock K, Knipper M. Thyroid hormone receptor alpha1 is a critical regulator for the expression of ion channels during final differentiation of outer hair cells. Histochem Cell Biol. 2007;128:65–75. [PubMed: 17520268]
- 186.
- Flamant F, Poguet AL, Plateroti M, Chassande O, Gauthier K, Streichenberger N, Mansouri A, Samarut J. Congenital hypothyroid Pax8(-/-) mutant mice can be rescued by inactivating the TRalpha gene. Mol Endocrinol. 2002;16:24–32. [PubMed: 11773436]
- 187.
- Hashimoto K, Curty FH, Borges PP, Lee CE, Abel ED, Elmquist JK, Cohen RN, Wondisford FE. An unliganded thyroid hormone receptor causes severe neurological dysfunction. Proc Natl Acad Sci U S A. 2001;98:3998–4003. [PMC free article: PMC31168] [PubMed: 11274423]
- 188.
- Roberts MR, Srinivas M, Forrest D, Morreale de Escobar G, Reh TA. Making the gradient: thyroid hormone regulates cone opsin expression in the developing mouse retina. Proc Natl Acad Sci U S A. 2006;103:6218–6223. [PMC free article: PMC1458858] [PubMed: 16606843]
- 189.
- Cheng SY, Leonard JL, Davis PJ. Molecular aspects of thyroid hormone actions. Endocr Rev. 2010;31:139–170. [PMC free article: PMC2852208] [PubMed: 20051527]
- 190.
- Davis PJ, Davis FB, Cody V. Membrane receptors mediating thyroid hormone action. Trends Endocrinol Metab. 2005;16:429–435. [PubMed: 16214361]
- 191.
- Davis PJ, Leonard JL, Davis FB. Mechanisms of nongenomic actions of thyroid hormone. Front Neuroendocrinol. 2008;29:211–218. [PubMed: 17983645]
- 192.
- Stenzel D, Wilsch-Brauninger M, Wong FK, Heuer H, Huttner WB. Integrin alphavbeta3 and thyroid hormones promote expansion of progenitors in embryonic neocortex. Development. 2014;141:795–806. [PubMed: 24496617]
- 193.
- Martin NP, Marron Fernandez de Velasco E, Mizuno F, Scappini EL, Gloss B, Erxleben C, Williams JG, Stapleton HM, Gentile S, Armstrong DL. A rapid cytoplasmic mechanism for PI3 kinase regulation by the nuclear thyroid hormone receptor, TRbeta, and genetic evidence for its role in the maturation of mouse hippocampal synapses in vivo. Endocrinology. 2014;155:3713–3724. [PMC free article: PMC4138568] [PubMed: 24932806]
- 194.
- Yamaguchi S, Aoki N, Kitajima T, Iikubo E, Katagiri S, Matsushima T, Homma KJ. Thyroid hormone determines the start of the sensitive period of imprinting and primes later learning. Nat Commun. 2012;3:1081. [PMC free article: PMC3658000] [PubMed: 23011135]
- 195.
- Farsetti A, Desvergne B, Hallenbeck P, Robbins J, Nikodem V. Characterization of myelin basic protein thyroid hormone response element and its function in the context of native and heterologous promoter. J Biol Chem. 1992;267:15784–15788. [PubMed: 1379237]
- 196.
- Zou L, Hagen SC, Strait KA, Oppenheimer JH. Identification of thyroid hormone response elements in rodent Pcp-2, a developmentally regulated gene of cerebellar Purkinje cells. J Biol Chem. 1994;269:13346–13352. [PubMed: 8175765]
- 197.
- Martinez de Arrieta C, Morte B, Coloma A, Bernal J. The human RC3 gene homolog, NRGN contains a thyroid hormone-responsive element located in the first intron. Endocrinology. 1999;140:335–343. [PubMed: 9886843]
- 198.
- García-Fernández LF, Urade Y, Hayaishi O, Bernal J, Muñoz A. Identification of a thyroid hormone response element in the promoter region of the rat lipocalin-type prostaglandin D synthase (beta-trace) gene. Mol Brain Res. 1998;55:321–330. [PubMed: 9582446]
- 199.
- White DM, Takeda T, DeGroot LJ, Stefansson K, Arnason BG. Beta-trace gene expression is regulated by a core promoter and a distal thyroid hormone response element. J Biol Chem. 1997;272:14387–14393. [PubMed: 9162076]
- 200.
- Thompson CC, Bottcher MC. The product of a thyroid hormone-responsive gene interacts with thyroid hormone receptors. Proc Natl Acad Sci U S A. 1997;94:8527–8532. [PMC free article: PMC22986] [PubMed: 9238010]
- 201.
- Iglesias T, Caubin J, Stunnenberg HG, Zaballos A, Bernal J, Muñoz A. Thyroid hormone-dependent transcriptional repression of neural cell adhesion molecule during brain maturation. EMBO J. 1996;15:4307–4316. [PMC free article: PMC452156] [PubMed: 8861959]
- 202.
- Ghorbel MT, Seugnet I, Hadj-Sahraoui N, Topilko P, Levi G, Demeneix B. Thyroid hormone effects on Krox-24 transcription in the post-natal mouse brain are developmentally regulated but are not correlated with mitosis. Oncogene. 1999;18:917–924. [PubMed: 10023667]
- 203.
- Dong H, Yauk CL, Rowan-Carroll A, You SH, Zoeller RT, Lambert I, Wade MG. Identification of thyroid hormone receptor binding sites and target genes using ChIP-on-chip in developing mouse cerebellum. PLoS One. 2009;4:e4610. [PMC free article: PMC2643481] [PubMed: 19240802]
- 204.
- Silva JE, Rudas P. Effects of congenital hypothyroidism on microtubule-associated protein-2 expression in the cerebellum of the rat. Endocrinology. 1990;126:1276–1282. [PubMed: 2298164]
- 205.
- Aniello F, Couchie D, Bridoux AM, Gripois D, Nunez J. Splicing of juvenile and adult tau mRNA variants is regulated by thyroid hormone. Proc Natl Acad Sci U S A. 1991;88:4035–4039. [PMC free article: PMC51588] [PubMed: 1902578]
- 206.
- Cuadrado A, Garcia-Fernandez LF, Imai T, Okano H, Munoz A. Regulation of tau RNA maturation by thyroid hormone is mediated by the neural RNA-binding protein musashi-1. Mol Cell Neurosci. 2002;20:198–210. [PubMed: 12093154]
- 207.
- Dowling AL, Iannacone EA, Zoeller RT. Maternal Hypothyroidism Selectively Affects the Expression of Neuroendocrine-Specific Protein A Messenger Ribonucleic Acid in the Proliferative Zone of the Fetal Rat Brain Cortex. Endocrinology. 2001;142:390–399. [PubMed: 11145602]
- 208.
- Sampson D, Pickard MR, Sinha AK, Evans IM, Leonard AJ, Ekins RP. Maternal thyroid status regulates the expression of neuronal and astrocytic cytoskeletal proteins in the fetal brain. J Endocrinol. 2000;167:439–445. [PubMed: 11115770]
- 209.
- Sinha RA, Pathak A, Mohan V, Bandyopadhyay S, Rastogi L, Godbole MM. Maternal thyroid hormone: a strong repressor of neuronal nitric oxide synthase in rat embryonic neocortex. Endocrinology. 2008;149:4396–4401. [PubMed: 18467447]
- 210.
- Navarro D, Alvarado M, Morte B, Berbel D, Sesma J, Pacheco P, Morreale de Escobar G, Bernal J, Berbel P. Late maternal hypothyroidism alters the expression of Camk4 in neocortical subplate neurons: a comparison with Nurr1 labeling. Cereb Cortex. 2014;24:2694–2706. [PubMed: 23680840]
- 211.
- Krebs J, Honegger P. Calmodulin kinase IV: expression and function during rat brain development. Biochim Biophys Acta. 1996;1313:217–222. [PubMed: 8898857]
- 212.
- Liu YY, Brent GA. Thyroid hormone-dependent gene expression in differentiated embryonic stem cells and embryonal carcinoma cells: identification of novel thyroid hormone target genes by deoxyribonucleic acid microarray analysis. Endocrinology. 2005;146:776–783. [PubMed: 15550503]
- 213.
- Schwartz HL, Ross ME, Oppenheimer JH. Lack of effect of thyroid hormone on late fetal rat brain development. Endocrinology. 1997;138:3119–3124. [PubMed: 9231758]
- 214.
- Bagamasbad PD, Espina JEC, Knoedler JR, Subramani A, Harden AJ, Denver RJ. Coordinated transcriptional regulation by thyroid hormone and glucocorticoid interaction in adult mouse hippocampus-derived neuronal cells. PLoS One. 2019;14:e0220378. [PMC free article: PMC6660079] [PubMed: 31348800]
- 215.
- Bauer M, Heinz A, Whybrow PC. Thyroid hormones, serotonin and mood: of synergy and significance in the adult brain. Mol Psychiatry. 2002;7:140–156. [PubMed: 11840307]
- 216.
- Simon NM, Blacker D, Korbly NB, Sharma SG, Worthington JJ, Otto MW, Pollack MH. Hypothyroidism and hyperthyroidism in anxiety disorders revisited: new data and literature review. J Affect Disord. 2002;69:209–217. [PubMed: 12103468]
- 217.
- Bauer M, London ED, Rasgon N, Berman SM, Frye MA, Altshuler LL, Mandelkern MA, Bramen J, Voytek B, Woods R, Mazziotta JC, Whybrow PC. Supraphysiological doses of levothyroxine alter regional cerebral metabolism and improve mood in bipolar depression. Mol Psychiatry. 2005;10:456–469. [PubMed: 15724143]
- 218.
- Baumgartner A, Bauer M, Hellweg R. Treatment of intractable non-rapid cycling bipolar affective disorder with high-dose thyroxine: an open clinical trial. Neuropsychopharmacology. 1994;10:183–189. [PubMed: 7916915]
- 219.
- Niculescu AB 3rd, Segal DS, Kuczenski R, Barrett T, Hauger RL, Kelsoe JR. Identifying a series of candidate genes for mania and psychosis: a convergent functional genomics approach. Physiol Genomics. 2000;4:83–91. [PubMed: 11074017]
- 220.
- Kriegstein A, Alvarez-Buylla A. The glial nature of embryonic and adult neural stem cells. Annu Rev Neurosci. 2009;32:149–184. [PMC free article: PMC3086722] [PubMed: 19555289]
- 221.
- Hatten ME. Riding the glial monorail: a common mechanism for glial-guided neuronal migration in different regions of the developing mammalian brain. Trends Neurosci. 1990;13:179–184. [PubMed: 1693236]
- 222.
- Rakic P. Mode of cell migration to the superficial layers of fetal monkey neocortex. J Comp Neurol. 1972;145:61–83. [PubMed: 4624784]
- 223.
- Benito-Kwiecinski S, Giandomenico SL, Sutcliffe M, Riis ES, Freire-Pritchett P, Kelava I, Wunderlich S, Martin U, Wray GA, McDole K, Lancaster MA. An early cell shape transition drives evolutionary expansion of the human forebrain. Cell. 2021; 184:2084-2102 e2019. [PMC free article: PMC8054913] [PubMed: 33765444]
- 224.
- Denver RJ, Hu F, Scanlan TS, Furlow JD. Thyroid hormone receptor subtype specificity for hormone-dependent neurogenesis in Xenopus laevis. Dev Biol. 2009;326:155–168. [PubMed: 19056375]
- 225.
- Chen C, Zhou Z, Zhong M, Zhang Y, Li M, Zhang L, Qu M, Yang J, Wang Y, Yu Z. Thyroid hormone promotes neuronal differentiation of embryonic neural stem cells by inhibiting STAT3 signaling through TRalpha1. Stem Cells Dev. 2012;21:2667–2681. [PMC free article: PMC3438880] [PubMed: 22468949]
- 226.
- Mohan V, Sinha RA, Pathak A, Rastogi L, Kumar P, Pal A, Godbole MM. Maternal thyroid hormone deficiency affects the fetal neocorticogenesis by reducing the proliferating pool, rate of neurogenesis and indirect neurogenesis. Exp Neurol. 2012;237:477–488. [PubMed: 22892247]
- 227.
- Chantoux F, Francon J. Thyroid hormone regulates the expression of NeuroD/BHF1 during the development of rat cerebellum. Mol Cell Endocrinol. 2002;194:157–163. [PubMed: 12242038]
- 228.
- Krieger TG, Moran CM, Frangini A, Visser WE, Schoenmakers E, Muntoni F, Clark CA, Gadian D, Chong WK, Kuczynski A, Dattani M, Lyons G, Efthymiadou A, Varga-Khadem F, Simons BD, Chatterjee K, Livesey FJ. Mutations in thyroid hormone receptor alpha1 cause premature neurogenesis and progenitor cell depletion in human cortical development. Proc Natl Acad Sci U S A. 2019;116:22754–22763. [PMC free article: PMC6842615] [PubMed: 31628250]
- 229.
- Martinez-Galan JR, Pedraza P, Santacana M, Escobar del Ray F, Morreale de Escobar G, Ruiz-Marcos A. Early effects of iodine deficiency on radial glial cells of the hippocampus of the rat fetus. A model of neurological cretinism. J Clin Invest. 1997;99:2701–2709. [PMC free article: PMC508116] [PubMed: 9169500]
- 230.
- Ernst A, Alkass K, Bernard S, Salehpour M, Perl S, Tisdale J, Possnert G, Druid H, Frisen J. Neurogenesis in the striatum of the adult human brain. Cell. 2014;156:1072–1083. [PubMed: 24561062]
- 231.
- Spalding KL, Bergmann O, Alkass K, Bernard S, Salehpour M, Huttner HB, Bostrom E, Westerlund I, Vial C, Buchholz BA, Possnert G, Mash DC, Druid H, Frisen J. Dynamics of hippocampal neurogenesis in adult humans. Cell. 2013;153:1219–1227. [PMC free article: PMC4394608] [PubMed: 23746839]
- 232.
- Kapoor R, Fanibunda SE, Desouza LA, Guha SK, Vaidya VA. Perspectives on thyroid hormone action in adult neurogenesis. J Neurochem. 2015;133:599–616. [PubMed: 25772646]
- 233.
- Remaud S, Gothie JD, Morvan-Dubois G, Demeneix BA. Thyroid hormone signaling and adult neurogenesis in mammals. Front Endocrinol (Lausanne). 2014;5:62. [PMC free article: PMC4009442] [PubMed: 24808891]
- 234.
- Ambrogini P, Cuppini R, Ferri P, Mancini C, Ciaroni S, Voci A, Gerdoni E, Gallo G. Thyroid hormones affect neurogenesis in the dentate gyrus of adult rat. Neuroendocrinology. 2005;81:244–253. [PubMed: 16113586]
- 235.
- Desouza LA, Ladiwala U, Daniel SM, Agashe S, Vaidya RA, Vaidya VA. Thyroid hormone regulates hippocampal neurogenesis in the adult rat brain. Mol Cell Neurosci. 2005;29:414–426. [PubMed: 15950154]
- 236.
- Lemkine GF, Raj A, Alfama G, Turque N, Hassani Z, Alegria-Prevot O, Samarut J, Levi G, Demeneix BA. Adult neural stem cell cycling in vivo requires thyroid hormone and its alpha receptor. Faseb J. 2005;19:863–865. [PubMed: 15728663]
- 237.
- Montero-Pedrazuela A, Venero C, Lavado-Autric R, Fernandez-Lamo I, Garcia-Verdugo JM, Bernal J, Guadano-Ferraz A. Modulation of adult hippocampal neurogenesis by thyroid hormones: implications in depressive-like behavior. Mol Psychiatry. 2006;11:361–371. [PubMed: 16446739]
- 238.
- Fanibunda SE, Desouza LA, Kapoor R, Vaidya RA, Vaidya VA. Thyroid Hormone Regulation of Adult Neurogenesis. Vitam Horm. 2018;106:211–251. [PubMed: 29407437]
- 239.
- Kapoor R, Desouza LA, Nanavaty IN, Kernie SG, Vaidya VA. Thyroid hormone accelerates the differentiation of adult hippocampal progenitors. J Neuroendocrinol. 2012;24:1259–1271. [PubMed: 22497336]
- 240.
- Lopez-Juarez A, Remaud S, Hassani Z, Jolivet P, Pierre Simons J, Sontag T, Yoshikawa K, Price J, Morvan-Dubois G, Demeneix BA. Thyroid hormone signaling acts as a neurogenic switch by repressing Sox2 in the adult neural stem cell niche. Cell Stem Cell. 2012;10:531–543. [PubMed: 22560077]
- 241.
- Gothie JD, Sebillot A, Luongo C, Legendre M, Nguyen Van C, Le Blay K, Perret-Jeanneret M, Remaud S, Demeneix BA. Adult neural stem cell fate is determined by thyroid hormone activation of mitochondrial metabolism. Mol Metab. 2017;6:1551–1561. [PMC free article: PMC5681236] [PubMed: 29107300]
- 242.
- Pérez-Juste G, Aranda A. The cyclin-dependent kinase inhibitor p27(Kip1) is involved in thyroid hormone-mediated neuronal differentiation. J Biol Chem. 1999;19:5026–5031. [PubMed: 9988748]
- 243.
- Qi JS, Yuan Y, Desai-Yajnik V, Samuels HH. Regulation of the mdm2 oncogene by thyroid hormone receptor. Mol Cell Biol. 1999;19:864–872. [PMC free article: PMC83943] [PubMed: 9858609]
- 244.
- Wood WM, Sarapura VD, Dowding JM, Woodmansee WW, Haakinson DJ, Gordon DF, Ridgway EC. Early gene expression changes preceding thyroid hormone-induced involution of a thyrotrope tumor. Endocrinology. 2002;143:347–359. [PubMed: 11796486]
- 245.
- Aniello F, Couchie D, Gripois D, Nunez J. Regulation of five tubulin isotypes by thyroid hormone during brain development. J Neurochem. 1991;57:1781–1786. [PubMed: 1717658]
- 246.
- Lorenzo PI, Menard C, Miller FD, Bernal J. Thyroid hormone-dependent regulation of Ta1 a-tubulin during brain development. Mol Cell Neurosci. 2002;19:333–343. [PubMed: 11906207]
- 247.
- Lima FR, Goncalves N, Gomes FC, de Freitas MS, Moura Neto V. Thyroid hormone action on astroglial cells from distinct brain regions during development. Int J Dev Neurosci. 1998;16:19–27. [PubMed: 9664219]
- 248.
- Manzano J, Bernal J, Morte B. Influence of thyroid hormones on maturation of rat cerebellar astrocytes. Int J Dev Neurosci. 2007;25:171–179. [PubMed: 17408906]
- 249.
- Clos J, Legrand C, Legrand J. Effects of thyroid state on the formation and early morphological development of bergmann glia in the developing rat cerebellum. Dev Neurosci. 1980;3:199–208. [PubMed: 7460792]
- 250.
- Alvarez-Dolado M, Cuadrado A, Navarro-Yubero C, Sonderegger P, Furley AJ, Bernal J, Muñoz A. Regulation of the L1 cell adhesion molecule by thyroid hormone in the developing brain. Mol Cell Neurobiol. 2000; 499-514. [PubMed: 11085884]
- 251.
- Alvarez-Dolado M, Gonzalez-Sancho JM, Bernal J, Muñoz A. Developmental expression of tenascin-C is altered by hypothyroidism in the rat brain. Neurosci. 1998;84:309–322. [PubMed: 9580330]
- 252.
- Farwell AP, Dubord-Tomasetti SA. Thyroid hormone regulates the expression of laminin in the developing rat cerebellum. Endocrinology. 1999;140:4221–4227. [PubMed: 10465295]
- 253.
- Gharami K, Das S. Thyroid hormone-induced morphological differentiation and maturation of astrocytes are mediated through the beta-adrenergic receptor. J Neurochem. 2000;75:1962–1969. [PubMed: 11032885]
- 254.
- Das M, Ghosh M, Das S. Thyroid Hormone-Induced Differentiation of Astrocytes is Associated with Transcriptional Upregulation of beta-arrestin-1 and beta-adrenergic Receptor-Mediated Endosomal Signaling. Mol Neurobiol. 2016;53:5178–5190. [PubMed: 26399643]
- 255.
- Alvarez-Dolado M, Iglesias T, Rodríguez-Peña A, Bernal J, Muñoz A. Expression of neurotrophins and the trk family of neurotrophin receptors in normal and hypothyroid rat brain. Mol Brain Res. 1994;27:249–257. [PubMed: 7898308]
- 256.
- Koibuchi N, Yamaoka S, Chin WW. Effect of altered thyroid status on neurotrophin gene expression during postnatal development of the mouse cerebellum. Thyroid. 2001;11:205–210. [PubMed: 11327610]
- 257.
- Lindholm D, Castren E, Tsoulfas P, Kolbeck R, Penha Berzaghi M, Leingartner A, Heisenberg CP, Tesarollo L. parada LF, Thoenen H. Neurotrophin-3 induced by tri-iodothyronine in cerebellar granullar cells promotes purkinje cell differentiation. J Cell Biol. 1993;122:443–450. [PMC free article: PMC2119654] [PubMed: 8320266]
- 258.
- Schoonover CM, Seibel MM, Jolson DM, Stack MJ, Rahman RJ, Jones SA, Mariash CN, Anderson GW. Thyroid hormone regulates oligodendrocyte accumulation in developing rat brain white matter tracts. Endocrinology. 2004;145:5013–5020. [PubMed: 15256491]
- 259.
- Lee JY, Petratos S. Thyroid Hormone Signaling in Oligodendrocytes: from Extracellular Transport to Intracellular Signal. Mol Neurobiol. 2016;53:6568–6583. [PubMed: 27427390]
- 260.
- Berbel P, Guadaño-Ferraz A, Angulo A, Cerezo JR. Role of thyroid hormones in the maturation of interhemispheric connections in rat. Behav Brain Res. 1994;64:9–14. [PubMed: 7840896]
- 261.
- Gravel C, Sasseville R, Hawkes R. Maturation of the corpus callosum of the rat: II. Influence of thyroid hormones on the number and maturation of axons. J Comp Neurol. 1990;291:147–161. [PubMed: 2298928]
- 262.
- Notterpek LM, Rome LH. Functional evidence for the role of axolemma in CNS myelination. Neuron. 1994;13:473–485. [PubMed: 8060622]
- 263.
- Ibarrola N, Rodriguez-Peña A. Hypothyroidism coordinately and transiently affects myelin proein gene expression in most rat brain regions during postnatal development. Brain Res. 1997;752:285–293. [PubMed: 9106469]
- 264.
- Johe KK, Hazel TG, Muller T, Dugich-Djordjevic MM, McKay RD. Single factors direct the differentiation of stem cells from the fetal and adult central nervous system. Genes Dev. 1996;10:3129–3140. [PubMed: 8985182]
- 265.
- Gao FB, Apperly J, Raff M. Cell-intrinsic timers and thyroid hormone regulate the probability of cell-cycle withdrawal and differentiation of oligodendrocyte precursor cells. Dev Biol. 1998;197:54–66. [PubMed: 9578618]
- 266.
- Nygard M, Wahlstrom GM, Gustafsson MV, Tokumoto YM, Bondesson M. Hormone-Dependent Repression of the E2F-1 Gene by Thyroid Hormone Receptors. Mol Endocrinol. 2003;17:79–92. [PubMed: 12511608]
- 267.
- Dugas JC, Ibrahim A, Barres BA. The T3-induced gene KLF9 regulates oligodendrocyte differentiation and myelin regeneration. Mol Cell Neurosci. 2012;50:45–57. [PMC free article: PMC4441621] [PubMed: 22472204]
- 268.
- Castelo-Branco G, Lilja T, Wallenborg K, Falcao AM, Marques SC, Gracias A, Solum D, Paap R, Walfridsson J, Teixeira AI, Rosenfeld MG, Jepsen K, Hermanson O. Neural stem cell differentiation is dictated by distinct actions of nuclear receptor corepressors and histone deacetylases. Stem Cell Reports. 2014;3:502–515. [PMC free article: PMC4266002] [PubMed: 25241747]
- 269.
- Billon N, Jolicoeur C, Tokumoto Y, Vennstrom B, Raff M. Normal timing of oligodendrocyte development depends on thyroid hormone receptor alpha 1 (TRalpha1). Embo J. 2002;21:6452–6460. [PMC free article: PMC136965] [PubMed: 12456652]
- 270.
- Billon N, Tokumoto Y, Forrest D, Raff M. Role of thyroid hormone receptors in timing oligodendrocyte differentiation. Dev Biol. 2001;235:110–120. [PubMed: 11412031]
- 271.
- Baxi EG, Schott JT, Fairchild AN, Kirby LA, Karani R, Uapinyoying P, Pardo-Villamizar C, Rothstein JR, Bergles DE, Calabresi PA. A selective thyroid hormone beta receptor agonist enhances human and rodent oligodendrocyte differentiation. Glia. 2014;62:1513–1529. [PMC free article: PMC4107024] [PubMed: 24863526]
- 272.
- Berbel P, Auso E, Garcia-Velasco JV, Molina ML, Camacho M. Role of thyroid hormones in the maturation and organisation of rat barrel cortex. Neuroscience. 2001;107:383–394. [PubMed: 11718994]
- 273.
- Berbel P, Guadano-Ferraz A, Martinez M, Quiles JA, Balboa R, Innocenti GM. Organization of auditory callosal connections in hypothyroid adult rats. Eur J Neurosci. 1993;5:1465–1478. [PubMed: 7506971]
- 274.
- Marin-Padilla M. Three-dimensional structural organization of layer I of the human cerebral cortex: a Golgi study. J Comp Neurol. 1990;299:89–105. [PubMed: 2212113]
- 275.
- Del Rio JA, Heimrich B, Borrell V, Forster E, Drakew A, Alcantara S, Nakajima K, Miyata T, Ogawa M, Mikoshiba K, Derer P, Frotscher M, Soriano E. A role for Cajal-Retzius cells and reelin in the development of hippocampal connections. Nature. 1997;385:70–74. [PubMed: 8985248]
- 276.
- Rakic P. Evolution of the neocortex: a perspective from developmental biology. Nat Rev Neurosci. 2009;10:724–735. [PMC free article: PMC2913577] [PubMed: 19763105]
- 277.
- García-Fernández LF, Rausell E, Urade Y, Hayaishi O, Bernal J, Muñoz A. Hypothyroidism alters the expression of prostaglandin D2 synthase/beta trace in specific areas of the developing rat brain. Eur J Neurosci. 1997;9:1566–1573. [PubMed: 9283811]
- 278.
- Laslett AL, Li LH, Jester WF Jr, Orth JM. Thyroid hormone down-regulates neural cell adhesion molecule expression and affects attachment of gonocytes in Sertoli cell-gonocyte cocultures. Endocrinology. 2000;141:1633–1641. [PubMed: 10803571]
- 279.
- Avci HX, Lebrun C, Wehrle R, Doulazmi M, Chatonnet F, Morel MP, Ema M, Vodjdani G, Sotelo C, Flamant F, Dusart I. Thyroid hormone triggers the developmental loss of axonal regenerative capacity via thyroid hormone receptor alpha1 and kruppel-like factor 9 in Purkinje cells. Proc Natl Acad Sci U S A. 2012;109:14206–14211. [PMC free article: PMC3435228] [PubMed: 22891348]
- 280.
- Fauquier T, Chatonnet F, Picou F, Richard S, Fossat N, Aguilera N, Lamonerie T, Flamant F. Purkinje cells and Bergmann glia are primary targets of the TRalpha1 thyroid hormone receptor during mouse cerebellum postnatal development. Development. 2014;141:166–175. [PubMed: 24346699]
- 281.
- Nicholson JL, Altman J. The effects of early hypo- and hyperthyroidism on the development of the rat cerebellar cortex. I. Cell proliferation and differentiation. Brain Res. 1972;44:13–23. [PubMed: 5056973]
- 282.
- Desouza LA, Sathanoori M, Kapoor R, Rajadhyaksha N, Gonzalez LE, Kottmann AH, Tole S, Vaidya VA. Thyroid hormone regulates the expression of the sonic hedgehog signaling pathway in the embryonic and adult Mammalian brain. Endocrinology. 2011;152:1989–2000. [PMC free article: PMC3179409] [PubMed: 21363934]
- Review Cellular Uptake of Thyroid Hormones.[Endotext. 2000]Review Cellular Uptake of Thyroid Hormones.Visser TJ. Endotext. 2000
- Single-Cell Transcriptome Profiling of Thyroid Hormone Effectors in the Human Fetal Neocortex: Expression of SLCO1C1, DIO2, and THRB in Specific Cell Types.[Thyroid. 2021]Single-Cell Transcriptome Profiling of Thyroid Hormone Effectors in the Human Fetal Neocortex: Expression of SLCO1C1, DIO2, and THRB in Specific Cell Types.Diez D, Morte B, Bernal J. Thyroid. 2021 Oct; 31(10):1577-1588. Epub 2021 Jul 29.
- Lack of action of exogenously administered T3 on the fetal rat brain despite expression of the monocarboxylate transporter 8.[Endocrinology. 2011]Lack of action of exogenously administered T3 on the fetal rat brain despite expression of the monocarboxylate transporter 8.Grijota-Martínez C, Díez D, Morreale de Escobar G, Bernal J, Morte B. Endocrinology. 2011 Apr; 152(4):1713-21. Epub 2011 Feb 8.
- Brain Gene Expression in Systemic Hypothyroidism and Mouse Models of MCT8 Deficiency: The Mct8-Oatp1c1-Dio2 Triad.[Thyroid. 2021]Brain Gene Expression in Systemic Hypothyroidism and Mouse Models of MCT8 Deficiency: The Mct8-Oatp1c1-Dio2 Triad.Morte B, Gil-Ibañez P, Heuer H, Bernal J. Thyroid. 2021 Jun; 31(6):985-993. Epub 2021 Mar 18.
- Review Mechanisms of disease: psychomotor retardation and high T3 levels caused by mutations in monocarboxylate transporter 8.[Nat Clin Pract Endocrinol Meta...]Review Mechanisms of disease: psychomotor retardation and high T3 levels caused by mutations in monocarboxylate transporter 8.Friesema EC, Jansen J, Heuer H, Trajkovic M, Bauer K, Visser TJ. Nat Clin Pract Endocrinol Metab. 2006 Sep; 2(9):512-23.
- Thyroid Hormones in Brain Development and Function - EndotextThyroid Hormones in Brain Development and Function - Endotext
Your browsing activity is empty.
Activity recording is turned off.
See more...