By agreement with the publisher, this book is accessible by the search feature, but cannot be browsed.
NCBI Bookshelf. A service of the National Library of Medicine, National Institutes of Health.
Alberts B, Bray D, Lewis J, et al. Molecular Biology of the Cell. 3rd edition. New York: Garland Science; 1994.

Molecular Biology of the Cell. 3rd edition.
Show detailsIntroduction
Single-cell organisms, such as bacteria and protozoa, have been so successful in adapting to a variety of different environments that they comprise more than half of the total biomass on earth. Unlike animals, many of these unicellular organisms can synthesize all of the substances they need from a few simple nutrients, and some of them divide more than once every hour. What, then, was the selective advantage that led to the evolution of multicellular organisms?
A short answer is that by collaboration and by division of labor it becomes possible to exploit resources that no single cell could utilize so well. This principle, applying at first to simple associations of cells, has been taken to an extreme in the multicellular organisms we see today. Multicellularity enables a plant, for example, to become physically large; to have roots in the ground, where one set of cells can take up water and nutrients; and to have leaves in the air, where another set of cells can efficiently capture radiant energy from the sun. Specialized cells in the stem of the plant form channels for transporting water and nutrients between the roots and the leaves. Yet another set of specialized cells forms a layer of epidermis to prevent water loss and to provide a protected internal environment (see Panel 1-2, pp. 28-29). The plant as a whole does not compete directly with unicellular organisms for its ecological niche; it has found a radically different way to survive and propagate.
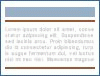
Panel 1-2>
The cell types and tissues from which higher plants are constructed.
As different animals and plants appeared, they changed the environment in which further evolution occurred. Survival in a jungle calls for different talents than survival in the open sea. Innovations in movement, sensory detection, communication, social organization - all enabled eucaryotic organisms to compete, propagate, and survive in ever more complex ways.
Single Cells Can Associate to Form Colonies
It seems likely that an early step in the evolution of multicellular organisms was the association of unicellular organisms to form colonies. The simplest way of achieving this is for daughter cells to remain together after each cell division. Even some procaryotic cells show such social behavior in a primitive form. Myxobacteria, for example, live in the soil and feed on insoluble organic molecules that they break down by secreting degradative enzymes. They stay together in loose colonies in which the digestive enzymes secreted by individual cells are pooled, thus increasing the efficiency of feeding (the "wolf-pack" effect). These cells indeed represent a peak of social sophistication among procaryotes, for when food supplies are exhausted, the cells aggregate tightly together and form a multicellular fruiting body (Figure 1-31), within which the bacteria differentiate into spores that can survive even in extremely hostile conditions. When conditions are more favorable, the spores in a fruiting body germinate to produce a new swarm of bacteria.
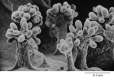
Figure 1-31
Fruiting bodies formed by a myxobacterium (Chondromyces crocatus), seen by scanning electron microscopy. Each fruiting body, packed with spores, is created by the aggregation and differentiation of about a million myxobacteria. (From P.L. Grilione and (more...)
Green algae (not to be confused with the procaryotic "blue-green algae" or cyanobacteria) are eucaryotes that exist as unicellular, colonial, or multicellular forms (Figure 1-32). Different species of green algae can be arranged in order of complexity, illustrating the kind of progression that probably occurred in the evolution of higher plants and animals. Unicellular green algae, such as Chlamydomonas, resemble flagellated protozoa except that they possess chloroplasts, which enable them to carry out photosynthesis. In closely related genera, groups of flagellated cells live in colonies held together by a matrix of extracellular molecules secreted by the cells themselves. The simplest species (those of the genus Gonium) have the form of a concave disc made of 4, 8, 16, or 32 cells. Their flagella beat independently, but since they are all oriented in the same direction, they are able to propel the colony through the water. Each cell is equivalent to every other, and each can divide to give rise to an entirely new colony. Larger colonies are found in other genera, the most spectacular being Volvox, some of whose species have as many as 50,000 or more cells linked together to form a hollow sphere. In Volvox the individual cells forming a colony are connected by fine cytoplasmic bridges so that the beating of their flagella is coordinated to propel the entire colony along like a rolling ball (see Figure 1-32). Within the Volvox colony there is some division of labor among cells, with a small number of cells being specialized for reproduction and serving as precursors of new colonies. The other cells are so dependent on one another that they cannot live in isolation, and the organism dies if the colony is disrupted.
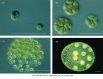
Figure 1-32
Four closely related genera of green algae, showing a progression from unicellular to colonial and multicellular organization. (Courtesy of David Kirk.)
The Cells of a Higher Organism Become Specialized and Cooperate
In some ways Volvox is more like a multicellular organism than a simple colony. All of its flagella beat in synchrony as it spins through the water, and the colony is structurally and functionally polarized and can swim toward a distant source of light. The reproductive cells are usually confined to one end of the colony, where they divide to form new miniature colonies, which are initially sheltered inside the parent sphere. Thus, in a primitive way, Volvox displays the two essential features of all multicellular organisms: its cells become specialized, and they cooperate. By specialization and cooperation the cells combine to form a coordinated single organism with more capabilities than any of its component parts.
Organized patterns of cell differentiation occur even in some procaryotes. For example, many kinds of cyanobacteria remain together after cell division, forming filamentous chains that can be as much as a meter in length. At regular intervals along the filament, individual cells take on a distinctive character and become able to incorporate atmospheric nitrogen into organic molecules. These few specialized cells perform nitrogen fixation for their neighbors and share the products with them. But eucaryotic cells appear to be very much better at this sort of organized division of labor; they, and not procaryotes, are the living units from which all the more complex multicellular organisms are constructed.
Multicellular Organization Depends on Cohesion Between Cells
To form a multicellular organism, the cells must be somehow bound together, and eucaryotes have evolved a number of different ways to satisfy this need. In Volvox, as noted above, the cells do not separate entirely at cell division but remain connected by cytoplasmic bridges. In higher plants the cells not only remain connected by cytoplasmic bridges (called plasmodesmata), they also are imprisoned in a rigid honeycomb of chambers walled with cellulose that the cells themselves have secreted (cell walls).
The cells of most animals do not have rigid walls, and cytoplasmic bridges are unusual. Instead, the cells are bound together by a relatively loose meshwork of large extracellular organic molecules (called the extracellular matrix) and by adhesions between their plasma membranes. Very often, side-to-side attachments between the cells hold them together to form a multicellular sheet, or epithelium.
Epithelial Sheets of Cells Enclose a Sheltered Internal Environment
Of all the ways in which animal cells are woven together into multicellular tissues, the epithelial arrangement is perhaps the most fundamentally important. The epithelial sheet has much the same significance for the evolution of complex multicellular organisms that the cell membrane has for the evolution of complex single cells.
The importance of epithelial sheets is well illustrated in the lowly group of animals known as coelenterates. The group includes sea anemones, jellyfish, and corals, as well as the small freshwater organism Hydra. Coelenterates are constructed from two layers of epithelium, the outer layer being the ectoderm, the inner being the endoderm. The endodermal layer surrounds a cavity, the coelenteron, in which food is digested (Figure 1-33). Among the endodermal cells are some that secrete digestive enzymes into the coelenteron, while other cells absorb and further digest the nutrient molecules that these enzymes release. By forming a tightly coherent epithelial sheet that prevents all these molecules from being lost to the exterior, the endodermal cells create for themselves an environment in the coelenteron that is suited to their own digestive tasks. Meanwhile, the ectodermal cells, facing the exterior, remain specialized for encounters with the outside world. In the ectoderm, for example, are cells that contain a poison capsule with a coiled dart that can be unleashed to kill the small animals on which Hydra feeds. The majority of other ectodermal and endodermal cells have musclelike properties, enabling Hydra to move, as a predator must.
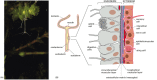
Figure 1-33
The body plan of Hydra. (A) Hydra oligactis in its natural environment; in this species of Hydra, the tentacles hang down to catch prey. The projections budding from the side of the body are progeny that will eventually detach from their parent. (B) Diagram (more...)
Within the double layer of ectoderm and endoderm is another compartment, separate both from the coelenteron and from the outside world. Here nerve cells lie, occupying narrow enclosed spaces between the epithelial cells, below the external surface where the specialized cell junctions between the epithelial cells form an impermeable barrier. The animal can change its shape and move by contractions of the musclelike cells in the epithelia, and it is the nerve cells that convey electrical signals to control and coordinate these contractions (Figures 1-33, 1-34, and 1-35). As we shall see later, the concentrations of simple inorganic ions in the medium surrounding a nerve cell are crucial for its function. Most nerve cells - our own included - are designed to operate when bathed in a solution with an ionic composition roughly similar to that of seawater. This may well reflect the conditions under which the first nerve cells evolved. Most coelenterates still live in the sea, but not all. Hydra, in particular, lives in fresh water. It has evidently been able to colonize this new habitat only because its nerve cells are contained in a space that is sealed and isolated from the exterior within sheets of epithelial cells that maintain the internal environment necessary for nerve cell function.
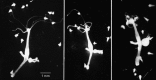
Figure 1-34
Hydra feeding. Feeding is one of a range of fairly complex activities this animal can perform. A single Hydra is photographed catching small water fleas in its tentacles; in the last panel it is stuffing these prey into its coelenteron for digestion. (more...)
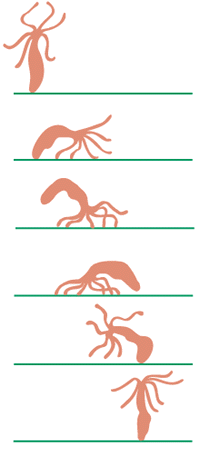
Figure 1-35
Hydra traveling. A Hydra can swim, glide on its base, or, as shown here, travel by somersaulting.
Cell-Cell Communication Controls the Spatial Pattern of Multicellular Organisms19
The cells of Hydra are not only bound together mechanically and connected by junctions that seal off the interior from the exterior environment, they also communicate with one another along the length of the body. If one end of a Hydra is cut off, the remaining cells react to the absence of the amputated part by adjusting their characters and rearranging themselves so as to regenerate a complete animal. Evidently, signals pass from one part of the organism to the other, governing the development of its body pattern - with tentacles and a mouth at one end and a foot at the other. Moreover, these signals are independent of the nervous system. If a developing Hydra is treated with a drug that prevents nerve cells from forming, the animal is unable to move about, catch prey, or feed itself. Its digestive system still functions normally, however, so that it can be kept alive by anyone with the patience to stuff its normal prey into its mouth. In such force-fed animals the body pattern is maintained, and lost parts are regenerated just as well as in an animal that has an intact nervous system.
The vastly more complex higher animals have evolved from simpler ancestors resembling coelenterates, and these higher animals owe their complexity to more sophisticated exploitation of the same basic principles of cell cooperation that underlie the construction of Hydra. Epithelial sheets of cells line all external and internal surfaces in the body, creating sheltered compartments and controlled internal environments in which specialized functions are performed by differentiated cells. Specialized cells interact and communicate with one another, setting up signals to govern the character of each cell according to its place in the structure as a whole. To show how it is possible to generate multicellular organisms of such size, precision, and complexity as a tree, a fly, or a mammal, however, it is necessary to consider more closely the sequence of events in development.
Cell Memory Permits the Development of Complex Patterns
The cells of almost every multicellular organism are generated by repeated division from a single precursor cell; they constitute a clone. As proliferation continues and the clone grows, some of the cells, as we have seen, become differentiated from others, adopting a different structure, a different chemistry, and a different function, usually in response to cues from their neighbors. It is remarkable that eucaryotic cells and their progeny will usually persist in their differently specialized states even after the influences that originally directed their differentiation have disappeared - in other words, these cells have a memory. Consequently, their final character is not determined simply by their final environment, but rather by the entire sequence of influences to which the cells have been exposed in the course of development. Thus as the body grows and matures, progressively finer details of the adult body pattern become specified, creating an organism of gradually increasing complexity whose ultimate form is the expression of a long developmental history.
Basic Developmental Programs Tend to Be Conserved in Evolution20
The final structure of an animal or plant reflects its evolutionary history, which, like development, presents a chronicle of progress from the simple to the complex. What then is the connection between the two perspectives, of evolution on the one hand and development on the other?
During evolution many of the developmental devices that evolved in the simplest multicellular organisms have been conserved as basic principles for the construction of their more complex descendants. We have already mentioned, for example, the organization of cells into epithelia. Some specialized cell types, such as nerve cells, are found throughout nearly the whole of the animal kingdom, from Hydra to humans. Molecular studies, to be discussed later in this book, reveal an astonishing number of developmental resemblances at a fundamental genetic level, even between species as remotely related as mammals and insects. In terms of anatomy, furthermore, early developmental stages of animals whose adult forms appear radically different are often surprisingly similar; it takes an expert eye to distinguish, for example, a young chick embryo from a young human embryo (Figure 1-36).
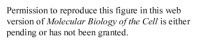
Figure 1-36
Comparison of the embryonic development of a fish, an amphibian, a reptile, a bird, and a selection of mammals. The early stages (above) are very similar; the later stages (below) are more divergent. The earliest stages are drawn roughly to scale; the (more...)
Such observations are not difficult to understand. Consider the process by which a new anatomical feature - say, an elongated beak - appears in the course of evolution. A random mutation occurs that changes the amino acid sequence of a protein or the timing of its synthesis and hence its biological activity. This alteration may, by chance, affect the cells responsible for the formation of the beak in such a way that they make one that is longer. But the mutation must also be compatible with the development of the rest of the organism; only then will it be propagated by natural selection. There would be little selective advantage in forming a longer beak if, in the process, the tongue was lost or the ears failed to develop. A catastrophe of this type is more likely if the mutation affects events occurring early in development than if it affects those near the end. The early cells of an embryo are like cards at the bottom of a house of cards - a great deal depends on them, and even small changes in their properties are likely to result in disaster. Fundamental steps appear to have been "frozen" into developmental processes, just as the genetic code or protein synthesis mechanisms have become frozen into the basic biochemical organization of the cell. In contrast, cells produced near the end of development (or produced early but forming accessory structures such as the placenta that are not incorporated in the adult body) have more freedom to change. It is presumably for this reason that the embryos of different species so often resemble each other in their early stages and, as they develop, seem sometimes to replay the steps of evolution.
The Cells of the Vertebrate Body Exhibit More Than 200 Different Modes of Specialization
The wealth of diverse specializations to be found among the cells of a higher animal is far greater than any procaryote can show. In a vertebrate more than 200 distinct cell types are plainly distinguishable, and many of these types of cells certainly include, under a single name, a large number of more subtly different varieties. Panel 1-3 (pp. 36-37) shows a small selection. In this profusion of specialized behaviors one can see displayed, in a single organism, the astonishing versatility of the eucaryotic cell. Much of our current knowledge of the general properties of eucaryotic cells has depended on the study of such specialized types of cells, because they demonstrate exceptionally well particular features on which all cells depend in some measure. Each feature and each organelle of the prototype that we have outlined in Panel 1-1 (pp. 18-19) is developed to an unusual degree or revealed with special clarity in one cell type or another. To take one arbitrary example, consider the neuromuscular junction, where just three types of cells are involved: a muscle cell, a nerve cell, and a Schwann cell. Each has a very different role (Figure 1-37):
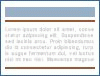
Panel 1-3
Some of the different types of cells present in the vertebrate body.
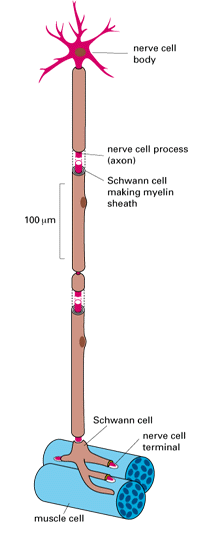
Figure 1-37
A nerve cell, with its associated Schwann cells, contacting a muscle cell at a neuromuscular junction. Schematic diagram.
1. The muscle cell has made contraction its specialty. Its cytoplasm is packed with organized arrays of protein filaments, including vast numbers of actin filaments. There are also many mitochondria interspersed among the protein filaments, supplying ATP as fuel for the contractile apparatus.
2. The nerve cell stimulates the muscle to contract, conveying an excitatory signal to the muscle from the brain or spinal cord. The nerve cell is therefore extraordinarily elongated: its main body, containing the nucleus, may lie a meter or more from the junction with the muscle. The cytoskeleton is consequently well developed so as to maintain the unusual shape of the cell and to transport materials efficiently from one end of the cell to the other. The most crucial specialization of the nerve cell, however, is its plasma membrane, which contains proteins that act as ion pumps and ion channels, causing a movement of ions that is equivalent to a flow of electricity. Whereas all cells contain such pumps and channels in their plasma membranes, the nerve cell has exploited them in such a way that a pulse of electricity can propagate in a fraction of a second from one end of the cell to the other, conveying a signal for action.
3. Lastly, Schwann cells are specialists in the mass production of plasma membrane, which they wrap around the elongated portion of the nerve cell, laying down layer upon layer of membrane like a roll of tape, to form a myelin sheath that serves as insulation.
Genes Can Be Switched On and Off
The various specialized cell types in a single higher plant or animal appear as different from one another as any cells could be. This seems paradoxical, since all of the cells in a multicellular organism are closely related, having recently descended from the same precursor cell - the fertilized egg. Common lineage implies similar genes; how then do the differences arise? In a few cases cell specialization involves the loss of genetic material. An extreme example is the mammalian red blood cell, which loses its entire nucleus in the course of differentiation. But the overwhelming majority of cells in most plant and animal species retain all of the genetic information contained in the fertilized egg. Specialization depends on changes in gene expression, not on the loss or acquisition of genes.
Even bacteria do not make all of their types of protein all of the time but are able to adjust the level of synthesis according to external conditions. Proteins required specifically for the metabolism of lactose, for example, are made by many bacteria only when this sugar is available for use; and when conditions are unfavorable for cell proliferation, some bacteria arrest most of their normal metabolic processes and form spores, which have tough, impermeable outer walls and a cytoplasm of altered composition.
Eucaryotic cells have evolved far more sophisticated mechanisms for controlling gene expression, and these affect entire systems of interacting gene products. Groups of genes are activated or repressed in response to both external and internal signals. Membrane composition, cytoskeleton, secretory products, even metabolism - all these and other features must change in a coordinated manner when cells become differentiated. The radical differences of character between cell types reflect stable changes in gene expression. The controls that bring about these changes have evolved in eucaryotes to a degree unmatched in procaryotes, defining the complex rules of cell behavior that can generate an organized multicellular organism from a single egg.
Sequence Comparisons Reveal Hundreds of Families of Homologous Genes12, 21
To outward appearances, evolution has transformed the universe of living things to such a degree that they are no longer recognizable as relatives. A human being, a fly, a daisy, a yeast, a bacterium - they seem so different that it scarcely makes sense to compare them. Yet all are descendants of one ancestor, and as we probe their inner workings more and more deeply, we find more and more evidence of their common origins. We now know that the basic molecular machinery of life has been conserved to an extent that would surely have astonished the originators of the theory of evolution. As we have seen, all life forms have essentially the same chemistry, based on amino acids, sugars, fatty acids, and nucleotides; all synthesize these chemical constituents in an essentially similar way; all store their genetic information in DNA and express it through RNA and protein. But the degree of evolutionary conservatism becomes even more striking when we examine the detailed sequences of nucleotides in specific genes and of amino acids in specific proteins. The chances are that the bacterial enzyme catalyzing any particular common reaction, such as the splitting of a six-carbon sugar into two three-carbon sugars in glycolysis, will have an amino acid sequence (and a three-dimensional structure) unmistakably similar to the enzyme catalyzing the same reaction in human beings. The two enzymes - and, equivalently, the genes that specify them - not only have a similar function, but also almost certainly a common evolutionary origin. One can exploit these relationships to trace ancient evolutionary pathways; and by comparing gene sequences and recognizing homologies, one discovers hidden parallels and similarities between different organisms.
Family resemblances are also often found among genes coding for proteins that carry out related functions within a single organism. These genes are also evolutionarily related, and their existence reveals a basic strategy by which increasingly complex organisms have arisen: genes and portions of genes become duplicated, and the new copies then diverge from the old by mutation and recombination to serve new, additional purposes. In this way, starting from a relatively small set of genes in primitive cells, the more complex life forms have been able to evolve the more than 50,000 genes thought to be present in a higher animal or plant. From an understanding of one gene or protein, we consequently gain insight into a whole family of others homologous to it. Thus molecular biology both underscores the unity of the living world and gives us tools to discover the general mechanisms that underlie its endless variety of inventions.
In the next chapter we begin our account of these mechanisms with a discussion of the most basic components of the biological construction kit - the small molecules from which all larger components of living cells are made.
Summary
The evolution of large multicellular organisms depended on the ability of eucaryotic cells to express their hereditary information in many different ways and to function cooperatively in a single collective. In animals one of the earliest developments was probably the formation of epithelial cell sheets, which separate the internal space of the body from the exterior. In addition to epithelial cells, primitive differentiated cell types would have included nerve cells, muscle cells, and connective tissue cells, all of which can be found in very simple present-day animals. The evolution of higher animals and plants (Figure 1-38) depended on production of an increasing number of specialized cell types and more sophisticated methods of coordination among them, reflecting an increasingly elaborate system of controls over gene expression in the individual component cells.
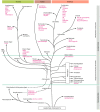
Figure 1-38
Evolutionary relationships among some of the organisms mentioned in this book. The branches of the evolutionary tree show paths of descent but do not indicate by their length the passage of time. (Note, similarly, that the vertical axis of the diagram shows (more...)
- Introduction
- Single Cells Can Associate to Form Colonies
- The Cells of a Higher Organism Become Specialized and Cooperate
- Multicellular Organization Depends on Cohesion Between Cells
- Epithelial Sheets of Cells Enclose a Sheltered Internal Environment
- Cell-Cell Communication Controls the Spatial Pattern of Multicellular Organisms
- Cell Memory Permits the Development of Complex Patterns
- Basic Developmental Programs Tend to Be Conserved in Evolution
- The Cells of the Vertebrate Body Exhibit More Than 200 Different Modes of Specialization
- Genes Can Be Switched On and Off
- Sequence Comparisons Reveal Hundreds of Families of Homologous Genes
- Summary
- From Single Cells to Multicellular Organisms - Molecular Biology of the CellFrom Single Cells to Multicellular Organisms - Molecular Biology of the Cell
Your browsing activity is empty.
Activity recording is turned off.
See more...