NCBI Bookshelf. A service of the National Library of Medicine, National Institutes of Health.
Feingold KR, Anawalt B, Blackman MR, et al., editors. Endotext [Internet]. South Dartmouth (MA): MDText.com, Inc.; 2000-.
ABSTRACT
The testes synthesize two important products: testosterone, needed for the development and maintenance of many physiological functions; and sperm, needed for male fertility. The synthesis of both products is regulated by endocrine hormones produced in the hypothalamus and pituitary, as well as locally within the testis. Testosterone is indispensable for sperm production, however both testosterone and Follicle Stimulating Hormone (FSH) are needed for optimal testicular development and maximal sperm production. Sperm are produced via the extraordinarily complex and dynamic process of spermatogenesis that requires co-operation between multiple testicular cell types. While it has long been known that testosterone and FSH regulate spermatogenesis, years of research has shed light on many of the intricate mechanisms by which spermatogonial stem cells develop into highly specialized, motile spermatozoa. Spermatogenesis involves the concerted interactions of endocrine hormones, but also many paracrine and growth factors, tightly co-ordinated gene and protein expression programs as well as epigenetic modifiers of the genome and different non-coding RNA species. This chapter provides a comprehensive overview of the fascinating process of spermatogenesis and of its regulation, and emphasises the endocrine regulation of testicular somatic cells and germ cells. The chapter also provides a summary of the clinically significant aspects of the endocrine regulation of spermatogenesis. For complete coverage of all related areas of Endocrinology, please see our online FREE web-book, www.endotext.org.
CLINICAL SUMMARY
The testes synthesize two essential products: testosterone, needed for the development and maintenance of many physiological functions including normal testis function; and sperm, needed for male fertility. The synthesis of both products is regulated by endocrine hormones produced in the hypothalamus and pituitary, as well as locally within the testis.
The secretion of hypothalamic gonadotropin-releasing hormone (GnRH) stimulates production of luteinizing hormone (LH) and follicle stimulating hormone (FSH) by the pituitary. LH is transported in the blood stream to the testes, where it stimulates Leydig cells to produce testosterone: this can act as an androgen (via interaction with androgen receptors) but can also be aromatized to produce estrogens. The testes, in turn, feedback on the hypothalamus and the pituitary via testosterone and inhibin secretion, in a negative feedback loop to limit GnRH and gonodotropin production. Both androgens and FSH act on receptors within the supporting somatic cells, the Sertoli cells, to stimulate various functions needed for optimal sperm production. Spermatogenesis is the process by which immature male germ cells divide, undergo meiosis and differentiate into highly specialized haploid spermatozoa. Optimal spermatogenesis requires the action of both testosterone (via androgen receptors) and FSH.
Spermatogenesis takes place within the seminiferous tubules of the testis. These tubules form long convoluted loops that pass into the mediastinum of the testis and join an anastomosing network of tubules called the rete testis. Spermatozoa exit the testes via the rete and enter the efferent ductules prior to their passage through, and final maturation in, the epididymis. The seminiferous tubules are comprised of the seminiferous epithelium: the somatic Sertoli cells and the developing male germ cells at various stages of development. Surrounding the seminiferous epithelium is a layer of basement membrane and layers of modified myofibroblastic cells termed peritubular myoid cells. Between the tubules is the interstitial space that contains blood and lymphatic vessels, immune cells including macrophages and lymphocytes, and the steroidogenic Leydig cells.
Male germ cell development relies absolutely on the structural and nutritional support of the somatic Sertoli cells. Sertoli cells are large columnar cells, with their base residing on basement membrane on the outside of the seminiferous tubules, and their apical processes surrounding germ cells as they develop into spermatozoa. Androgens (and estrogens) and FSH act on receptors within Sertoli cells: germ cells lack both androgen and FSH receptors, therefore these hormones act directly on Sertoli cells to support spermatogenesis. Sertoli cells regulate the internal environment of the seminiferous tubule by secreting paracrine factors and expressing cell surface receptors needed for germ cell development. Sertoli cells form intercellular tight junctions at their base: these occluding junctions prevent the diffusion of substances from the interstitium into the tubules and create a specialized milieu required for germ cell development. These junctions are a major component of the so-called ‘blood-testis-barrier’, wherein the passage of substances from the circulation is prevented from entering the inner part of the seminiferous tubules. The most immature germ cells, including germline stem cells, reside near the basement membrane of the seminiferous tubules and thus have free access to factors from the interstitium, however germ cells undergoing meiosis and haploid cell differentiation develop “above” the blood-testis-barrier and thus are entirely reliant on the Sertoli cell microenvironment. The seminiferous tubules are also an immune-privileged environment. Meiotic and post-meiotic germ cells develop after the establishment of immune tolerance, and could thus be recognized as “foreign” by the immune system, therefore the seminiferous tubules, via a number of different mechanisms including the blood-testis-barrier, actively exclude immune cells and factors from entering the seminiferous tubules and being exposed to meiotic and haploid germ cells.
The number of Sertoli cells determines the ultimate spermatogenic output of the testes. In humans, Sertoli cells proliferate during the fetal and early neonatal period and again prior to puberty. At puberty, Sertoli cells cease proliferation and attain a mature, terminally differentiated phenotype that is able to support spermatogenesis. Disturbances to Sertoli cell proliferation during these times can result in smaller testes with lower sperm production. Conversely, disturbances to the cessation of proliferation can result in larger testes with more Sertoli cells and a greater sperm output. It seems likely that the failure of many men with congenital hypogonadotropic hypogonadism (HH) to achieve normal testicular size and sperm output, when treated by gonadotropic stimulation, may result from deficient Sertoli cell proliferation during fetal and prepubertal life. The action of both androgens and FSH on Sertoli cells is necessary for the ability of Sertoli cells to support full spermatogenesis. In addition, the expression of many genes and paracrine factors within Sertoli cells is necessary for spermatogenesis.
Spermatogenesis relies on the ability of Leydig cells to produce testosterone under the influence of LH. Fetal Leydig cells appear following gonadal sex differentiation (gestational weeks 7-8 in humans) and, under the stimulation of placental human chorionic gonadotropin (hCG), results in the production of testosterone during gestation. In humans, fetal cells decrease in number towards term and are lost from the interstitium at about twelve months of age. The adult population of Leydig cells in the human arises from the division and differentiation of mesenchymal precursor cells under the influence of LH at puberty. Factors secreted by Sertoli cells and peritubular myoid cells are also necessary for Leydig cell development and steroidogenesis. Optimal Leydig cell steroidogenesis also relies on a normal complement of macrophages within the testicular interstitium as well as on the presence of androgen receptors in peritubular myoid cells, presumably because these cells secrete factors necessary for Leydig cell development and function.
The process of spermatogenesis begins in the fetal testis, when the Sertoli cell population is specified in the embryonic testis under the influence of male sex determining factors, such as SRY and SOX9. Newly-specified Sertoli cells enclose and form seminiferous cord structures and direct primordial germ cells to commit to the male pathway of gene expression. Fetal Sertoli cells proliferate and drive seminiferous cord elongation; this process is also dependent on factors secreted by Leydig cells. In the neonatal testis, primordial germ cells undergo further maturation and migrate to the basement of the seminiferous tubules where they provide a pool of precursor germ cells for postnatal spermatogenesis.
Spermatogonia are the most immature germ cell type. This heterogeneous population includes spermatogonial stem cells, which self-renew throughout life to provide a pool of stem cells available for spermatogenesis, as well as proliferating cells that differentiate and become committed to entry into meiosis. Spermatogonial development is hormonally independent and as such they are present even in the absence of GnRH. Spermatogonia eventually differentiate into spermatocytes that proceed through the process of meiosis that begins with DNA synthesis resulting in a tetraploid gamete. During the long meiotic prophase, which lasts ~2 weeks, homologous chromosomes pair and meiotic recombination occurs; this involves the induction and repair of DNA double-strand breaks allowing the exchange of genetic information between paired chromosomes, thereby creating genetic diversity between gametes. At the end of prophase, the meiotic cells proceed through two rapid and successive reductive divisions to yield haploid spermatids. The completion of meiosis depends absolutely on androgen action in Sertoli cells; in the absence of androgen, no haploid spermatids will be produced.
Newly formed haploid round spermatids differentiate, with no further division, into the highly specialized spermatozoan during the process of spermiogenesis. This involves many complex processes, including development of the acrosome (an organelle on the surface of the sperm head that contains enzymes required to penetrate the zona pellucida of the oocyte and thus facilitate fertilization), the flagella (the motile microtubule-based structure required for sperm motility) and the remodelling of the spermatid’s DNA into a tightly coiled structure within a small, streamlined nucleus that will not hinder motility. This remodelling of the DNA involves the cessation of gene transcription up to 2 weeks prior to the final maturation of the sperm; therefore spermiogenesis involves the translational delay of many mRNA species which must then be translated at precise times throughout their final development. Spermatogenesis ends with the process of spermiation. This involves removal of the spermatid’s large cytoplasm, revealing the streamlined mature spermatozoa, and the final disengagement of sperm from the Sertoli cells into the tubule lumen, prior to their passage to the epididymis. Both the survival of spermatids during spermiogenesis and their release at the end of spermiation is dependent on optimal levels of androgen and FSH.
Spermatogenesis is a long process, taking up to 64 days in the human, and its inherent complexity demands precise timing and spatial organization. Within the seminiferous tubules, Sertoli cells and surrounding germ cells in various phases of development are highly organized into a series of cell associations, known as stages. These stages result from the fact that a particular spermatogonial cell type, when it appears in the epithelium, is always associated with a specific stage of meiosis and spermatid development. The stages follow one another along the length of the seminiferous tubule, and the completion of a series of stages is termed a “cycle”. This cycle along the length of tubule is obvious in rodents, however in humans several cycles are intertwined in a helical pattern; thus a human seminiferous tubule viewed in cross section will contain up to three stages. The completion of one cycle results in the release of mature spermatozoa into the tubule lumen; the cycles are repeated along the tubules, resulting in constant “pulses” of sperm production. These pulses of sperm release allow the testes to continually produce millions of sperm, with the average normospermic man able to produce approximately 1000 sperm per heartbeat.
The precise timing and co-ordination of spermatogenesis is achieved by many factors. Emerging evidence suggests that retinoic acid, metabolized within the testis from circulating retinol (a product of vitamin A) is a major driver of spermatogenesis. A precise pulse of retinoic acid action is delivered to a particular stage of the spermatogenic cycle; this pulse is achieved by the constrained expression of enzymes involved in retinoic acid synthesis, degradation and storage, as well as the local expression of retinoic acid receptors. This pulse of retinoic acid acts directly on spermatogonia to stimulate their entry into the pathway committed to meiosis. It also acts directly on Sertoli cells to regulate its cyclic functions. Sertoli cells contain an internal “clock” that allows them to express genes and proteins at precise times. This clock appears to be set by retinoic acid, however the timing of the clock can be influenced by the germ cells themselves.
The timing of spermatogenesis also relies on an extraordinarily complex program of gene transcription and protein translation. Alternative splicing of mRNA is highly prevalent in the testis, and generates many germ cell-specific transcripts that are important for the ordered procession of germ cell development. Noncoding RNAs, including microRNAs, small interfering RNAs, piRNAs and long noncoding RNAs, are highly expressed in the testis, particularly by the germ cells. Indeed, studies on male germ cells have revealed much of what is known about the biology and function of non-coding RNAs. These non-coding RNAs have many and varied roles and are particularly required for the transcriptional program executed during meiosis and spermiogenesis.
The male germ cell transmits both genetic and epigenetic information to the offspring. Epigenetic modifications of the genome are heritable; epigenetic processes such as DNA methylation and histone modifications regulate chromatin structure and modulate gene transcription and silencing. The male germ cell undergoes major epigenetic programming in the fetal testis, during the genome wide de-methylation and re-methylation to establish the germline-specific epigenetic pattern that is eventually transmitted to the offspring. The sperm epigenome is then further remodelled during postnatal spermatogenesis by various mechanisms. It is now known that a man’s sperm epigenome can be altered by environmental factors (including diet and lifestyle as well as exposure to environmental factors) throughout his lifetime and this altered sperm epigenome can influence both his fertility and the health of his future children.
It is clear from the above summary that spermatogenesis relies on many intrinsic and extrinsic factors. However spermatogenesis is absolutely dependent on androgen-secretion by the Leydig cells; androgens stimulate and maintain germ cell development throughout life. Testicular testosterone levels are very high, by virtue of its local production, however they are considerably higher than those required for the initiation and maintenance of spermatogenesis. Androgen action on receptors within Leydig cells, peritubular myoid cells and Sertoli cells is essential for normal steroidogenesis and spermatogenesis. While testosterone is essential for spermatogenesis, it is also important to note that exogenous testosterone administration resulting in even slightly supraphysiological serum levels suppresses gonadotropin secretion via negative feedback effects on the hypothalamus and pituitary, leading to the cessation of sperm production.
In contrast to androgens, spermatogenesis can proceed in the absence of FSH; however, testes are smaller and sperm output is reduced. This is due to FSH’s role in the peri-pubertal proliferation and differentiation of Sertoli cells and in the maintenance of germ cell survival. While FSH is thus not essential for spermatogenesis, it is generally considered that optimal spermatogenesis requires the combined actions of both androgen and FSH, with both hormones having independent, co-operative and synergistic effects to promote maximal sperm output.
These factors are an important consideration in the stimulation of spermatogenesis in the setting of HH. As androgens are essential for the initiation of sperm production, the induction of spermatogenesis in HH acquired after puberty is achieved by the administration of hCG (as an LH substitute). Prolonged therapy is required to produce sperm in the ejaculate, given that human spermatogenesis takes more than 2 months to produce sperm from spermatogonia. Treatment with hCG alone may be sufficient for the induction of spermatogenesis in men with larger testes due to potential residual FSH action, however, for many men, and particularly for those with congenital HH, the co-administration of FSH is needed for maximal stimulation of sperm output. In men with congenital HH, FSH is needed to induce Sertoli cell maturation, whereas men with acquired HH and smaller testes benefit from the co-administration of FSH due to the synergistic actions of FSH and androgens on spermatogenesis.
In summary, the testes, under the influence of gonadotropins, produce testosterone and sperm. These processes require the co-ordinated actions of multiple cell types and the secretion of paracrine factors. Spermatogenesis is a long and complex process that relies on multiple somatic cells as well as on the co-ordinated expression of genes, proteins and non-coding RNAs. Inherent vulnerabilities exist in spermatogenesis meaning that lifestyle and environmental factors can potentially influence a man’s sperm epigenome, his fertility and the health of his future children.
GENERAL ANATOMY OF THE MALE REPRODUCTIVE SYSTEM
The Testis
The testis lies within the scrotum and is covered on all surfaces, except its posterior border, by a serous membrane called the tunica vaginalis. This structure forms a closed cavity representing the remnants of the processus vaginalis into which the testis descends during fetal development (Figure 1). Along its posterior border, the testis is loosely linked to the epididymis which at its lower pole gives rise to the vas deferens (1).

Figure 1
The relationships of the tunica vaginalis to the testis and epididymis is illustrated from the lateral view and two cross sections at the level of the head and mid-body of the epididymis. The large arrows indicate the sinus of the epididymis posteriorly. Reproduced with permission from de Kretser et.al.1982 in 'Disturbances in Male Fertility' Eds K Bandhauer and J Frick, Springer - Verlag Berlin.
The testis is covered by a thick fibrous connective tissue capsule called the tunica albuginea. From this structure, thin imperfect septa run in a posterior direction to join a fibrous thickening of the posterior part of the tunica albuginea called the mediastinum of the testis. The testis is thus incompletely divided into a series of lobules.
Within these lobules, the seminiferous tubules form loops, the terminal ends of which extend as straight tubular extensions, called tubuli recti, which pass into the mediastinum of the testis and join an anastomosing network of tubules called the rete testis. From the rete testis, in the human, a series of six to twelve fine efferent ducts join to form the duct of the epididymis. This duct, approximately 5-6m long in the human, is extensively coiled and forms the structure of the epididymis that can be divided into the head, body and tail of the epididymis (1). At its distal pole, the tail of the epididymis gives rise to the vas deferens (Figure 2).
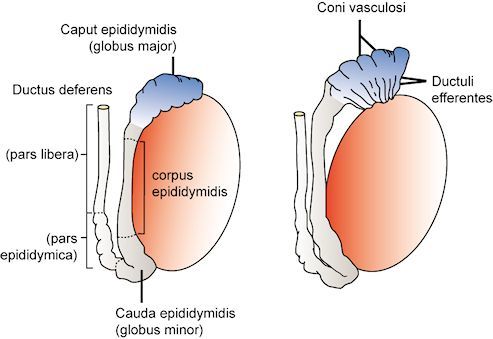
Figure 2
The arrangement of the efferent ducts and the subdivisions of the epididymis and vas are shown. Reproduced with permission from de Kretser et.al.1982 in 'Disturbances in Male Fertility' Eds K Bandhauer and J Frick, Springer - Verlag Berlin.
The arterial supply to the testis arises at the level of the second lumbar vertebra from the aorta on the right and the renal artery on the left and these vessels descend retroperitoneally to descend through the inguinal canal forming part of the spermatic cord. The testicular artery enters the testis on its posterior surface, sending a network of branches that run deep to the tunica albuginea before entering the substance of the testis (2). The venous drainage passes posteriorly and emerges at the upper pole of the testis as a plexus of veins termed the pampiniform plexus (Figure 3). As these veins ascend they surround the testicular artery, forming the basis of a countercurrent heat exchange system which assists in the maintenance of a temperature differential between the scrotally placed testis and the intra-abdominal temperature (3).

Figure 3
The arrangement of the vasculature of the testis in the region of the distal spermatic cord and testis is shown. Reproduced with permission from de Kretser et.al.1982 in 'Disturbances in Male Fertility' Eds K Bandhauer and J Frick, Springer - Verlag Berlin.
The Distal Reproductive Tract
The vas deferens ascends from the testis on its posterior surface as a component of the spermatic cord passing through the inguinal canal and descends on the posterolateral wall of the pelvis to reach the posterior aspect of the bladder where its distal end is dilated forming the ampulla of the vas (Figure 4). At this site it is joined by the duct of the seminal vesicle, on each side, to form an ejaculatory duct that passes through the substance of the prostate to enter the prostatic urethra. The seminal vesicles and the prostate, the latter of which opens by a series of small ducts into the prostatic urethra, contribute approximately 90-95% of the volume of the ejaculate. During the process of ejaculation, these contents, together with sperm transported through the vas, are discharged through the prostatic and penile urethra. Retrograde ejaculation is prevented by contraction of the internal sphincter of the bladder during ejaculation. Failure of this sphincter to contract results in retrograde ejaculation and a low semen volume.
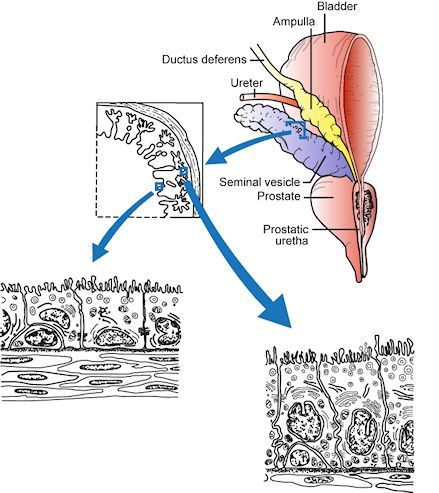
Figure 4
The diagram depicts the relationship between the vas deferens, the seminal vesicles, the posterior aspect of the bladder and the prostate gland. The cytological features of the epithelium of the seminal vesicles is shown: this tissue is androgen dependent. Reproduced with permission from de Kretser et.al.1982 in 'Disturbances in Male Fertility' Eds K Bandhauer and J Frick, Springer - Verlag Berlin.
AN OVERVIEW OF SPERMATOGENESIS
Spermatogenesis is the process by which precursor germ cells termed spermatogonia undergo a complex series of divisions to give rise to spermatozoa (4-5). This process takes place within the seminiferous epithelium (Figure 5), a complex structure composed of germ cells and radially-oriented supporting somatic cells called Sertoli cells. The latter cells extend from the basement membrane of the seminiferous tubules to reach the lumen. The cytoplasmic profiles of the Sertoli cells are extremely complex as this cell extends a series of processes that surround the adjacent germ cells in an arboreal pattern (5-7).

Figure 5
The top panel illustrates the typical structure of the human seminiferous epithelium containing the germ cells and Sertoli cells. The position of Sertoli cell nuclei within the epithelium is indicated, as is the tubule lumen. The tubules are surrounded by thin plate-like contractile cells called peritubular myoid cells. The Leydig cells and blood vessels lie within the interstitium. The bottom panel illustrates the nuclear morphology of the major cell types found within the human seminiferous epithelium, showing the progress of spermatogenesis from immature spermatogonia through meiosis and spermiogenesis to produce mature elongated spermatids. Abbreviations: Ad: A dark spermatogonia, Ap: A pale spermatogonia, B: type B spermatogonia, Pl: preleptotene spermatocyte, L-Z: leptotene to zygotene spermatocyte, PS: pachytene spermatocyte, M: meiotic division, rST: round spermatid, elST: elongating spermatid, eST: elongated spermatid. All germ cell micrographs were taken at the same magnification to indicate relative size. Micrograph of seminiferous epithelium was provided by Dr Sarah Meachem.
Spermatogenesis can be divided into three major phases (i) proliferation and differentiation of spermatogonia, (ii) meiosis, and (iii) spermiogenesis which represents a complex metamorphosis of round haploid germ cells into the highly specialized structure of the spermatozoon (Figure 5). It is important to note that, as germ cells divide and differentiate through these phases, they do not separate completely after mitosis but remain joined by intercellular bridges (8). These intercellular bridges persist throughout all stages of spermatogenesis and are thought to facilitate biochemical interactions allowing synchrony of germ cell maturation.
Spermatogonial Renewal and Differentiation
Spermatogonia are precursor male germ cells that reside near the basement membrane of the seminiferous epithelium. Spermatogonial stem cells (SSC) divide to renew the stem cell population and to provide spermatogonia that are committed to the spermatogenic differentiation pathway. Adult mouse and human SSC are pluripotent, and have the ability to differentiate into derivatives of all three germ layers (9-10).
In general, two main types of spermatogonia, known as Type A and B, can identified in mammalian testes on the basis of nuclear morphology (5). Type A spermatogonia exhibit fine pale-staining nuclear chromatin and are considered to include the SSC pool, the undifferentiated spermatogonia (Aundiff) pool, and spermatogonia which have become committed to differentiation (Adiff). The Aundiff pool is comprised of the SSC, single A spermatogonia (As), and interconnected cysts of either 2 (known as A paired, or Apr) or more (aligned or Aal) undifferentiated spermatogonia that remain connected by intercellular bridges. Once per cycle (see section below), the Aundiff cells transform into Adiff cells, which are then designated A1, A2, etc. Adiff spermatogonia ultimately divide to produce type B spermatogonia. Type B spermatogonia show coarse chromatin collections close to the nuclear membrane (11) and represent the more differentiated spermatogonia that are committed to entry into meiosis (12).
Recent studies have focused on dissecting the molecular properties of the various A spermatogonial subtypes in an effort to identify the SSC population of the testis. Studies have also investigated their clonal behavior as they divide and differentiate. The pioneering technique of spermatogonial transplantation (13-16) is used to determine the regenerative capacity of a cell population and to define subtypes with SSC potential.
The current, widely-accepted model of Type A spermatogonial division and differentiation includes the concept of As representing the least differentiated spermatogonial population. Within this population, some As cells express the ID4 protein and have both regenerative and self-renewal properties, suggesting these are the true stem cells of the adult testis (17-18). As can divide completely to renew their population, or divide incompletely to produce Apr cells, which represents an initial step towards differentiation. The Apr cells subsequently divide to produce Aal cells which then divide to produce chains (or cysts) of more differentiated spermatogonia, termed Aal4-16. As the A spermatogonia subtypes progress through these steps, there are changes in their molecular signature and the expression of cell surface markers, likely reflecting their differentiation state and functional capabilities, see (19).
Recent in vivo imaging studies of fluorescently-tagged A spermatogonial subtypes challenge some aspects of the current model (20-21). These studies suggest that there may be more fluidity in the transition between undifferentiated A spermatogonial subtypes (i.e. As, Apr, Aal), and in their ability to attain SSC characteristics (20-21). In vivo imaging and pulse labeling studies suggest that fragmentation of spermatogonial cysts (e.g fragmentation of Apr or Aal clones) to produce As is a commonly observed phenomenon, and biophysical modeling studies suggest that fragmentation of Apr and Aal clones may be an important source of As that can then exhibit SSC behavior (20). Thus there may be a less linear relationship between As→Apr→Aal, and more flexibility as they fragment and transition back and forth between subtypes. Clone fragmentation appears likely to be an important aspect of steady state spermatogonial kinetics, as well as during the repopulation of the testis following an insult to spermatogenesis, such as via radiation or chemotherapeutic agents (20).
In humans and other primates, the Type A spermatogonia can only be classified into two subtypes; A dark (Ad) and A pale (Ap) spermatogonia (12). Some investigators have proposed that the Ad spermatogonia are similar to As in the rodent, and thus represent the SSC or reserve spermatogonial population (22-24) whereas others have suggested that the Ap spermatogonia are the true stem cell of the testis (25). More recent studies suggest that Ap spermatogonia also show characteristics of As spermatogonia in rodents, reviewed in (26), however it remains unclear how primate Type A spermatogonial subtypes relate to those in rodents. In primates, both Ap and Ad spermatogonia express GFRα (27), a marker of Aundiff in rodents, reviewed in (19). Like rodent Aundiff spermatogonia, there are heterogeneous subpopulations within GFRα1+ human Ap spermatogonia (28). Differentiation of A spermatogonia in monkeys is associated with the cytoplasmic to nuclear translocation of the transcription factor SHLH1 (27). Further studies on markers of rodent spermatogonial subtypes, including SSC, and their analysis in primate and human testes will inform our understanding of human spermatogonial biology (26).
Meiosis
Meiosis is the process by which gametes undergo reductive division to provide a haploid spermatid, and in which genetic diversity of the gamete is assured via the exchange of genetic material. During meiosis I, DNA synthesis is initiated, resulting in a tetraploid gamete. The exchange of genetic information is achieved during meiotic recombination, which involves the induction of DNA double-strand breaks (DSBs) during pairing of homologous chromosomes and the subsequent repair of DSBs using homologous chromosomes as templates. Once exchange of genetic material is complete, the cells proceed through two successive reductive divisions to yield haploid spermatids. This process is governed by genetically programmed checkpoint systems.
Meiosis commences when Type B spermatogonia lose their contact with the basement membrane and form preleptotene primary spermatocytes. The preleptotene primary spermatocytes commence DNA synthesis and the condensation of individual chromosomes begins, resulting in the appearance of thin filaments in the nucleus which identify the leptotene stage (29). At this stage, each chromosome consists of a pair of chromatids (Figure 6). As the cells move into the zygotene stage, there is further thickening of these chromatids and the pairing of homologous chromosomes. The further enlargement of the nucleus and condensation of the pairs of homologous chromosomes, termed bivalents, provides the nuclear characteristics of the pachytene stage primary spermatocyte. During this stage, there is exchange of genetic material between homologous chromosomes derived from maternal and paternal sources, thus ensuring genetic diversity of the gametes. The sites of exchange of genetic material are marked by the appearance of chiasmata and these become visible when the homologous chromosomes separate slightly during diplotene. The exchange of genetic material involves DNA strand breakage and subsequent repair (30).

Figure 6
The diagrammatic representation of the events occurring between homologous chromosomes during the prophase of the first meiotic division shows the period of DNA synthesis, the formation of the synaptonemal complex and the processes involved in recombination. Reproduced with permission from de Kretser and Kerr (1994) in "The Physiology of Reproduction" Ed E Knobil & J D Neill, Lippincott, Williams & Wilkins.
The diplotene stage is recognized by partial separation of the homologous pairs of chromosomes that still remain joined at their chiasmata and each is still composed of a pair of chromatids. With dissolution of the nuclear membrane, the chromosomes align on a spindle and each member of the homologous pair moves to opposite poles of the spindle during anaphase. The resultant daughter cells are called secondary spermatocytes and contain the haploid number of chromosomes but, since each chromosome is composed of a pair of chromatids, the DNA content is still diploid. After a short interphase, which in the human represents approximately six hours, the secondary spermatocytes commence a second meiotic division during which the chromatids of each chromosome move to opposite poles of the spindle forming daughter cells that are known as round spermatids (12, 31). Meiotic maturation in the human takes about 24 days to proceed from the preleptotene stage to the formation of round spermatids.
It is well known that advancing maternal age is associated with increased meiotic errors leading to reduced gamete quality, however whether this phenomenon occurs in males has been the subject of debate. A recent study in mice showed that advanced age was associated with increased defects in chromosome pairing, however no increase in anueploidy was observed at Metaphase II, suggesting that such errors were corrected during metaphase checkpoints in males (32). Therefore advanced age, at least in mice, has more of an impact on gamete aneuploidy in females compared to males.
Spermiogenesis and Spermiation
The transformation of a round spermatid into a spermatozoon represents a complex sequence of events that constitute the process of spermiogenesis. No cell division occurs, but a conventional round cell becomes converted into a spermatozoon with the capacity for motility. The basic steps in this process (Figure 7) are consistent between all species and consist of (a) the formation of the acrosome (b) nuclear changes (c) the development of the flagellum or sperm tail (d) the reorganisation of the cytoplasm and cell organelles and (e) the process of release from the Sertoli cell termed spermiation (5, 33-37).

Figure 7
The changes during spermiogenesis involving the transformation of a round spermatid to a mature spermatozoon are shown. Redrawn with permission from de Kretser and Kerr (1994) in "The Physiology of Reproduction" Ed E Knobil & J D Neill, Lippincott, Williams & Wilkins.
The formation of the acrosome commences by the coalescence of a series of granules from the Golgi complex. These migrate to come into contact with the nuclear membrane where they form a cap-like structure which becomes applied over approximately 30-50% of the nuclear surface (33). Acrosome biogenesis begins early in round spermatid development, and progressively extends as a “cap” over the nucleus as round spermatids differentiate further.
Once the acrosome is fully extended, round spermatids begin what is known as the elongation phase of spermiogenesis. As spermatid elongation commences, the nucleus polarizes to one side of the cell (Figure 7) and comes into close apposition with the cell membrane in a region where it is covered by the acrosomal cap. Soon after this polarization, the spermatid’s chromatin starts to visibly condense, forming progressively larger and more electron dense granules together with a change in the shape of the condensed nucleus. This change in nuclear shape varies significantly between species. The condensation of chromatin is achieved by the replacement of lysine-rich histones with transitional proteins which in turn are subsequently replaced by arginine-rich protamines (38-39). The spermatid chromatin subsequently becomes highly stabilized and resistant to digestion by the enzyme DNAse. Associated with these changes is a marked decrease in nuclear volume and, importantly, the cessation of gene transcription (40). Therefore, the subsequent spermatid elongation phase proceeds in the absence of active gene transcription (see (36)).
At the commencement of spermatid elongation, a complex, microtubule-based structure known as the manchette is formed. The microtubule network emanates from a perinuclear ring at the base of the acrosome and extends outwards into the cytoplasm. The manchette is closely opposed to the nuclear membrane, and is thought to participate in nuclear head shaping, perhaps by exerting a force on the nucleus as it progressively moves distally towards the posterior portion of the nucleus (41-43).
The formation of the tail commences early in spermiogenesis in the round spermatid phase, when a filamentous structure emerges from one of the pair of centrioles which lie close to the Golgi complex. Associated with the changing nuclear-cytoplasmic relationships, the developing flagellum and the pair of centrioles become lodged in a fossa in the nucleus at the opposite pole to the acrosome. The central core of the flagella’s axial filament, called the axoneme, consists of nine doublet microtubules surrounding two single central microtubules, which represents a common pattern found in cilia. This basic structure is modified at the region of its articulation with the nucleus through the formation of a complex structure known as the connecting piece (44).
Metamorphosis of the flagella proceeds during the elongation phase, as it acquires its characteristic neck region, mid-, principal- and end-pieces (37). The development of the flagella is thought to involve a mechanism known as Intra-Manchette Transport (IMT), which is proposed to be similar to the Intra-Flagellar Transport (IFT) systems used in other ciliated cells. IMT involves proteins being “shuttled” from the spermatid nucleus down to the developing flagellum via molecular motors travelling along “tracks” of microtubules and filamentous actin (42-43, 45).
The middle and principal pieces contain the mitochondrial and fibrous sheath components, respectively, and include the outer dense fibers. The biochemical characteristics of these components of the sperm tail are emerging (46-51), reviewed in (37). While these components provide some structural stability to the tail, evidence suggests that they may serve as a molecular scaffold to position key enzymes critical to successful sperm motility. For instance, CatSper 1, an ion channel plasma membrane-associated protein present in the principal-piece, has been shown to regulate calcium ion fluxes critical for the process of hyperactivation of sperm motility associated with capacitation (52). Studies demonstrate that CatSper, or a directly associated protein, is a non-genomic progesterone receptor that mediates the effects of progesterone on sperm hyperactivation and acrosome reaction (53-54). Further studies have shown that plasma membrane calcium-ATPase 1 is also located in the principal-piece and has been shown to be critical for the process of hyperactivation of sperm motility (55). While these are plasma membrane-located complexes, TPX1 (also called CRISP2), a protein localized to the outer dense fibers of the tail and acrosome (56) has been shown to regulate ryanodine receptor calcium signalling (57).
The formation of the mitochondrial sheath occurs at the time of the final reorganization of the cytoplasm and organelles of the spermatid (5, 33, 58). The mitochondria that had remained around the periphery of the spermatid aggregate around the proximal part of the flagellum to form a complex helical structure (Figure 8).
The mature elongated spermatids undergo a further complex remodeling during spermiation, the process by which the mature spermatids are remodeled and then released from the Sertoli cells prior to their passage to the epididymis, see (35) for review. This remodeling includes the removal of specialized adhesion junctions that have ensured tight adhesion of the spermatid to the Sertoli cell during its elongation process, further remodeling of the spermatid head and acrosome and removal of the extensive cytoplasm to produce the streamlined spermatozoon. The cytoplasm of the spermatid migrates to a caudal position around the tail and is markedly reduced in volume. Some observations suggest that prolongations of Sertoli cell cytoplasm send finger-like projections which invaginate the cell membrane of the spermatid cytoplasm and literally 'pull' the residual cytoplasm off the spermatid (33). The remnants of the spermatid cytoplasm form what is termed the residual body. The residual bodies contain mitochondria, lipid and ribosomal particles, and are phagocytosed and moved to the base of the Sertoli cell where they are broken down by lysosomal mechanisms. The final release of sperm at the end of spermiation is an instantaneous event, and likely involves phosphorylation-dependent signaling cascades within the Sertoli cell resulting in changes in the adhesive nature of cell adhesion molecules (35), culminating in the Sertoli cell “letting go” of the mature spermatid (59). The morphological features of spermiation are relatively conserved between species, particularly among mammals (60). Spermiation is highly susceptible to perturbation by pharmacological modulators and by agents that suppress gonadotropins, reviewed in (35), and failure of spermiation can be recognized by the presence of mature elongated spermatid nuclei being phagocytosed by the Sertoli cells (61).

Figure 8. A
cross-section through the developing mid-piece of the sperm tail shows the aggregation of mitochondria (arrows) surrounding the outer dense fibres (labelled 1-9) which in turn surround the axoneme composed of 9 doublet microtubules surrounding two central microtubules. Reproduced with permission from "Visual atlas of human sperm structure and function for assisted reproductive technology" Ed A.H. Sathanathan 1996.
The Cycle of the Seminiferous Epithelium
Within the seminiferous epithelium, the cell types that constitute the process of spermatogenesis are highly organized to form a series of cell associations or stages. These cell associations, or stages of spermatogenesis, result from the fact that a particular spermatogonial cell type, when it appears in the epithelium, is always associated with a specific stage of meiosis and spermatid development. The stages follow one another along the length of the seminiferous tubule, and the completion of a series of stages is termed a “cycle” (see Figure 9). This cycle along the length of tubule is obvious in rodents, however in humans the situation is more complex (see below). The completion of one cycle results in the release of mature spermatozoa into the tubule lumen; the cycles are repeated along the length of the tubules (Figure 9), resulting in constant “pulses” of sperm production along the tubules. Thus the cyclic nature of spermatogenesis enables continual sperm production within the testis. These pulses of sperm release along the length of the seminiferous tubules allow the testes to continually produce millions of sperm, with the average normospermic man able to produce approximately 1000 sperm per heartbeat.
The cycle of the seminiferous epithelium was defined by LeBlond and Clermont (62), as the series of changes in a given area of the seminiferous tubule between two appearances of the same developmental stage or cell association. They defined 14 stages in the rat cycle based on the 19 phases of spermiogenesis (Figure 9) as identified by the periodic acid Schiff (PAS) stain. In effect, if it was possible to observe the same region of the seminiferous epithelium by phase contrast microscopy over time, the appearance would progress through the 14 stages before stage I reappeared. They also demonstrated that the duration of any one stage was proportional to the frequency with which it was observed in the testis. As type A spermatogonia in any one area of the epithelium progress through meiosis and spermiogenesis to become spermatozoa, the specific area of the tubule would pass through the 14 stages four times. In each progression, the progeny of the spermatogonia progressively move toward the lumen of the tubule.

Figure 9
The top panel shows a diagrammatic representation of the stages of the seminiferous cycle in the rat and shows the types of germ cell associations which form the stages. The stage is denoted by roman numerals. These stages follow one another in a cyclic manner along the length of the seminiferous tubule, as illustrated in the diagram in the middle panel. Examples of the histology of the seminiferous epithelium at two different stages are given in the bottom panel.
Studies in many mammalian species demonstrated that the cycle of spermatogenesis could be identified for each species but showed that the duration of the cycle varied for each species (12). In many species, especially the rat, the same stage of spermatogenesis extends over several millimetres of the adjacent tubule and it is possible, by observation under transillumination, to dissect lengths of seminiferous tubules at the same phase of spermatogenesis (63). Such observations amply confirmed the earlier studies of Perey and colleagues (64), that the stages of spermatogenesis were sequentially arranged along the length of the tubule (Figure 9). As the cycle progress, this arrangement resulted in a "wave of spermatogenesis" along the tubule. Regaud (65) interpreted his observations correctly by the statement "the wave is in space what the cycle is in time".
For many years, investigators believed that such a cycle did not occur in the human testis but the careful studies of Clermont (66) showed that human spermatogenesis could be subdivided into 6 stages. However unlike the rat, each stage often only occupied one quadrant of any given tubule cross section giving the disorganized appearance. By careful studies using tritiated thymidine injections into the testis, Clermont and Heller (31) demonstrated that the duration of the cycle in the human took 16 days and the progression from spermatogonia to sperm took 70 days or four and a half cycles of the seminiferous cycle. Other studies showed that the cycle length was specific for each species (eg rat 49 days) and the progression of each cell type in spermatogenesis involved a defined duration (12). It is likely that the relatively poor definition of stages in human seminiferous tubules, compared to the rat, is due to a greater number of spermatogonia entering each phase of the cycle in the rat, their cell progeny therefore occupying a greater length of the tubule.
Transcriptional profiling studies described the changing patterns of gene expression across the rat spermatogenic cycle, and demonstrated that Sertoli cells and germ cells showed highly co-ordinated stage-dependent changes in gene expression (67). The mechanisms underlying these temporal constraints on spermatogenesis have been the subject of speculation as to whether these were intrinsic or were imposed by the Sertoli cells. The latter proposition is supported by the demonstration that when rat germ cells were transplanted into the mouse testis, spermatogenesis proceeded at the normal rate for the rat, indicating that the kinetics of the spermatogenic cycle are determined by intrinsic mechanisms within germ cells (68). In contrast however, Sertoli cells demonstrate cyclic expression of certain proteins in the embryonic and pre-pubertal period, even in the absence of germ cells (69). Recent studies demonstrate that retinoic acid “sets the clock” within post-pubertal Sertoli cells, however differentiating germ cells are required to “fine tune” the clock (70) (see below for further information). Taken together, these observations demonstrate that the Sertoli cell contains a “clock” that modulates cyclic gene and protein expression, and that the precise timing of this clock is modulated by germ cells.
THE ROLE OF SERTOLI CELLS IN SPERMATOGENESIS
Sertoli cells have an intimate physical relationship with the germ cells (Figure 10) during the process of spermatogenesis (5, 7, 71). The cytoplasmic extensions that pass between the germ cell populations surrounding the Sertoli cell provides structural support through a microfilament and microtubular network present in the cytoplasm of the Sertoli cell (72). This architecture is not static but changes in the tubule depending on the stage of the spermatogenic process.
Sertoli cells regulate the internal environment of the seminiferous tubule. This regulation is facilitated by specialized inter-Sertoli cell occluding-type junctions which are formed at the sites where processes of Sertoli cell cytoplasm from adjacent cells meet (73). These junctions contribute to the blood-testis barrier that regulates the entry of a variety of substances into the seminiferous tubule (74). These occluding junctions towards the base of Sertoli cells prevent the diffusion of substances from the interstitium into the inner part of the seminiferous tubule (see Figure 11). Because of the location of the junctions, spermatogonia have free access to substances from the interstitium (including the vasculature), however the germ cells “above” this junction, including meiotic and post-meiotic germ cells, have their access to factors from the interstitium restricted by the blood-testis-barrier. This effectively divides the seminiferous epithelium into a basal compartment containing spermatogonia, and an adluminal compartment containing meiotic and post-meiotic germ cells. As preleptotene spermatocytes migrate from the basement membrane of the tubule into the adluminal compartment, these tight junctions open up to allow this cellular migration to take place (Figure 11) and reform beneath the preleptotene spermatocytes which have now left the basement membrane to form leptotene spermatocytes. The formation and dissolution of these junctional specializations are under the control of numerous physiological regulators including endocrine (75-76) and paracrine (77) factors, see for (78) recent review.
The Sertoli cell junctions and the blood-testis barrier are required for fertility (79). These junctions allow the environment of meiotic and post-meiotic germ cells to be precisely controlled by the Sertoli cell, enabling the precisely timed delivery of factors uniquely required for germ cell development. For example, the Sertoli cell provides substrates for germ cell glycolysis (80-82); lactate rather than glucose is the preferred substrate for glycolysis in primary spermatocytes and Sertoli cells generate lactate from glucose.
The blood-testis barrier has long been thought to contribute to the immune-privileged environment within the seminiferous epithelium. Meiotic and post-meiotic germ cells develop after the establishment of immune tolerance, and could thus be recognized as “foreign” by the immune system, therefore this barrier protects the developing germ cells from immune cell attack (83). However some studies show that seminiferous tubules continue to exclude immune cells when Sertoli cell junctions are absent (79) or even when Sertoli cells are ablated (84), raising questions as to the precise role of these junctions in immune privilege. It seems likely that many factors, including the production of anti-inflammatory cytokines, regulate the immune privileged environment of the testis.
In the adult rat testis, activin A protein peaks at the time of blood-testis-barrier remodeling and migration of leptotene spermatocytes into the adluminal compartment, suggesting that activin A could regulate blood-testis barrier function (for review see (85)). More recently it has been shown that elevated activin A action in vivo and in vitro suppresses the Sertoli cell tight junctions that form a major component of the blood-testis barrier (86), suggesting that activin A could facilitate blood-testis-barrier remodeling.
Recent studies have revealed that the blood-testis-barrier shows differential permeability and can exclude different sized molecules depending on its functional status (87). Tracer studies showed that the barrier can exclude all molecules between 0.6-150kDa in size when it is “fully sealed”, however in some situations and stages it can exclude large (150kDa+ molecules) but remain permeable to smaller molecules. These studies reveal that the barrier is more selective in its function than previously thought, and highlight the complexity of this structure and its important role in spermatogenesis.
Sertoli cells are indispensible for germ cell development, as they provide physical, metabolic and nutritional support at precisely timed intervals as dictated by the spermatogenic process. Transgenic mouse models have revealed many Sertoli cell genes that are required for all aspects of spermatogenesis, reviewed in (88). For example, the Etv5 transcription factor within Sertoli cells is essential for the maintenance of the stem cell niche (89), reviewed in (90). Sertoli cells respond to the changing needs of the developing germ cells as evidenced by the remarkable stage-specificity in the expression patterns of many Sertoli cell genes (67).
The differentiation status of Sertoli cells is related to their capacity to support spermatogenesis. For example, perinatal hypothyroidism extends the duration of Sertoli cell proliferation but also delays their maturation; this is also associated with a delay in the onset of spermatogenesis (91-92). It was widely believed that once Sertoli cells ceased pre-pubertal proliferation, they attained a so-called “terminally differentiated” phenotype. However it is now clear that Sertoli cells can de-differentiate in certain conditions of impaired spermatogenesis, reviewed in (93). For example, a loss of claudin 11 (a protein involved in Sertoli cell occluding junctions) causes Sertoli cells to remain proliferative during development and to lose their epithelial phenotype (94). De-differentiated Sertoli cells in cell cycle are not observed in normospermic men, but are present in men after 12 weeks of gonadotropin suppression (95). Intriguingly, adult Sertoli cells can even trans-differentiate into granulosa cells in the absence of the Sertoli cell transcription factor Dmrt1; this activates Foxl2-mediated female somatic cell programming (96). Therefore the maintenance of an adult Sertoli cell phenotype is essential for normal spermatogenesis.
While it has long been known that a healthy Sertoli cell is required for germ cell development, it is now clear that Sertoli cells support the development and function of other testicular cells. Recent studies using a mouse model of acute and specific ablation of Sertoli cells have revealed they are essential for the maintenance of peritubular myoid cell fate and function, and are required for Leydig cell development and normal steroidogenesis (84, 97). Therefore Sertoli cells are required for both sperm and androgen production within the testis.

Figure 10
The general architecture of the Sertoli cell is shown. Note the thin cytoplasmic processes that extend between the germ cells. The Sertoli cell is in contact with a variety of germ cells and adjacent Sertoli cells when three dimensional perspectives are considered.

Figure 11
The position of the blood testis barrier in the seminiferous epithelium, which is formed by tight, occluding and adhesion junctions between adjacent Sertoli cells. This barrier restricts the diffusion of substances from the interstitum and blood vessels, and thus allows the Sertoli cell to determine the microenvironment above the junctions. This barrier effectively divides the seminiferous epithelium into two compartments, the basal compartment with free access to substances from outside the tubule, and the adluminal compartment, the environment of which is controlled by the Sertoli cell. Meiosis and the differentiation of spermatids occurs in the adluminal compartment. The inter-Sertoli cell junctions transiently remodel to allow germ cells to move from the basal to the adluminal compartments, whilst protecting the functionality of the barrier. Diagram provided by Jenna Haverfield.
The number of Sertoli cells determines the ultimate spermatogenic potential of the testis. In rodents, Sertoli cells proliferate in fetal and early postnatal life and even into adulthood, reviewed in (93), whereas in humans there are two waves of proliferation; during the fetal and early neonatal period when the population increases 5 fold, and again prior to puberty when the population increases more than two fold (98), reviewed in (93, 99). Studies in mice show that apoptosis of Sertoli cells during fetal life results in abnormal cord development, smaller testes and reduced seminiferous tubule size (100), suggesting the proliferation of Sertoli cells during the fetal period is an important driver of seminiferous tubule formation. That Sertoli cell number determines the total sperm output of the testis, reviewed in (93, 101), is emphasized by studies showing that perinatal induction of hypothyroidism extends the duration of Sertoli cell proliferation, which in turn leads to increased Sertoli cell numbers and increased sperm output of the adult testis (91, 102). Other Sertoli cell mitogens such as FSH and activin (103-104), together with thyroxine, can also exert significant changes in the number of Sertoli cells in the testis, depending on the temporal pattern of their secretion. The latter must occur before the cessation of Sertoli cell proliferation. In the rat, this occurs at about 20 days whereas in the human, Sertoli cells cease to divide during the pubertal process (98). It is possible that the failure of many men with hypogonadotropic hypogonadism to achieve normal testicular size and normal sperm counts, when treated by gonadotropic stimulation, may result from abnormal Sertoli cell proliferation during fetal and prepubertal life resulting in a decreased Sertoli cell complement (105).
LEYDIG CELLS AND STEROIDOGENESIS
The Leydig cells lie within the intertubular regions of the testis and are found adjacent to blood vessels and the seminiferous tubules (5, 106). They are the cell type responsible for testosterone production which is essential for the maintenance of spermatogenesis. There are very significant organizational differences in the intertubular tissue betweens species reflecting the number of Leydig cells and differing architecture involving blood vessels and lymphatic sinusoids (107). Additionally, fibroblasts, macrophages, lymphocytes and small numbers of mast cells are found in the intertubular regions of the testis (108-109), reviewed in (110-111).
In most species there are two populations of Leydig cells, fetal and adult (112-113), that differ in terms of morphology, androgen synthesis, and regulation by paracrine and autocrine factors, reviewed in (110, 114-115). The fetal population appears following gonadal sex differentiation (gestational weeks 7-8 in humans) and, under the stimulation of hCG, results in the production of testosterone during gestation (116). In the human, these cells decrease in number towards term and degenerate and are lost from the intertubular region at about twelve months of age (117), although recent lineage-tracing experiments have indicated that fetal Leydig cells persist in the postnatal rodent testis (118). The adult population of Leydig cells in the human results from LH stimulation commencing at the time of puberty. This generation arises by division and differentiation of mesenchymal precursors under the influence of LH (119). Evidence in humans also supports a third neonatal Leydig cell population that peaks at 2-4 months after birth although their function is poorly understood (120), for review see (110). Whether or not the various Leydig cell populations share a common stem cell precursor also remains unclear (111).
Much of the data investigating gene regulatory systems that control fetal and adult Leydig cell differentiation is derived from rodent models, and differences may exist in the human. For example, placental hCG action via the LH/hCG receptor is required for human fetal Leydig cell development but not for mouse fetal Leydig cells (121). However, both species have in common the two main factors that influence fetal Leydig cell differentiation; Desert hedgehog (Dhh) and Platelet-derived growth factor A (Pdgfa). Interestingly both of these factors are Sertoli cell-derived and act in a paracrine fashion via their respective receptors, Patched1 (Ptch1) and platelet-derived growth factor receptor A (Pdgfra), on fetal Leydig cells to stimulate differentiation and steroidogenesis ((122-124), also see (110) and references therein). Dhh and Pdgfra also play an important role in adult Leydig cell development (124-126). Targeted deletion of Sertoli cell Dhh in mice causes major reductions in fetal Leydig cell number and androgen synthesis and results in undescended testes and feminized external genitalia (124, 127). A similar phenotype, termed complete pure gonadal dysgenesis, is observed in 46,XY patients with mutations in the DHH gene (128). A number of other important regulatory genes are also recognized to influence fetal and adult Leydig cell differentiation [e.g. Wt1, (129), Nrg1 (130), Inhba (125), for review see (110).
Leydig cells have the capacity to synthesize cholesterol from acetate or to take up this substrate for steroidogenesis from lipoproteins (106, 131). Typical of any steroid secreting cell, the Leydig cell contains abundant smooth endoplasmic reticulum and mitochondria which have tubular cristae that are unique to steroidogenic cells. The enzymes required for steroidogenesis are located in the mitochondria and in endoplasmic reticulum requiring intracellular transport of substrates between these organelles to achieve successful androgen production.
Leydig cells also produce the peptide hormone, insulin-like factor 3 (INSL3), which is structurally related to the insulin, IGF1 and IGF2 family (132-133), for review see (134). Targeted disruption of the Insl3 gene in mice causes bilateral cryptorchidism due to failure of gubernaculum development during embryogenesis (133). In the adult testis, INSL3 acts via its receptor, RXFP2 (formerly known as LGR8) found both on meiotic and post-meiotic germ cells, and on Leydig cells themselves (135-136). In gubernacular tissue, RXFP2 expression is up-regulated by androgen and abolished by an androgen receptor antagonist, suggesting a link between INSL3 and androgen signaling pathways (137). INSL3 has an anti-apoptotic function in the germ cell compartment (136), and could form part of an autocrine feedback loop in Leydig cells (135) which respond in vitro by increasing cyclic AMP and testosterone (138). In the human testis, INSL3 is a constitutive biomarker of both Leydig cell differentiation status and cell number, otherwise known as Leydig cell ‘functional capacity’ (134). This functionality has been useful to follow pubertal onset and increasing testicular volume (139) or to evaluate treatment for hypogonadism (134, 140), but does not have predictive value for sperm retrieval in patients with Klinefelter’s syndrome (141).
Control of Testosterone Production
Testosterone is the major androgen secreted by the Leydig cells found in the inter-tubular spaces of the testis. These cells arise from mesenchymal precursors and studies in the rat have identified that these precursors express the platelet-derived growth factor-α but not 3β hydroxysteroid dehydrogenase (142). Further, they suggest that many of these precursors are situated in close proximity to the surface of the seminiferous tubules. A normal male produces approximately 7 mg testosterone daily but also produces lesser amounts of weaker androgens such as androstenedione and dihydroepiandrosterone. In addition to testosterone, through the actions of the enzyme 5α reductase, the more potent androgen dihydrotestosterone is produced by the testis in smaller amounts. The testis also contributes approximately 25% of the total daily production of 17β-estradiol through the local action of the enzyme aromatase which converts androgenic substrates to this estrogen (143) (also see Endotext, Endocrinology of Male Reproduction, Chapter 17, Estrogens and Male Reproduction (144)). The remainder of the circulating estradiol is produced by the adrenal and peripheral tissues through the actions of aromatase. The biosynthesis and regulation of testosterone production is covered extensively elsewhere in Endotext (Endotext, Endocrinology of Male Reproduction, Chapter 2, Androgen Physiology, Pharmacology and Abuse (145)).
It is important to recognise that LH enhances the transcription of genes that encode a range of enzymes in the steroidogenic pathway (for reviews see (111, 114)) and that continued LH stimulation results in Leydig cell hypertrophy and hyperplasia (119, 146-147). In the normal male, the episodic nature of LH stimulation is likely to avoid prolonged periods of Leydig cell refractoriness to LH stimulation (148). It is recognized that the testosterone secretory capacity of the human testis declines in ageing men (for review see (149)) and this has been shown to result from a reduction in the efficacy of the ageing testis to respond to intravenous pulses of LH (150). These researchers showed that the estimated down-regulation of the Leydig cell achieved by exogenous LH pulses was augmented in these healthy older men making them refractory to further pulses for a longer period. (138).
It is well accepted that the level of production of androgens and estrogens by the testis can regulate bone mass, with decreased production causing osteoporosis. More recently, the production of osteocalcin by bone has been shown to influence testicular function (151), reviewed in (152). Using co-cultures of osteoblasts with testicular tissue, osteocalcin acted via G-protein coupled receptors (Gprc6a) to stimulate testosterone production (153).
Control of Leydig Cell Function by Other Testicular Cell Types
As alluded to earlier, Leydig cell development and function is critically dependent on other testicular cell types including Sertoli-, germ-, macrophages and peritubular myoid (see below) cells. In particular, a significant body of evidence has accumulated from studies in rodents to suggest that the seminiferous tubules influence Leydig cell number, maturation and testosterone production (154-155) (156). This data emerges from various experimental approaches where changes in Leydig cell function have been demonstrated, including knockout or over-expression of the androgen receptor or other signaling genes in Sertoli cells (157-158), temporary disruption of spermatogenesis via antagonist or toxicant treatment (159) or heat-treatment (160-161), or acute ablation of Sertoli or germ cell types to study global changes in Leydig cell function ((84, 97) for review see (162)). Collectively, these data show that Sertoli cells support adult Leydig cell development and survival by recruiting and maintaining their progenitors, and by regulating steroidogenic function (158, 162). These conclusions are supported by observations from unilateral testicular damage, such as that induced by cryptorchidism or efferent duct ligation, wherein the Leydig cells from the testis with spermatogenic damage show an increased capacity for testosterone biosynthesis and a decrease in LH receptor number (163-164). In contrast, germ cells appear to have little direct impact on Leydig cell gene expression in adulthood (156, 159), although post-meiotic germ cells have major impacts on Sertoli cell gene expression (162).
While similar mechanisms are difficult to identify in the human, it is recognized that elevated LH and low to low-normal testosterone concentrations, indicative of compromised Leydig cell function, are found in 15-20% of men with severe seminiferous tubule failure. Further support for the concept that the state of spermatogenesis can affect the function of the Leydig cells in men has emerged from the studies of Andersson et al (165), who showed that lower testosterone and higher estradiol concentrations were present, and accompanied by higher LH levels in infertile men. They concluded that this may reflect an extension of testicular dysgenesis to affect steroidogenesis or alternatively may result from inter-compartment interactions in the testis. There is also increasing support for the concept that environmental factors such as the phthalates are able to influence Leydig cell function (166). In utero exposure of rats to di(n-butyl)phthalate during the masculinization programming window in fetal life has been shown to cause focal testicular dysgenesis as expressed by Leydig cell aggregation and malformed seminiferous tubules (166). These features were linked to impaired intra-testicular testosterone levels and a decreased ano-genital distance, an emerging marker of deficient androgen action in utero.
Compelling evidence exists to demonstrate that other interstitial cells can also impact Leydig cell function. In particular, when resident testicular macrophages are absent, Leydig cells fail to develop normally, whereas activated macrophages suppress Leydig cell steroidogenesis (for reviews see (85, 162, 167)). Androgen action via the peritubular myoid cell androgen receptor is also essential for the normal differentiation and function of adult Leydig cells (discussed below) (168). The nature of the factors and molecular mechanisms involved in intercellular communication between Leydig cells and the various other testicular cell types remains unknown.
ROLE OF PERITUBULAR MYOID CELLS
External to the basement membrane of the seminiferous tubule, are several layers of modified myofibroblastic cells termed peritubular myoid cells (PMCs) (169-170). PMCs are contractile and are responsible for the irregular contractions of the seminiferous tubules which propel seminiferous tubule fluid and released spermatozoa through the tubular network to the rete testis (171). PMC contractility is stimulated by various factors, reviewed in (171) including endothelin, prostaglandin F2 alpha and angiotensin (172-174). These contractions are associated with dramatic changes in PMC shape and their cytoskeletal actin networks (175). PMCs and Sertoli cells both contribute to the composition of the basement membrane that surrounds the seminiferous tubules, reviewed in (171). PMCs also produce various growth factors such as activin A and platelet derived growth factors (176-177), that may influence the function of other testicular cells, reviewed in (171).
PMCs have long been known to influence Sertoli cell function and protein expression, reviewed in (178) and the presence of Sertoli cells is required for normal PMC development and function (84, 97). PMCs influence Sertoli cell number, function and ability to support germ cell development, as revealed by studies in mice lacking androgen receptor expression in PMCs (179). This model also revealed that PMCs influence Leydig cell development and steroidogenesis (168). Further studies in transgenic mice reveal that an R-spondin receptor, LGR4, is selectively expressed in PMCs, participates in Wnt/β-catenin signaling and is necessary for germ cell development during meiosis (180). PMCs, under the influence of androgen, secrete the growth factor glial cell line-derived neurotrophic factor (GDNF), which is necessary for the maintenance of the spermatogonial stem cell niche (181-182). Therefore it is clear that PMCs modulate spermatogenesis via the regulation of Leydig, Sertoli and germ cell development and function.
THE REGULATION OF SPERMATOGENESIS
Many studies in the past 30 years have focused on the endocrine regulation of spermatogenesis. It is clear that the gonadotropins LH and FSH are required for the initiation and maintenance of quantitatively normal spermatogenesis. LH targets the Leydig cells to stimulate androgen biosynthesis, and the resulting androgens (testosterone and its androgen metabolites) act on receptors within the seminiferous epithelium to stimulate and support spermatogenesis. FSH targets receptors in the Sertoli cells directly to support spermatogenesis. However the roles of other endocrine factors, such as vitamin A and its metabolite retinoic acid, are emerging. While both androgens and FSH are required for optimal spermatogenesis (see below), spermatogenesis relies on the local production of growth factors, signaling molecules and other intrinsic mechanisms.
The following sections consider key aspects of the regulation of Sertoli cells and germ cell development and function, with the roles of the “traditional” endocrine regulators, androgen and FSH, briefly discussed at the end of each section. The role of estrogens in spermatogenesis is considered elsewhere in Endotext (Endocrinology of Male Reproduction Section, Chapter 17, Estrogens and Male Reproduction (144)).
Regulation of Sertoli cell Development and Function
The complexity of the Sertoli cell’s structure and function is reflected in the complexity of its regulation. A detailed review on the many factors regulating Sertoli cell function is out of the scope of this chapter, and only a few important functions will be discussed here. The reader is referred to the excellent book on Sertoli cell Biology (183) for comprehensive information.
The Sertoli cell population is specified in the embryonic testis, under the influence of male sex determining factors, such as Sry and Sox9, reviewed in (184-185). Newly-specified embryonic Sertoli cells enclose and form seminiferous cord structures around primordial germ cells. Expression of the retinoic acid degrading enzyme Cyp26b1 and other factors by early Sertoli cells (E12.5 in the mouse) controls the specification of primordial germ cells to commit to the male pathway of gene expression and meiosis (186). Sertoli cells proliferate and drive seminiferous cord elongation late in embryonic development; this process is dependent on activin A signaling from Leydig cells to Sertoli cells, reviewed in (185).
Sertoli cells proliferate in late fetal life and before puberty. Prior to puberty, the exit of Sertoli cells from an immature, proliferative phase to a non-proliferative, maturation phase represents an important cell fate decision that results in the establishment of the adult Sertoli cell population. Experimental modifications that interfere with these periods of Sertoli cell proliferation and maturation can impact on the ultimate size and spermatogenic output of the adult testis; extended periods of Sertoli cell proliferation increase testis size (e.g. (187)), whereas premature cessation of proliferation and entry into the maturation phase results in smaller testes (e.g. (188)). Several factors act as mitogens for immature Sertoli cell proliferation, including FSH (189), thyroid hormone (187), and transcription factors, such as Dmrt1 (190) and Rhox genes (191), and various other genes are essential for the proliferation to maturation switch, reviewed in (88).
As the Sertoli cells attain an adult phenotype capable of supporting sperm production, their nucleus moves to the base of the cell, they attain the specialized cytoskeletal features characteristic of these cells (192) and they form the so-called ‘blood testis barrier’ tight junctions necessary for the entry of germ cells into meiosis (78). As Sertoli cells develop during puberty and the first wave of spermatogenesis, they show an extraordinary degree of plasticity in terms of their gene expression program, which reflect functional changes, and their response to the appearance of different germ cell types, as they mature (193). In adulthood, Sertoli cells increase or decrease the expression of genes depending on the stage of the spermatogenic cycle (67). This cyclic expression of genes allows the Sertoli cell to respond to the changing needs of germ cells as they proceed through spermatogenesis.
It has been known for many years that an absence of vitamin A disrupts cyclic function of Sertoli cells and spermatogenesis. It is now clear that the metabolism of Vitamin A to the active metabolite retinoic acid (RA) is essential for the cyclic activity of Sertoli cells, reviewed in (194). Retinoic acid signaling is mediated through nuclear RA receptors (RARs) that bind to DNA and either activate or suppress target genes. Mice lacking RARα expression in Sertoli cells show disruption of the spermatogenic cell cycle, whereas the administration of exogenous RA to testes without advanced germ cells causes all Sertoli cells to “reset” to stage VII of the spermatogenic cycle (70). These studies indicate that RA is a master driver of Sertoli cell cyclic gene expression.
Multiple lines of evidence suggest there is a very specific pulse of RA synthesis at the mid-spermatogenic stages VII and VIII ((70, 195), reviewed in (194)) which have been confirmed by studies measuring RA in synchronized testes (196). This pulse may be achieved by a combination of events including an increase in RA synthesis enzymes (ALDH enzymes), a decrease in enzymes that store or degrade RA, and an increase in the RA uptake protein Stra6 in Sertoli cells. Advanced germ cells such as pachytene spermatocytes could possibly synthesise RA and may contribute to this mid-cycle peak (see (194). Recent studies suggest that ALDH enzymes are unlikely to play a major role in the mid-cycle RA pulse (197) but stage-specific expression of enzymes involved in the rate limiting conversion of retinol to retinaldehyde, or enzymes involved in retinol availability, could play a role (196-197). Termination of the RA pulse in late stage VIII could be facilitated by a sharp increase in the expression of the RA degradation enzyme Cyp26a1 (70), however other studies did not support this concept (196).
This pulse of RA in the mid-spermatogenic stages is thus likely to be a driver of Sertoli cell function. It not only appears to be necessary for the entry of spermatogonia into meiosis (see below) but it also likely regulates other important Sertoli cell functions occurring in these stages, notably sperm release (see (198) and references therein) and the formation and maintenance of the blood-testis-barrier (e.g. (197, 199-200), reviewed in (194)). Therefore the mid-cycle peak of RA likely drives these stage-specific Sertoli cell functions and cycle-dependent gene expression, highlighting its role as a driver of Sertoli cell cyclic function. The precise mechanisms governing the pulsatile nature of the RA production and response pathways in the seminiferous epithelium, however, remain to be elucidated.
FSH and Androgen Regulation of Sertoli Cells
Sertoli cells, unlike germ cells, express receptors for androgens and FSH, and thus “transduce” the effects of these hormones to the developing germ cells. Spermatogenesis does not proceed in the absence of androgens, whereas spermatogenesis can proceed but is quantitatively reduced in the absence of FSH (reviewed in (156, 201-203)). It is well known that both of these hormones are needed for quantitatively normal spermatogenesis. Both androgens and FSH have independent effects on Sertoli cells, but also act co-operatively and synergistically to initiate and maintain normal spermatogenesis and, by inference, optimal Sertoli cell functions.
FSH acts a mitogen for pubertal Sertoli cell proliferation and in the absence of FSH or its receptor, testes are smaller, Sertoli cell populations are reduced, as is sperm output (201, 204). Interestingly, FSH requires the insulin/IGF signaling pathways to mediate its effects on pubertal Sertoli cell proliferation (205). Thus FSH supports postnatal Sertoli cell proliferation to establish a quantitatively normal population and, since Sertoli cell number determines sperm output (see The Role of Sertoli Cells in Spermatogenesis), is required for the production of normal numbers of sperm. Another event occurring during the establishment of spermatogenesis is a wave of germ cell apoptosis that is important for establishing future spermatogenesis, perhaps by achieving a balance in the Sertoli cell:germ cell ratio (206). Since reductions in FSH at this time cause even greater apoptosis, it is possible that FSH acts on Sertoli cells to limit this apoptotic wave and establish normal spermatogenesis, reviewed in (201). FSH appears to support various Sertoli cell functions and their ability to support normal numbers of germ cells, as evidenced by reduced Sertoli cell-germ cell ratios in mice lacking FSHβ (207) and abnormal Sertoli cell morphology in mice lacking FSH receptor (208). FSH can maintain germ cell development in gonadotropin-deficient men for 6 weeks (209), and has permissive effects on spermatogenesis in non-human primates and men, see (210-211). Therefore FSH is not essential for spermatogenesis, but is required for normal Sertoli cell number and function.
Androgens, including testosterone and DHT, act on androgen receptors (AR) in the testis to support normal spermatogenic function. Androgens can act on the AR and produce the so-called classical signaling pathway, whereby ligand-bound AR translocates to the nucleus, binds to Androgen Response Elements (AREs) in the promoter region of androgen-dependent genes, and modulates transcription. This pathway produces a response hours after androgen stimulation. However androgens can have much more rapid effects via non-classical pathways, involving AR-mediated intracellular calcium influx or activation of SRC and the ERK phosphorylation pathway, reviewed in (212). Both classical and non-classical pathways are active in Sertoli cells (212) and both are necessary for spermatogenesis (213).
In the absence of AR in Sertoli cells, no sperm are produced and spermatogenesis is arrested at the end of meiosis (214-215), highlighting the fact that androgen action on Sertoli cells is needed for the completion of meiosis and spermiogenesis. Androgens regulate Sertoli cell number during pubertal development (reviewed in (201)) and are a driver of Sertoli cell maturation; this latter requirement was demonstrated in transgenic mice with premature activation of AR expression in postnatal Sertoli cells, causing Sertoli cells to prematurely exit the proliferative phase and enter the maturation phase, leading to a reduction in Sertoli cell numbers (188). Thus the precise timing of AR expression in Sertoli cells is important for normal testis development and optimal sperm output. Androgens are necessary for the normal formation of tight junctions between Sertoli cells which contribute to the blood-testis-barrier, reviewed in (201), and they drive the expression and translation of many genes expressed in the Sertoli cells themselves, and indirectly modulate the expression of genes in germ cells (e.g. (216)). Interestingly, Sertoli cell morphology, function and androgen-dependent gene expression is impaired when AR is ablated from peritubular myoid cells (179), indicating that androgen action on these cells also supports Sertoli cell function and spermatogenesis.
As reviewed extensively elsewhere, androgens and FSH have co-operative and synergistic effects on spermatogenesis (156, 201, 203-204) and, since Sertoli cells are the only testicular cells to express both FSH and androgen receptors, some synergistic actions likely occur within the Sertoli cells themselves. Their ability to support germ cells is impaired when Sertoli cells lack expression of either FSH receptors or AR, however the effect is exacerbated when both receptors are depleted (217). Similar synergistic actions of FSH and androgen in Sertoli cells are apparent when measuring the ability of Sertoli cells to release mature sperm at spermiation (218). FSH and androgen signaling pathways can converge in Sertoli cells, for example in activating the MAP kinase pathway and elevating intracellular Ca2+ levels, reviewed in (219) and both hormones co-operate to modulate the Sertoli cell expression of particular miRNAs (220).
Regulation of Spermatogonial Proliferation and Development
Spermatogonia and SSC reside within a specialized microenvironment within the testis known as the “niche”, where the balance between SSC renewal and differentiation is regulated. This niche is comprised of cells, extracellular matrix and soluble factors that regulate the functions of cells within the niche. Within this niche, the expansion of spermatogonial clones and their commitment to differentiation are the foundation for the continual production of spermatozoa during adulthood.
Maintenance of the niche and the balance between SSC renewal and differentiation in the testis is regulated by a number of factors, see (221-223) for reviews. The Sertoli cell directly dictates the number and function of spermatogonial niches (224). Sertoli cells secrete Glial-cell line Derived Neurotrophic Factor (GDNF) which acts on receptors on undifferentiated spermatogonia to control differentiation and self-renewal of SSC (225-229) via the regulation of several transcription factors (221). Sertoli cells also regulate the stem cell niche via the expression of the Etv5 gene and by mediating FGF9 responses, reviewed in (90) as well as by the production of other factors such as activin A, reviewed in (223).
Other somatic cells within the testis are important for SSC self-renewal and differentiation. An example is colony stimulating factor (Csf1), expressed by the surrounding peritubular myoid cells and Leydig cells, that has been demonstrated to be important for SSC self-renewal (230). Intriguingly, macrophages have recently been shown to be important for maintenance of the spermatogonial niche; distinct macrophage populations aggregate on the surface of the seminiferous tubule over regions containing undifferentiated spermatogonia, and their depletion disrupts spermatogonial differentiation (231). The mechanism by which local resident macrophages may promote spermatogonial differentiation is not yet known, but it may involve their expression of Csf1 and enzymes involved in retinoic acid synthesis (231).
In the neonatal period, the migration and proliferation of the primordial germ cells and the subsequent pre-spermatogonia (gonocytes) represents a crucial step in the establishment of spermatogenesis (232-233). In turn, the constant commitment of type A spermatogonia to differentiation and entry into meiosis is a key aspect driving the spermatogenic cell cycle (70) and thus in providing the “pulses” of sperm production along the seminiferous tubule. A fundamental requirement for both gonocyte differentiation and spermatogonial commitment to meiosis is the action of stem cell factor (SCF) produced by the Sertoli cells and its receptor, c-KIT, located on spermatogonia (232). Action of Sertoli cell-derived SCF on c-KIT induces the PI3 kinase signaling pathway in spermatogonia which is required for their entry into meiosis (234). The acquirement of c-KIT protein on the surface of spermatogonia is a key marker of differentiation and is essential for spermatogonial development and entry into meiosis (232-233).
Vitamin A and the retinoic acid signaling pathway are emerging as critical regulators of spermatogonial differentiation. As described above (see Regulation of Sertoli cell Development and Function) a “pulse” of retinoic acid is generated at the mid-stages of spermatogenesis via a tightly controlled series of events, including the regulation of retinoic acid synthesis and degradation enzymes (70, 196, 235-236). Retinoic acid is required for the differentiation of neonatal gonocytes and for the differentiation of spermatogonia in the post-pubertal testis, and thus is an essential factor required to drive entry of spermatogonia into meiosis, reviewed in (196, 222, 237). Ectopic expression of retinoic acid receptor gamma drives undifferentiated spermatogonia to differentiate (238), highlighting a direct action of retinoic acid on spermatogonia. Retinoic acid may drive spermatogonial differentiation by stimulating the PI3K-AKT-mTOR signalling pathway to induce the translation of c-KIT protein (239) as well as other proteins involved in spermatogonial differentiation (240).
FSH and Androgen Regulation of Spermatogonia
The above section demonstrates that local factors within the testis support the spermatogonial stem cell nice, the expansion of cohorts of both undifferentiated and differentiated spermatogonia and entry into meiosis. What impacts do the major endocrine regulators of spermatogenesis, FSH and androgen, have on spermatogonial differentiation and proliferation?
Combined suppression of androgen and FSH results in a relatively small decrease in spermatogonial populations in rodents but causes a major block in spermatogonial development in primates and humans, reviewed in (241). It is clear that androgen and FSH have supportive effects on spermatogonia, but there is species-specific variability in the sensitivity of these cells to each of these hormones, reviewed in (204, 241-242).
Spermatogonia lack receptors for both FSH and androgen and therefore actions of these hormones must be indirect, via Sertoli cells and/or other testicular somatic cells. Studies in rodents suggest that spermatogonial development is not particularly susceptible to a loss of androgens and that spermatogonia can enter meiosis in the absence of androgen action on Sertoli cells (e.g. (215, 243)). Ablation of AR within peritubular myoid cells results in reduced numbers of spermatogonia (179) however it is not clear if this is a peritubular myoid cell-mediated effect, or whether the high concentrations of testicular testosterone produced in this model have inhibitory effects on spermatogonia, as noted in other studies (244). Conversely, spermatogonia are very sensitive to FSH in rodents and monkeys, e.g. (243, 245-246), therefore it is possible that the major reductions in spermatogonial populations in response to androgen and FSH suppression in monkeys and humans is primarily a consequence of FSH, rather than androgen, depletion. The mechanism by which FSH supports spermatogonia is likely to be via stimulating the Sertoli cell to provide a supportive environment for maintenance of the SSC niche, as well as on spermatogonial proliferation and differentiation. Studies in rodents have shown that FSH regulates the levels of GDNF and FGF2 in Sertoli cells, which in turn are essential for spermatogonial development, reviewed in (247). A recent study in transgenic mice suggests that maintenance of the SSC niche is normal in mice lacking FSH and therefore it may not play a major role in stimulating GDNF-dependent effects on SSC (248). Interestingly, this study also revealed that SSC renewal is enhanced during LH (and probably testosterone) suppression, and this effect is mediated by the transcription factor Wnt5a in Sertoli cells (248); perhaps this mechanism could preserve the SSC pool in situations where endocrine factors are temporarily compromised.
Regulation of Meiosis
Meiosis technically begins with the differentiation of type B spermatogonia into preleptotene spermatocytes which begin DNA synthesis. However, spermatogonia become committed to further differentiation and entry into meiosis during the A to A1 transition; this commitment to meiosis is an irreversible step leading to the production of preleptotene spermatocytes (237). There is abundant evidence that entry into meiosis in both sexes, and the production of spermatocytes in males in particular, requires the RA pathway, reviewed in (237). In the absence of the RA-inducible gene Stra8, preleptotene spermatocytes are formed and replicate their DNA, but their subsequent entry into the meiotic prophase is prevented (249-250). RA also induces Rec8, a meiosis-specific component of the cohesion complex, in a Stra8-independent manner, suggesting that RA acts through multiple pathways to initiate meiosis (251). However initiation of meiosis is not solely dependent on RA, as it also requires a RA-independent protein, MEIOC, that stabilizes mRNA transcripts from multiple meiosis-associated genes (252).
Many studies, including those in transgenic mouse models, have identified proteins necessary for the completion of male meiosis, reviewed in (253). Targeted gene disruption approaches have also identified sexually dimorphic meiosis-associated proteins, suggesting different levels of checkpoint control between males and females, particularly in terms of chromosome recombination and homologous pairing, see (254). Failure of normal meiotic recombination events is related to an increased incidence of gamete aneuploidy, which has a higher incidence in infertile men compared to case controls, reviewed in (255). Many proteins have been shown to be essential for male meiotic recombination events, including those involved in synaptonemal complexes and DNA repair mechanisms, reviewed in (253, 255) . For example, genetic ablation of the DNA repair protein PMS2 results in very few synaptonemal complexes forming and improper homologous chromosome pairing (256). Meiosis is not arrested however, and some abnormal sperm are produced (256). The induction of spermatocyte apoptosis and arrest at the spermatocyte phase is commonly observed in other transgenic models in which the expression of other meiotic recombination proteins is perturbed , reviewed in (253).
Many proteins are required for male meiotic division, see (253). For example, the testis-specific heat shock protein, HSP 70-2, is essential for male meiosis. It is required for desynapsis of the synaptonemal complexes and for the activation of CDC2 to form the active CDC2/cyclin B1 complex to enable progression into the first meiotic division (34, 257). The ability of HSP 70-2 to activate CDC2 is regulated by the interaction with a testis-specific linker histone chaperone, tNASP (258). Recent studies have revealed that a neuropeptide, nociceptin, in Sertoli cells acts on its receptor in spermatocytes to stimulate the phosphorylation of Rec8, a key regulatory component of the cohesin complex that mediates chromosome dynamics during meiosis, including synaptonemal complex formation and chromosome recombination (259). Nociceptin-mediated Rec8 phosphorylation stimulates chromosome dynamics and meiotic prophase progression, reviewed in (260). These latter studies highlight the fact that the progression and completion of meiosis relies on cues from the Sertoli cell.
The transcriptional regulator A-MYB (encoded by the Mybl gene) is likely a important regulator of male meiosis (261). A point mutation in Mybl1 in mice causes spermatocyte arrest, aberrant chromosome synapsis, defects in DSB repair and abnormal cell cycle progression. Chromatin immunoprecipitation experiments revealed that A-MYB directly targets various genes involved in different aspects of meiosis, suggesting that A-MYB is a “master” transcriptional regulator of male meiosis (261).
FSH and Androgen Regulation of Meiosis
It is well known that the completion of meiosis requires androgen. Meiosis arrests at the pachytene/diplotene stage in mice lacking AR in Sertoli cells, and no haploid spermatids are produced (214-215). However, spermatocyte numbers are even further reduced when AR is ablated from peritubular myoid cells (179), suggesting that androgenic support of meiosis is mediated via both Sertoli cells and peritubular myoid cells. Meiosis was disrupted in pubertal rats when the non-classical AR pathway was blocked, suggesting that meiosis requires rapid actions of androgen on testicular somatic cells (213). Interestingly, while the completion of meiosis is absolutely dependent on androgen, it requires comparatively lower levels of androgen than the later process of spermiogenesis (203, 262-263).
Mice lacking FSH show a modest but significant reduction in the progression of meiosis (207), perhaps via effects on spermatocyte survival. It is well known the both FSH, as well as androgen, can support meiotic cell survival, particularly in the hormone-sensitive stages VII and VIII. Preleptotene and pachytene spermatocytes in stages VII and VIII are particularly vulnerable to FSH and/or androgen suppression, and apoptosis of these cells is a feature of gonadotropin suppression, reviewed in (203). The replacement of either FSH or androgen prevents spermatocyte loss/apoptosis in rodents (264) and humans (209), highlighting the fact that both of these hormones can support meiotic germ cell survival.
Regulation of Spermiogenesis and Spermiation
As discussed earlier in this chapter, the steps in the formation of a sperm from its precursor, the haploid round spermatid, represent a fascinating process in cell biology. The development of the sperm tail, the remarkable nuclear changes involving the condensation and complexing of DNA, the cessation of transcription and delay in protein translation, and the changes in the relative positions of the nucleus, cell organelles and the cytoplasm, all pose innumerable questions as to how these events are controlled. Many genes and proteins have been implicated in the control of these cellular processes during spermiogenesis, as demonstrated by transgenic mouse models, reviewed in (36-37).
The intrinsic and tightly-regulated control of gene transcription and translation is especially important for the complex cellular differentiation occurring during spermiogenesis. Haploid spermatids, as well as meiotic spermatocytes, express many unique genes that are not expressed in somatic cells (265). Alternative splicing is highly prevalent in the testis, and generates many germ cell-specific transcripts likely important for carrying out the ordered procession of germ cell development (266). One example of the importance for alternative splicing in spermiogenesis is the CREM gene, whereby the use of alternative splicing mechanisms regulates the expression of either repressor or activator forms of the CREM transcription factor (267).
Alternative polyadenylation is another mechanism that is particularly utilized within the testis to increase the diversity of the transcriptional program. mRNA polyadenylation involves cleavage of the pre-mRNA at its 3’ end, followed by the addition of multiple adenosine residues, creating what is known as the polyA tail. Polyadenylation can modulate the mRNA transcript’s stability, localization, splicing and translation (268). The position at which the polyA sequences are inserted can vary on a cell and tissue-specific basis, leading to a phenomenon known as alternative polyadenylation, reviewed in (269). Many mRNAs in the testis are subjected to alternative polyadenylation, and can lead to the production of germ cell-specific isoforms (269). RNASeq analyses have revealed widespread alternative polyadenylation (including within introns and exons) and 3’UTR shortening during germ cell development, with the shortest 3’UTRs observed in round spermatids (270). Not all round spermatid genes displayed shortened 3’UTRs, however those that did had functions associated with sperm maturation and protein ubiquitination. The authors propose that alternative polyadenylation is a major feature of germ cell development, and that 3’UTR shortening may be important for the storage and translation of spermatid-specific mRNAs during spermiogenesis (270).
Spermiogenesis uses other unique mechanisms to modulate transcription (also see Gene Transcription and Translation During Spermatogenesis: Roles of Noncoding RNAs and Epigenetic Modifiers). The transcription factor CREM is a master regulator of the transcription of many genes involved in haploid spermatid development reviewed in (271). The activation of CREM target genes is influenced by CREM binding to a spermatid-specific co-activator protein known as ACT. The localization of ACT in the nucleus of spermatids is controlled by a kinesin, whereby the kinesin effectively exports ACT out of the nucleus at certain stages, thus inhibiting CREM-dependent gene transcription (272). These studies reveal sophisticated and unique mechanisms governing the control of gene transcription during spermiogenesis. Other round spermatid transcription factors that could be “master transcriptional regulators” and influence the expression of a large number of genes involved in spermiogenesis include TRF2 (273) and RFX2 (274); the latter appears to target a cohort of genes involved in the development of the flagella (274).
As spermatids lose their ability to perform active gene transcription during the remodeling of their chromatin into the compact sperm nucleus, the post-transcriptional and translational control of gene expression becomes particularly important. All mRNA transcripts expressed in meiotic and post-meiotic germ cells are subjected to some degree of translational repression and there are many examples whereby genes transcribed earlier on in germ cell development are translationally repressed until the proteins are required during spermatid elongation, reviewed in (275). mRNAs are stored in free messenger ribonucleoproteins (mRNPs) for 3 or more days in round spermatids, followed by translational activation in elongating or elongated spermatids. The mechanisms governing translational repression are not well understood, but an emerging candidate is the YBX2 protein. This protein binds to sequences near the 3’ end of the 3’UTR in well known translationally repressed genes, such as Prm1, and likely interacts with various proteins and cis-elements to promote the assembly of a repressive complex that inhibits translation (276-277). YBX2 can therefore selectively inhibit the translation of certain genes, however it is also likely to participate in global mRNA translational repression in round spermatids (276-277).
The proper development of the sperm flagella is essential for sperm motility and hence fertility. Many proteins are now known to be required for flagella development and motility, reviewed in (37). Even structurally normal sperm can fail to move as shown by the genetic inactivation of the gene encoding a sperm calcium ion channel (278). Mutations in a number of genes required for assembly of the axoneme, such as dyenin, are associated with a syndrome known as Primary Ciliary Dyskinesia (PCD). PCD is associated with a range of pathologies, including male infertility, and is caused by a failure of proper development and function of cilia in various organs, including the sperm flagellum (279). The identification of the molecular mechanisms governing flagellar development and motility is important for the development of new therapies for male infertility.
Both spermiogenesis and spermiation appear to be regulated by the retinoic acid signaling pathway, reviewed in (194). Sertoli cell-derived RA acting on RARα/RXRβ heterodimers in Sertoli cells is essential for spermiation, reviewed in (35, 194). Deletion of the gene encoding RARα, Rara, from Sertoli cells causes abnormalities in both spermiogenesis and spermiation, reviewed in (194). Interestingly, the expression of Rara in spermatids rescues the spermiogenesis and spermiation defects seen in Rara null mice, suggesting that germline expression of Rara is also important for spermiogenesis and spermiation (280).
The regulation of spermiation is very complex, as reviewed extensively elsewhere (35, 60) The complexity of its regulation is due to the fact that spermiation is actually a multifaceted process involving a co-ordinated series of cellular processes, signaling cascades, endocytic pathways and adhesion complexes. Abnormalities in different aspects of spermiation are seen in many experimental settings, including the administration of pharmacological agents, toxicants and environmental stressors, the suppression of hormones and the introduction of genetic mutations (35, 59, 61). It seems likely that the Sertoli cell directs spermiation; the mature spermatid at this time is transcriptionally inactive and thus likely plays a fairly passive role in the process (35, 59). However, there are examples of mutations in genes expressed in spermatids that impair the ability of the spermatid’s cytoplasm to by removed during spermiation, leading to a failure of spermatid release, reviewed in (35). Therefore the regulation of spermiation is governed by the Sertoli cell, but defects within spermatids can influence their ability to be released.
FSH and Androgen Regulation of Spermiogenesis and Spermiation
Both spermiogenesis and spermiation are well known targets of androgen action in the testis. While the complete ablation of androgen action in Sertoli cells causes an arrest at the end of meiosis (214-215), androgen insufficiency causes a failure of round spermatids to attach to Sertoli cells and enter the elongation phase of spermiogenesis, and the failure of mature spermatids to be released at the end of spermiation, e.g. (218, 281-282), see (202-203) for review. Spermiation failure is an early feature of androgen suppression during adult spermatogenesis, however continued suppression eventually causes the death and/or detachment of round spermatids from Sertoli cells so that they are unable to elongate into mature spermatids (218).
Spermiation failure is observed when gonadotropins are suppressed in rodents, monkeys and men (241). It is induced rapidly after gonadotropin suppression and is the first morphological disturbance to spermatogenesis (35). In men undergoing gonadotropin suppression for the purpose of male contraception, spermiation failure can occur early in some men, leading to a rapid decline in sperm counts (283). Whether or not spermiation failure is achieved could determine whether male hormonal contraceptive-mediated gonadotropin suppression induces azoospermia (zero sperm in the ejaculate) or oligospermia (low but detectable levels of sperm in the ejaculate), reviewed in (35).
It seems likely that androgens and FSH co-operate to regulate spermiation. Acute suppression of FSH alone causes spermiation failure in rats (218), whereas the administration of FSH to men undergoing gonadotropin suppression can support spermiation (209). Suppression of either FSH or testosterone alone causes significant spermiation failure in rats, but the suppression of both has a synergistc effect, indicating that both hormones co-operate to promote spermiation (218). Thus the action of both testosterone and FSH on Sertoli cells support the normal release of sperm at the end of spermatogenesis.
Regulation of Gene Transcription and Translation During Spermatogenesis: Roles of Noncoding RNAs and DNA methylation
The long process of spermatogenesis, taking up to 64 days in men (284), involves an incredibly complex program whereby the transcription and translation of thousands of genes is precisely constrained as the germ cell proceeds through proliferation, meiosis and spermiogenesis. The male germ cell transmits both genetic and epigenetic information to the offspring, and as such the modulation of the germ cell genome has a major impact on subsequent generations. Epigenetic modifications of the genome are heritable; epigenetic processes such as DNA methylation and histone modifications regulate chromatin structure and modulate gene transcription and silencing.
The transcriptome of the male germ cell during meiosis and spermiogenesis is the most complex transcriptome of all tissues in the body; substantially more of the germ cell genome is transcribed and subjected to more complex alternative splicing compared to other tissues (285). The regulation of this transcriptome is central to successful spermatogenesis and for male fertility. The precise constraints on gene and protein expression in germ cells, and on the sperm genome as a whole, are achieved via a number of different processes including RNA binding proteins, epigenetic modifiers, such as DNA methylation and transposable elements, and multiple types of noncoding RNAs (ncRNAs). The following section provides a brief overview of the ncRNA and epigenetic processes that contribute to each stage of male germ cell development. The reader is encouraged to seek more detailed reviews on specific mechanisms, e.g. (286-292), and references therein.
ncRNAs do not encode proteins but regulate gene transcription and translation. They are arbitrarily classified into small ncRNAs of less than 200 nucleotides (nt) and long ncRNAs (lncRNAs, >200nt). Small ncRNAs are further sub-classified based on their size, function, mode of action and whether they interact with PIWI proteins (expressed only in the germline) or AGO proteins (widespread expression). Three major classes of small ncRNAs have been shown to play essential roles in spermatogenesis: 1) MicroRNAs (miRNAs) interact with AGO family proteins and generally act at the post-transcriptional level to regulate mRNA stability and/or translation; 2) Endogenous small interfering RNAs (endo-siRNAs) are derived from double stranded RNAs, interact with AGO proteins and can silence both gene and transposon transcripts; 3) PIWI-associated RNAs (piRNAs) are derived from single-stranded piRNA precursors and interact with PIWI proteins (a sub family of the AGO protein family). piRNAs are predominantly, but not exclusively, found in the male germline and regulate transposable element activity as well post-transcriptional gene expression and are required for normal spermatogenesis (see below). miRNA and endo-siRNA generation involves the RNA processing enzyme Dicer, whereas piRNA generation is Dicer-independent (reviewed in (291)).
Although these ncRNAs have various roles including regulating the epigenome (see below), they are probably best known for their role in RNA silencing; prevention of an mRNA transcript being translated into a protein. This is accomplished by the RNA silencing-induced complex (RISC), the core of which consists of an AGO/PIWI protein and the ncRNA that acts to “guide” the RISC to its target mRNA. Silencing of the target mRNA is then achieved by cleavage (by the “slicer” activity of various proteins), or by recruiting other proteins that affect translation, transcript stability or chromatin structure, reviewed in (286-287, 291).
The Embryonic Testis
The control of epigenetic modifications of the genome, and the participation of ncRNAs, is very important in the fetal testis. The genomes of primordial germ cells undergo widespread demethylation as they colonise the embryonic gonad; this erasure of epigenetic information allows the subsequent establishment of a germline-specific epigenetic pattern that is eventually transmitted to the offspring (reviewed in (292)). After sex determination in the embryonic gonads, primordial germ cells become committed to the male pathway of differentiation and cease proliferation, entering a period of mitotic quiescence. During differentiation and the subsequent mitotic quiescence, remarkable modifications are made to the male germ cell genome. After the erasure of vast areas of DNA methylation earlier in development, fetal male germ cells undergo the re-establishment of DNA methylation marks by de novo DNA methyltransferases; this process is essential for gametogenesis and creates an epigenome that is required for successful embryonic development of the offspring (reviewed in (292)). During this time there are also extensive histone modifications of the genome that impact on chromatin structure and, ultimately, on embryonic development of the offspring (reviewed in (292)).
A striking feature of fetal male germ cells is the regulation of transposons, or transposable elements, which is central to the successful development and function of the male gamete. Transposable elements are DNA sequences that are “mobile”; they can literally move from one area of the genome to another. Retrotransposons make up the majority of transposable elements in the genome and are replicated by a “copy and paste” mechanism whereby the transcription of the transposon’s DNA sequence is “copied” into RNA and, via reverse transcription, into DNA, and then inserted (pasted) into another area of the genome (293). Transposons can thus create heritable alterations of the genome. At least 48% of the human genome is comprised of transposable elements and these elements are a major driver of generating genetic diversity during evolution (see (294) for recent review). Transposons can modulate gene expression by a variety of mechanisms, such as by modulating regulatory elements within promoter regions or generating noncoding functional elements that will impact on gene transcription and translation (294). However, transposons rarely produce beneficial effects and instead could have potentially deleterious consequences, thus evolution has produced sophisticated mechanisms to control their activity.
Potentially harmful transposon activity in the genome is repressed by the methylation of transposon DNA sequences. However the genome-wide de-methylation that occurs during fetal male germ cell re-programming could leave the germ cell genome vulnerable to increased transposition. For example, deletion of the de novo DNA methyltransferase Dnmt3l or of the Morc1 gene in male germ cells disrupts the methylation of retrotransposon sequences and leads to an activation of retrotransposon transcription and the eventual failure of germ cell development (295-296). Thus the processes governing DNA methylation of transposons during male germ cell development is essential for safeguarding the genome against unwanted transposable element activity.
Another important mechanism that has evolved within germ cells for the control of transposable elements involves piRNAs. The fetal testis expresses a unique set of piRNAs, termed fetal piRNAs ((297), reviewed in (291)). piRNAs expressed in pro-spermatogonia in the fetal testis and in spermatogonia in the postnatal testis are further classified as “pre-pachytene piRNAs”, to distinguish them from piRNAs involved in the postnatal development of spermatocytes. Fetal pre-pachytene piRNAs associate with the PIWI proteins MIWI and MILI2, and approximately half of all fetal piRNAs arise from sequences within transposable elements (reviewed in (288)). piRNAs and the MIWI and MILI2 proteins are essential for transposable element suppression in the fetal testis (298-299). piRNAs, in association with PIWI proteins, appear to silence transposon activity in the genome at a) the posttranscriptional level, by targeting and cleavage of transposable element transcripts, and b) at the epigenetic level, via the recruitment of DNA methylation machinery to re-establish repressive methylation marks on the promoters of transposable elements (reviewed in (286, 288, 291)). Thus piRNAs and their associated proteins defend the genome against inappropriate transposable element activity during fetal male germ cell development (287).
Although mechanisms to control transposon activity in the male germline have evolved, it is apparent that fetal male germ cells are still inherently vulnerable to transposable elements during genome de-methylation and re-methylation. Intriguingly, it has been proposed that the transposon-mediated generation of genetic diversity within individual male germ cells could be critical for the evolution of complex species such as mammals (300). Evolution is driven by a basic algorithm of “generate variation and test”: the generation of individuals with genetic and phenotypic variation, and the subsequent natural selection of those variants that offer the best opportunity to survive and reproduce. Transposons introduce genetic diversity, particularly into the promoter regions of the germ cell genome, and could thus be an important driver of the generation of genetic variation. The consequences of such genetic diversity derived by transposable elements are then tested by the subsequent survival and reproduction of the offspring. However, this “testing” of the genetic variation could also occur during the spermatogenic process itself, as individual germ cells proceed through spermatogenesis and fertilization. Such tests could include whether the gamete: is eliminated via apoptosis during spermatogenesis; is released by the Sertoli cell at the end of spermiation; survives and traverses the female reproductive tract; achieves fertilization; contributes to a viable zygote. Therefore transposon-mediated shuffling of the germ cell genome and the subsequent selection of sperm could be an important driver of mammalian evolution (300).
The Postnatal Testis
The male germline expresses high levels of ncRNAs that are involved not only in the generation of sperm, but also in shaping the sperm epigenome and in the ability of the sperm genome to have influence future generations (e.g. (286-288, 291, 301-302)). In germ cells, a specialized organelle known as the nuage, or germ granule, exists. The germ granule contains various ncRNAs and other related molecules (290, 303) and changes its structure and composition as germ cells develop through the fetal and postnatal periods. The germ granule exists in a form known as intermitochondrial cement (IMC) in fetal germ cells, postnatal spermatogonia and spermatocytes and as an intriguing germ cell-specific structure called the chromatoid body in spermatocytes and spermatids (290, 304). In round spermatids, the chromatoid body is highly mobile, moving rapidly around the nucleus, frequently making contact with nuclear pores, and even moving across intercellular bridges into adjacent spermatids (290). The chromatoid body is thought to function as an organizing center for RNA and ncRNA, performing important roles in the post-transcriptional processing of germ cell gene products (290).
While the modulation of DNA methylation of the epigenome of germ cells is a major feature of embryonic testis development (see above), it is worth noting that epigenetic modifications of the DNA in developing postnatal germ cells is also important for successful spermatogenesis, see (305). An example of this is the epigenetic “switch” involved in spermatogonial differentiation (306). Spermatogonia exhibit major epigenetic differences in DNA methylation patterns as they develop from Aal to A1 spermatogonia, and the DNA methylation machinery is involved in the shift from an undifferentiated, KIT- state towards a differentiating KIT+ state (306). Changes in DNA methylation of the germ cell genome throughout meiosis and spermiogenesis are associated with the ability of germ cells to transcribe RNA (285). Epigenetic modifications in the mature sperm are particularly important for the development of the offspring (see next section).
Analysis of the testis transcriptome has revealed that spermatocytes and spermatids transcribe more of their genome than any other tissue examined (285). While round spermatids are very abundant and are a major contributor to the testicular transcriptome, pachytene spermatocytes transcribe very high levels of RNA, ~6 times more than round spermatids, and therefore also contribute to the diversity of the testis transcriptome. Spermatocytes and spermatids transcribe substantially more genic and intergenic regions of DNA than other tissues, including many lncRNAs and pseudogenes, and exhibit a much more complex pattern of alternative splicing. The widespread transcription of the genome in these cells is associated with decreased DNA methylation and an open and transcriptional active chromatin state (285); presumably this open chromatin state is a consequence of the dramatic remodeling of the chromosomes and chromatin that occurs during meiosis and spermiogenesis. This “promiscuous” germ cell transcription is conserved across amniote species and could have important evolutionary consequences (285). While it is likely that much of this transcription is “leaky’” and non-functional, it could also be associated with the emergence of new genes and the generation of genetic diversity during mammalian evolution (285).
miRNAs are highly conserved and bind to complementary sequences in target mRNAs, preventing their efficient translation into proteins via a number of mechanisms including transcript cleavage and destabilization. A single miRNA can target many mRNA transcripts, and a single mRNA transcript can be the target of multiple miRNAs; in this way miRNAs are estimated to regulate ~60% of the protein coding genes in the genome (reviewed in (286)). miRNAs are generated from short hairpin loop RNA sequences that are subjected to a series of processing steps in the nucleus and then in the cytoplasmic RISC, reviewed in (286, 291, 307-308). miRNAs commonly arise from sequences within the introns of protein coding genes, reviewed in (286), and miRNA genes are significantly enriched within the X chromosome compared to autosomes, see (291). The enzymes RNA processing enzymes DROSHA and DICER are essential for miRNA biogenesis and are both required male fertility, see (286, 291, 307); spermiogenesis is disrupted when Drosha and Dicer are ablated from postnatal germ cells (309). Many miRNAs are preferentially expressed in the testis and in particular germ cells, including in spermatids and spermatozoa (310), reviewed in (286, 291). A number of germ cell miRNAs have now been shown to play defined roles during spermatogenesis, reviewed in (286, 291). Androgens and FSH can regulate particular miRNA species in Sertoli cells which in turn modulate the expression of particular proteins (220). Various studies suggest a correlation between altered miRNA profiles and particular disorders of human spermatogenesis, suggesting that miRNA-regulated pathways have important consequences for human male fertility, reviewed in (286).
The role of endo-siRNAs in spermatogenesis is less clear, but these small RNAs have the potential to influence the spermatogenic transcriptional program. As is the case for miRNAs, endo-siRNAs require processing by DICER and interactions with AGO proteins to exert their RNA interference activity, however unlike miRNAs, endo-siRNAs do not require processing by the DROSHA enzyme reviewed in (286, 291). In C. elegans, the male germline expresses specific endo-siRNAS that are important for spermatogenesis (311) and mutants with defective endo-siRNA expression exhibit male sterility (312). Mouse spermatogenic cells express 75 endo-siRNAs that have the potential to target hundreds of transcripts (313). Interestingly, the fact that these endo-siRNAs map to thousands of sequences within DNA (313) has lead to the hypothesis that these small RNAs could have an impact on the sperm epigenome (291, 313).
piRNAs are essential for adult spermatogenesis. This class of ncRNA consists of sequences ~25-30nt in length, slightly longer than miRNAs and siRNAs (288). piRNAs are predominantly expressed in the germline, however piRNA-like species (pilRNAs) have now been described in various somatic cells, including Sertoli cells (314). Millions of distinct piRNA sequences are thought to exist in mammals, although these sequences are poorly conserved between species (288). Their biogenesis is distinct from, and less well characterized than, the biogenesis of miRNAs, and piRNAs are 2’O-methylated on their 3’ end to prevent their degradation (286-288). piRNAs specifically interact with the PIWI sub-family of the AGO proteins, which includes PIWIL1, PIWIL2, and PIWIL4 (also known as MIWI, MILI, and MIWI2, respectively); PIWIL2 and 4 interact with piRNAs in gonocytes whereas PIWIL1 and 2 interact with piRNAs in meiotic and post-meiotic germ cells. Different sub-species of piRNAs exist in the postnatal testis: the so-called “pre-pachytene piRNAs” are expressed in fetal gonocytes and spermatogonia in the postnatal testis; whereas “pachytene piRNAs” are expressed in spermatocytes and spermatids of the postnatal testis. While pre-pachytene piRNAs are often derived from transposon sequences (see above), pachytene piRNAs are mostly derived from intergenic regions known as piRNA clusters, reviewed in (288). Pachytene piRNAs constitute approximately 95% of piRNAs and are very highly expressed in meiotic and post-meiotic germ cells, reviewed in (286-288, 291). A fundamental role for piRNAs in adult spermatogenesis has been revealed in many studies, reviewed in (286-288, 291); transgenic mice with targeted disruption of piRNA interacting proteins are usually infertile, with germ cell DNA damage and an arrest of spermatogenesis during meiosis or spermiogenesis being commonly observed, see (288).
While a role for piRNAs in the regulation of the epigenome in fetal gonocytes has been well described (see above), the specific roles of piRNAs in adult spermatogenesis are less clear, possibly because piRNAs could have widespread functions in the postnatal testis. Elevation of transposon sequences is seen in adult germ cells from mice with various genetic defects in piRNA associated proteins (e.g (315-317)), indicating that piRNAs may also repress transposable elements during adult spermatogenesis. Consistent with this proposition, the most abundant piRNAs in human sperm target LINE1 retrotransposon sequences (318). Various studies suggest that piRNAs are essential for the execution of the complex meiotic and post-meiotic transcriptional program (e.g. (319-321)). Pachytene spermatocytes from transgenic mice lacking a functional Henmt1 methylation gene have abnormally methylated piRNAs, which influences their stability and results in their degradation (315). Spermatocytes from these mice had a more “open” and transcriptionally permissive chromatin state compared to wildtype, suggesting a role for piRNAs in maintaining normal chromatin structure in germ cells. This abnormal chromatin state was associated with premature germ cell gene transcription, suggesting that piRNAs might regulate postnatal germ cell gene transcription via epigenetic mechanisms (315). Another way that piRNAs may influence the germ cell transcriptional program is by negatively regulating the expression of mRNAs from particular protein coding genes as well as lncRNA transcripts (320). Intriguingly this latter study also revealed that piRNAs can arise from non-coding pseudogenes and target the mRNAs arising from that pseudogene’s cognate functional protein-coding gene (320). Consistent with this finding, human sperm contain piRNAs that arise from pseudogenes and are predicted to target the expression of protein-coding genes (318). Thus the mechanisms by which piRNAs and their associated proteins regulate the spermatogenic program are likely many and varied, and the role of the piRNA pathway in spermatogenesis is the subject of ongoing studies.
In comparison to short ncRNAs, lncRNAs are less well studied and are a relatively recent addition to the field of male fertility research. lncRNAs (generally >200nt) can regulate gene expression by a number of mechanisms. For example, lncRNAs can act as repressors or enhancers of epigenetic modifiers of the genome, and can influence gene expression by regulating DNA methylation and histone modifications, reviewed in (322-323). RNASeq experiments show that lncRNAs are more abundant in testis than other tissues; this enrichment of lncRNAs in the testis is due to an over abundance of lncRNA transcripts particularly in spermatocytes and spermatids (285). lncRNAs are significantly more testis-specific than mRNAs (324), suggesting a particular requirement of lncRNAs for the spermatogenic process. Consistent with this, mouse germ cells at each developmental stage express specific lncRNAs (325). Experiments performed in Drosophila ablated 105 of the 128 testis-specific lncRNAs; a third of these mutants showed reduced or ablated male fertility and defects in spermiogenesis, suggesting that lncRNAs are particularly important for spermatid development (326). lncRNAs have also been implicated in regulating other aspects of germ cell development, reviewed in (322-323). A recent study identified the elegant mechanisms by which lncRNAs can influence spermatogenesis by studying a lncRNA essential for the maintenance of the spermatogonial stem cell niche (327). Transcription of this lncRNA (lncRNA03386) was stimulated in SSCs by the growth factor GDNF. This lncRNA is an antisense transcript of the Gfra1 gene, the receptor for GDNF, and it interacts directly with the Gfra1 gene, stimulating its transcription. Therefore GDNF stimulates the expression of a lncRNA which in turn enhances expression of its own receptor and facilitates its ability to stimulate SSCs (327). lncRNAs will likely emerge as important regulators of spermatogenesis and male fertility.
Sperm Epigenetic Modifications and Transgenerational Inheritance
Many epidemiological studies have shown that parental exposure to various lifestyle and environmental factors can increase the risk of chronic, non-genetic diseases in offspring, suggesting that epigenetic factors are transmitted from parents to their children. It is now clear that epigenetic modifications of the germ cell genome can be inherited and impact on multiple generations of offspring, i.e. have transgenerational effects. As detailed above, the genome of male gametes is remodeled during embryogenesis and postnatal spermatogenesis, resulting in the genome of mature sperm being extensively modified by DNA methylation and the retention of specific histone modifications, reviewed in (328). Alterations to the male germ cell epigenome can thus arise during the male’s embryonic development or during postnatal spermatogenesis (329).
There are now many examples of alterations in the sperm epigenome impacting on subsequent generations, reviewed in (330-331). The first evidence of epigenetic transgenerational inheritance via the male germ line came from studies in mice exposed to the endocrine disruptor vinclozolin, which is an agricultural fungicide with antiandrogenic activity, reviewed in (332-333). Female mice exposed to vinclozolin produced male offspring with spermatogenic and fertility defects and altered sperm DNA methylation; changes in the expression of DNA methylation enzymes and the sperm epigenome arose during the males’ embryonic exposure to vinclozolin (334) and these alterations were transmitted via the male germ line through subsequent generations of male offspring (335). Paternal obesity can also alter the sperm epigenome and have transgenerational impacts on offspring. Obesity induced by a high fat diet in male rats results in their female offspring exhibiting increased adiposity, insulin sensitivity, impaired glucose metabolism and pancreatic β cell dysfunction (336). Male mice fed a high fat diet also produce offspring with increased adiposity and insulin resistance, and this phenotype is associated with altered testicular mRNA, DNA methylation and sperm miRNA signatures in the fathers (337). While maternal deficiencies in folate are well known to cause abnormalities in offspring, it has been shown that male mice consuming a diet low in folate have altered sperm epigenetic profiles and produce offspring with various birth defects (338), providing further evidence of paternal diet being able to influence the sperm epigenome and the health of future generations. It is also important to note that alterations in the epigenome of sperm in men is associated with sperm quality and can influence their fertility, reviewed in (339).
Therefore it is clear that a man’s sperm epigenome can be altered by environmental (including diet and lifestyle) factors throughout his lifetime (329), and this altered sperm epigenome can influence both his fertility and the health of his future children. The mechanisms of male-specific transgenerational inheritance could involve multiple factors, such as the sperm epigenome, seminal fluid signaling and microbiome transfer (340). Transgenerational effects can be mediated by sperm via the alteration of its epigenome by DNA methylation machinery and the regulation of histone modifications, but also by RNAs and proteins within the sperm that can diffuse into the oocyte at fertilization (341). Some authors have speculated that alterations in sperm DNA methylation and histone marks may have less of an impact on subsequent generations, whereas sperm-borne RNAs could be of greater importance (328, 341). In C.elegans, male germline epigenetic inheritance involves Argonaute proteins and the generation of small ncRNAs that target female-specific germline mRNAs (342). Paternal miRNAs and endo-siRNAs in mouse sperm can regulate the transcriptome of fertilized eggs and early embryos (343) and traumatic stress in early life in male mice can impact on the health of subsequent generations via sperm-borne small RNAs (344). Recent studies have shed new light on an intriguing mechanism by which diet-induced changes in the sperm epigenome can impact on the offspring. A low protein diet in male mice affected the complement of small tRNAs fragments in sperm, and these tRNA fragments regulated genes that are highly expressed during early embryo development (345). Surprisingly, the sperm acquired this tRNA fragment complement during their post-testicular maturation as they traversed the epididymis, via the release of small vesicles called epididymosomes from epididymal cells (345). Therefore multiple pathways exist that can modulate the paternal sperm epigenome to impact on the offspring.
THE HYPOTHALAMIC-PITUITARY-TESTIS AXIS
The successful initiation of testicular function is dependent on the hypothalamic secretion of GnRH which in turn stimulates FSH and LH to act on the testis. These actions initiate spermatogenesis and testosterone production.It is well recognised that the testis in turn, through the secretion of hormones produced in the Sertoli and Leydig cells, exerts a negative feedback control on the production of gonadotropins.
The presence of such a negative feedback control by the testis on pituitary FSH and LH secretion is best demonstrated by the rapid rise of FSH and LH after castration. The mechanisms by which the secretion of FSH and LH increases in response to castration involves a rise in the hypothalamic secretion of GnRH and also involves direct actions at the pituitary level which allow an increase in pulse amplitude. Further, the fact that LH and FSH are co-secreted by the majority of gonadotroph cells in the anterior pituitary raises a number of unresolved questions as to how GnRH and the inhibitory signals act on the pituitary to result in the differential regulation of FSH and LH secretion.
The secretion of the gonadotropins FSH and LH are regulated by the episodic secretion of gonadotropin releasing hormone (GnRH) produced in the hypothalamus (also see Endotext, Endocrinology of Male Reproduction section, Chapter 5,Hypogonadotropic Hypgonadism (HH) and Gonadotropin Therapy (346)). There is now a substantial body of evidence that indicates that the kisspeptins, a family of neuropeptides localized to the arcuate nucleus of the brain are upstream regulators of GnRH secretion (for reviews see (347) (348). For instance, arcuate kisspeptin-neurokinin B-dynorphin expressing hypothalamic neurons are critically involved in the increase in gonadotropin secretion that occurs after gonadectomy (349). The regulation is further complicated by the isolation and characterization of gonadotropin-inhibitory hormone (GnIH), which acts both upstream of GnRH and also may operate at the levels of the gonads as an autocrine/paracrine regulator of steroidogenesis (350-351).
The pituitary secretion of FSH and LH by the gonadotrophs is also controlled by the feedback inhibition that occurs via the steroids, testosterone and estradiol (for an extensive review on the role of estradiol in the hypothalamic-pituitary-testis axis, see Endotext, Endocrinology of Male Reproduction section, Chapter 17, Estrogens and Male Reproduction (144)). The secretion of FSH and LH is also regulated by protein inhibitors, inhibin, secreted by the gonads, and follistatin, produced locally within the pituitary by the follicular-stellate cells (352), reviewed in (353). Follistatin exerts its inhibition of FSH secretion by its capacity to bind and block the actions of the activins A and B, the latter locally produced by the pituitary gland (354).
Control of LH Secretion
There is a substantial body of evidence to indicate that the steroid hormones testosterone, estradiol and dihydrotestosterone inhibit LH secretion (355). The demonstration that non-aromatisable androgens could inhibit LH secretion established that testosterone can exert its action directly without metabolism to estradiol or dihydrotestosterone (356-357). From the studies by Santen and Bardin (358), it is evident that testosterone acts at the hypothalamic level by decreasing GnRH pulse frequency without a change in pulse amplitude. The action of estradiol appears to be predominantly at the pituitary where it decreases LH pulse amplitude without changing pulse frequency (359). Further support for the action of testosterone at the hypothalamus emerged from the observation of a decrease in GnRH pulse frequency in portal blood (360). In addition, these studies demonstrated that treatment with estradiol lowered LH levels by decreasing LH pulse amplitude without altering GnRH secretory patterns in portal blood. These conclusions have been challenged by observations that a selective aromatase inhibitor, anastrozole, caused an increase in LH pulse amplitude and pulse frequency (359). These changes were seen in the presence of increased testosterone concentrations and were accompanied by an increase in LH and FSH. The investigators concluded that estradiol exerted a negative feedback by acting at the hypothalamus to decrease GnRH pulse frequency and at the pituitary to decrease the responsiveness to GnRH, both actions lowering LH secretion. Also see Endotext, Endocrinology of Male Reproduction section, Chapter 17, Estrogens and Male Reproduction (144).
Control of FSH Secretion
In addition to their feedback regulation of LH, testosterone and estradiol are also capable of suppressing FSH in the male (361). For many years, it was proposed that the action of the steroid hormones could account for the entire negative feedback exerted on FSH levels by the testis despite the existence of a hypothesis that a specific non-steroidal FSH feedback regulator named inhibin existed (362).
Over the past thirty years, a substantial body of evidence has accumulated to confirm the existence of a glycoprotein hormone termed inhibin that exerts a specific negative feedback inhibition on FSH secretion at the pituitary level (363). Two forms of inhibin have been isolated, namely inhibin A and inhibin B (364-367). These proteins represent disulphide-linked dimers of an α and β subunit. The alpha subunit is common both to inhibin A and B but the β subunits, though closely related, are different (αβA = inhibin A: aβB = inhibin B). Both inhibin A and inhibin B have the capacity to specifically inhibit FSH secretion by pituitary cells in culture. However, the circulating form in males is inhibin B. In contrast, dimers of the β subunit, termed activins (activin A = βAβA: activin B = βBβB; activin AB = βAβB) all have the capacity to stimulate FSH secretion by pituitary cells in culture (368-369). Finally, a structurally unrelated protein termed follistatin, has the capacity to suppress FSH secretion specifically by pituitary cells in culture (370-372). This action has been demonstrated to be due to the capacity of follistatin to bind and neutralize the actions of activin thereby suppressing FSH secretion (373).
In men and males from other species, testosterone, when administered in an amount similar or greater to its production rate, can suppress FSH as well as LH (355). However, in most instances there was a parallel and often greater suppression of LH secretion in contrast to the actions of inhibin (361). Further, there appears to be a difference in the response of FSH to testosterone in primates, where the actions are totally inhibitory in contrast to rats, where following an initial suppression of FSH by testosterone, higher doses caused a return of FSH levels to baseline (374-375).
Clear evidence for a physiological role of testosterone in the control of FSH can be shown in experiments in which the Leydig cells were destroyed by the cytotoxin ethane dimethane sulphonate (EDS). This treatment results in a rapid decline in testosterone levels and a concomitant increase in FSH concentrations to levels which were only 50% of those found in castrates (376). Since the inhibin levels in these experiments did not change, the maintenance of FSH levels at 50% of those seen in castrate animals was likely to be due to the continuing feedback control by inhibin (377). Further support for the dual role of testosterone and inhibin in the control of FSH emerged from the use of EDS in cryptorchid rats where baseline FSH levels were increased in association with decreased inhibin concentration. The removal of testosterone feedback in these animals with low basal inhibin levels resulted in an increase in FSH to the castrate range (378). The observation of an increase in FSH levels in men treated with a selective aromatase inhibitor raised the possibility that estradiol exerts a negative feedback action on FSH especially since the treated men experienced a concomitant significant increase in testosterone (359).
It is now well accepted that in the male, inhibin is produced by the Sertoli cell and is secreted both basally across the basement membrane of the seminiferous tubule and also into the lumen (379-380). Several studies have now demonstrated that the predominant form of inhibin secreted by the testis is inhibin B since the predominant mRNA was βB (381-382). The levels of inhibin B in males, measured by a specific ELISA, are inversely related to the levels of FSH (383-384). However, FSH predominantly stimulates inhibin α subunit production and does not alter the β subunit message (379, 385). This action results in the testis predominantly secreting inhibin rather than activin. Further support for this concept emerges from the studies of men undergoing chemotherapy where declining inhibin B levels are associated with a rise in FSH. However, with assays that detect α subunit products, there was a clear increase in these substances under the stimulation of elevated FSH levels (386). There is also evidence that a subunit of inhibin can be produced by Leydig cells (387) and increased LH levels result in the release of α subunit products into the circulation (388-389). There is still controversy as to whether the Leydig cells can produce bioactive inhibin (387).
In men, testosterone-induced gonadotropin suppression reduced circulating inhibin B and α subunit (measured as the pro-alpha C form of the α subunit) levels by only 25% and 50%, respectively, indicating that their secretion is not fully gonadotropin-dependent (390). In that model, exogenous FSH and LH both restored pro-alpha C levels supporting the view that Sertoli and Leydig cell are the origins of alpha subunit peptides, respectively, but only FSH restored inhibin B presumably reflecting Sertoli cell βB synthesis.
While there is evidence that the Sertoli cells, Leydig cells and peritubular myoid cells can produce activin, castration does not result in a decrease in circulating activin A levels (176, 391-393). Unfortunately, due to the lack of a suitable assay to measure activin B, there is no data available concerning the behaviour of this substance after castration. While activin acts on the pituitary, it also exerts local actions within the testis such as the stimulation of spermatogonial mitosis (394), Sertoli cell mitosis during testis development (103-104, 395-397) and possibly acts directly on germ cells (398).
Follistatin is also produced in the Sertoli cells, spermatogonia, primary spermatocytes and round spermatids in the testis (399-400). However, castration does not result in a net decrease in follistatin levels in the circulation suggesting that the testis does not contribute significantly to circulating levels of follistatin (401). In fact, in these studies follistatin levels rose but the rise was also found in the sham operated rams indicating that the follistatin response was part of the acute phase response to surgery, further supported by the demonstration that IL1β could also cause such an increase (402).
The fact that activin and follistatin remain unchanged after castration yet inhibin B in the circulation becomes undetectable strongly suggests that the gonadal feedback signal on FSH secretion is inhibin B. This is supported by studies in arcuate nucleus-lesioned monkeys maintained on a constant GnRH pulse regime, where testosterone could prevent the post-castration rise in LH but not FSH (403) (for review see (347)). The infusion or injection of recombinant human inhibin A in several species caused a rapid and specific fall in FSH secretion (404-406) and inhibin A administration to castrate rams suppressed FSH levels in the absence of testosterone (407).
Activin and follistatin can exert a paracrine role directly in the pituitary gland. The α and β subunit mRNAs are present in gonadotropes within the pituitary gland (408). The studies of Corrigan et al (409) strongly suggest that these substances exert a local action on FSH secretion since the inhibition of the action of activin B in pituitary cells in vivo suppressed endogenous FSH secretion. Follistatin mRNAs are also present in a number of different pituitary cell types including the folliculo-stellate cells (408, 410). This local production of follistatin also has the capacity to regulate the actions of activin (411). Additionally, the studies of Bilizekian et al have demonstrated that GnRH and the sex steroids estradiol and testosterone can modulate the local production of α, βA, βB and follistatin mRNAs within the pituitary (412-413). Clearly these interactions are complex and no clear answer can be given as to the relative roles of paracrine and endocrine actions of these glycoprotein hormones.
Some correlative evidence supporting the action of inhibin on FSH secretion is the decrease in inhibin production by Sertoli cells in parallel with the rise in FSH in a number of models of spermatogenic damage (414-415). The levels of circulating inhibin B appear to be inversely related to the levels of FSH following testicular damage in a number of studies (383-384, 416). Further, even in studies of large numbers of normal men, there is an inverse relationship between serum inhibin B levels and FSH (416). It is therefore likely that the actions of inhibin are predominantly exerted through secretion from the testis and transport via the peripheral circulation whereas the actions of activins and follistatin on FSH secretion occur through paracrine actions at the level of the pituitary gland. Further evidence supporting the stimulation of FSH by activin secretion emerges from the decline in FSH levels in mice with targeted disruption of the activin type II receptor gene (417).
SUMMARY OF THE ENDOCRINE REGULATION OF SPERM PRODUCTION: CLINICAL CONSIDERATIONS
Androgens and Spermatogenesis
The primary stimulus for the initiation of spermatogenesis is the LH-induced rise in testosterone at puberty. The absolute requirement of androgen for the initiation of spermatogenesis is demonstrated by the ability of the non-aromatisable androgen DHT to initiate complete spermatogenesis in hpg mice (418), and by the observation that spermatogenesis proceeds only to meiosis in mice lacking Sertoli cell AR expression (214-215). While androgens together with FSH are required for quantitatively normal spermatogenesis (see below), it is clear that androgens can initiate and support some degree of sperm production. Once spermatogenesis has been initiated during puberty, androgen alone can restore or maintain adult sperm production after experimentally-induced gonadotropin suppression, as has been demonstrated in many rodent, primate and human studies (reviewed in (156, 203, 241, 263, 419)).
By virtue of its local production in the testis, testicular concentrations of testosterone are 50 fold higher than that is serum, and are above those required for the initiation and maintenance of spermatogenesis. Adult spermatogenesis can be maintained by testicular testosterone levels at least 4 fold lower than normal as demonstrated in rodent models (420), reviewed in (419). When testicular testosterone levels are low, such as in the pre-pubertal testis and during gonadotropin suppression, the 5α-reduction of testosterone to the more potent androgen DHT appears necessary to amplify the androgenic signal and exert its stimulatory effects on spermatogenesis, as highlighted by studies in rodents (reviewed in (203)). However in the normal adult testis when testosterone levels are very high, it is likely that testosterone acts directly on the AR to maintain androgen-dependent functions (421).
The initiation of spermatogenesis during puberty requires a higher concentration of androgen than is required to maintain adult spermatogenesis once it is initiated, as exemplified by studies in hpg mice (422). Also, the restoration of adult spermatogenesis following gonadotropin suppression occurs over a very narrow dose range, wherein small changes in testicular androgen levels can produce large changes in sperm production, reviewed in (419). It is also worth noting that even very low levels of androgen are likely to produce a stimulatory effect on spermatogenesis. This can be illustrated by the demonstration of low levels of sperm production in older mice lacking LH receptor expression (423). Therefore, when considering the androgenic stimulation of adult spermatogenesis, “a little goes a long way”, and continued androgen action on AR can occur in the absence of gonadotropin stimulation, reviewed in (419).
Within the testis, AR is expressed in Sertoli cells, peritubular myoid cells, Leydig cells and vascular endothelial cells ((424-426), whereas germ cells lack AR and rely solely on somatic AR expression (427-428). Therefore androgens act on AR within the testicular somatic cells to support spermatogenesis. Studies in mice show that androgen action on AR in each of the testicular somatic cell types is important for testis function. AR expression in Sertoli cells is essential, as no sperm are produced in mice with targeted deletion of Sertoli cell AR expression (214-215) or in mice where the DNA binding domain of Sertoli cell AR has been deleted (429). However AR expression in peritubular myoid cells is also important for normal spermatogenesis (179) and for development and function of Leydig cells (168). The autocrine action of androgen on AR in Leydig cells is required for normal steroidogenesis and hence optimal testosterone production (428), and AR in endothelial cells of the testicular arterioles is involved in maintaining normal fluid dynamics and vasomotion in the testis (426). In summary, androgens act on AR in various testicular somatic cells, but not germ cells, to support normal testicular function and sperm production.
As summarized above, various phases of germ cell development are known to rely on androgen action. In the absence of androgen signaling in Sertoli cells, spermatocytes cannot complete meiotic division, and no haploid round spermatids are produced e.g. (214-215, 217, 429). The progression of haploid spermatids through spermiogenesis also relies on androgens, and in the absence of androgen, round spermatid development is halted during mid-spermiogenesis due round spermatid apoptosis and an inability of newly elongating spermatids to adhere to Sertoli cells (281, 430-431). The final release of spermatids during the process of spermiation is also sensitive to androgen and/or gonadotropin inhibition, reviewed in (35). Many functions of Sertoli cells are androgen-dependent, such as the maintenance of tight junction function at the blood testis barrier (432-434) and the production of androgen-responsive miRNAs (220), and are necessary to support germ cell development.
The mechanisms by which Sertoli cells support each androgen-dependent phase of germ cell development however, such as the signal required for the completion of meiosis (reviewed in (263)), are as yet unknown. Interestingly, the different androgen-dependent processes within germ cell development have different sensitivities to, or requirements for, androgens, reviewed in (419). For example, the completion of meiosis requires more androgen action than the completion of spermiogenesis (418). Individual variations in the sensitivities of different spermatogenic processes to androgens may explain why a correlation between sperm output and testicular testosterone levels has been so difficult to establish in gonadotropin-suppressed monkeys and men (390, 435-437).
FSH and Spermatogenesis
For many years, the relative roles of androgen vs FSH in initiating, restoring and maintaining spermatogenesis were unclear. This was in part due to the synergistic actions of these two hormones (see below), but also due to difficulties associated with investigating FSH action in a setting of complete androgen ablation. Transgenic mouse models have provided important information regarding specific roles for FSH in spermatogenesis, reviewed in (156, 263, 438). FSH receptors are found only on Sertoli cells and are expressed in a stage-dependent manner (439-440).
One of the most important functions of FSH is to establish a quantitatively normal adult Sertoli cell population. FSH acts as a mitogen for postnatal Sertoli cell proliferation and is required for establishing normal Sertoli cell numbers in mice, reviewed in (156, 204). Since Sertoli cell number determines spermatogenic output in adulthood (101), this function of FSH is important for optimal sperm production. Observations in transgenic mice also show that FSH is needed for normal Sertoli cell morphology and for their ability to support the maximal number of germ cells, e.g. (207-208, 217, 441).
FSH also plays an important role in the regulation of spermatogonia, as revealed in studies in hpg mice (217, 243) and primates (245-246). Numbers of type B spermatogonia correlate more closely with circulating FSH than testicular testosterone levels in gonadotropin-suppressed monkeys and humans (283, 442), indicating that these cells may be particularly supported by FSH. Transgenic human FSH expressed in hpg mice can also exert stimulatory effects on spermatocyte numbers, indicating a permissive effect on meiosis, (243) however FSH alone cannot support the completion of spermiogenesis. The acute suppression of FSH alone can also cause spermiation failure, presumably via effects on the Sertoli cell’s ability to release mature spermatids (218).
Optimal Spermatogenesis Requires Synergistic Actions of Androgens and FSH
The data reviewed above indicate that androgens and FSH have distinct roles in spermatogenesis but that these hormones also act co-operatively and synergistically to promote maximal spermatogenic output (156, 203-204, 219).
Androgens and FSH co-operate by supporting different aspects of germ cell development, for example FSH stimulation of spermatogonial populations and androgen stimulation of spermiogenesis. FSH establishes a quantitatively normal Sertoli cell population, whereas androgen initiates and maintains sperm production, thus both hormones co-operate via independent functions to enable maximal spermatogenic output.
Both androgens and FSH facilitate normal Sertoli cell morphology and function, which are likely essential for the ability of Sertoli cells to support the maximum number of germ cells. Both hormones also promote germ cell survival, particularly of spermatocytes and round spermatids in the mid-spermatogenic stages in rodents (264), reviewed in (204). The fact that both hormones can prevent germ cell apoptosis explains why either hormone can maintain germ cell development, at least in the short term, following gonadotropin suppression in humans (209).
There are many examples of synergy between testosterone and FSH, reviewed in (156, 203-204). It has been demonstrated in many experimental settings that testosterone and FSH can support spermatogenesis at a lower dose when the other is present, reviewed in (203). Testosterone and FSH likely act synergistically in the control of signaling pathways and gene expression in Sertoli cells, which in turn are important for germ cell development (156, 219). An example of such synergism is the demonstration that, after acute suppression of either androgen or FSH in rats, approximately 10% of mature spermatids failed to be released at spermiation, whereas suppression of both hormones resulted in 50% of spermatids failing to spermiate (218). Both testosterone and FSH modulate the expression of many miRNA species in Sertoli cells, which likely mediate a large spectrum of proteomic changes important for Sertoli and germ cell function (220).
It should be noted that there are species differences in the response of spermatogenesis to combined androgen and FSH suppression, reviewed in (241, 443). In rodents, suppression of gonadotropins causes a decline in spermatogonial populations but spermatogenesis is primarily arrested at the spermatocyte stage (444). In monkeys and humans however, spermatogenesis is primarily arrested at spermatogonial development, however meiosis and spermiogenesis can be maintained until they undergo a gradual attrition due to the lack of spermatogonia entering meiosis (241, 435, 442).
The requirement for both testosterone and FSH to support normal spermatogenesis in men was revealed in studies by Matsumoto and colleagues (445-446) whereby gonadotropins were suppressed by the administration of testosterone until suppression of spermatogenesis occurred. They then introduced injections of hCG to stimulate Leydig cell function and to restore intratesticular testosterone concentrations which increased sperm counts but not to pre-treatment levels (Figure 12). These data suggested that, in association with undetectable FSH levels, increasing intratesticular androgen could partially restore sperm output (446). Using the same model, they initiated hFSH treatment when sperm counts were suppressed and showed that, in the presence of low intratesticular testosterone concentrations, FSH alone could partially restore sperm output (447). The latter study strongly suggests a role for FSH which appears to be able to synergise with low testosterone to stimulate sperm production in men.

Figure 12
The response in the sperm counts from normal volunteers to a suppression of FSH and LH by testosterone injections is shown. Note the recovery in sperm counts when hCG and hFSH were introduced singly into the treatment regime. Data from Matsumoto et. al. (reference 171, 172) and Bremner et. al. (reference 172).
Considerations for the Stimulation of Sperm Production for Fertility Treatment
Male infertility due to undetectable (azoospermia) or low (oligozoospermia) numbers of sperm in the ejaculate may occur in many clinical settings. Details of the approach to the treatment of men with reduced sperm counts are reviewed elsewhere (346, 448-449). Gonadotropic stimulation of sperm production is appropriate in men with gonadotropin deficiency, such as hypogonadotropic hypogonadism (HH) or acquired androgen deficiency, may be of limited benefit in some men with oligospermia (449) but is of no or minimal benefit in men with non-obstructive azoospermia due to primary testicular failure (448) in whom gonadotropic drive is already high.
As androgens are essential for the initiation of sperm production, the induction of spermatogenesis in HH acquired after puberty is achieved by the administration of hCG (as an LH substitute), 1000-2000 IU sc 2-3 times per week (449). Prolonged therapy is required to produce sperm in the ejaculate (346, 449), given that human spermatogenesis takes more than 2 months to produce sperm from immature spermatogonia. Treatment with hCG alone may be sufficient for the induction of spermatogenesis in men with larger testes due to potential residual FSH action (346). However, for many men, and particularly for those with congenital HH, the co-administration of FSH (75–150 IU sc 3 times per week) is needed for maximal stimulation of sperm output (346, 449). In men with congenital HH, FSH is needed to induce Sertoli cell maturation, whereas men with acquired HH and smaller testes benefit from the co-administration of FSH due to the well known synergistic actions of FSH and androgens on spermatogenesis as described above. It is also worth nothing that in some men, treatment may need to be particularly protracted (1-2 years) to enable pubertal maturation of the testis, for example the induction of spermatogenesis in Kallmann’s syndrome (449).
Considerations for the Suppression of Sperm Production for Contraception
As detailed in this chapter, both androgens and FSH co-operate and synergize to stimulate spermatogenesis. In a male hormonal contraceptive context, this means that adequate suppression of both androgens and FSH is required to halt sperm production. The most promising contraceptive strategies in terms of efficacy and rate of sperm count suppression are based on a combination of non-androgenic steroids (e.g. progestins) to suppress gonadotropins, and testosterone to maintain physiological androgen actions outside the testis (see extensive review in Endotext, Endocrinology of Male Reproduction, Chapter 15, Male Contraception (450).
The induction of azoospermia is seen as desirable for maximal contraceptive efficacy and acceptability, however no contraceptive regimen as yet is able to consistently induce azoospermia in all men (450). As discussed above, a very narrow dose range exists between testicular testosterone levels and sperm output, meaning that a “little testosterone goes a long way”. In addition, the presence of even low levels of FSH likely potentiates the action of residual androgen on spermatogenesis. In practice, this means that achieving the level of testosterone suppression needed for complete suppression of spermatogenesis may be difficult in some men. A minority of men (~5%) undergoing combined hormone-based therapies fail to achieve adequate sperm count suppression (450). The complete abolition of androgen production does not appear to be achievable because of LH-independent androgen secretion by Leydig cells (423) and the need to maintain extra-testicular androgen actions in men. A complete elimination of androgen action on spermatogenesis could theoretically be achieved via testis-specific enzyme or androgen receptor inhibition, however novel therapeutic tools to achieve this have not yet been identified (see Endotext, Endocrinology of Male Reproduction, Chapter 2, Androgen Physiology, Pharmacology and Abuse (145)).
REFERENCES
- de Kretser D, Temple-Smith P, Kerr J (1982) Anatomical and functional aspects of the male reproductive organs. Handbook of Urology, Vol XVI, Disturbances in Male Fertility. 16: 1-131
- Jarow JP (1990) Intratesticular arterial anatomy. J Androl. 11(3): 255-9
- Dahl EV, Herrick JF (1959) A vascular mechanism for maintaining testicular temperature by counter-current exchange. Surg Gynecol Obstet. 108(6): 697-705
- Clermont Y, Huckins C (1961) Microscopic anatomy of the sex cords and seminiferous tubules in growing and adult male albino rats. Am J Anat 108: 79-97
- de Kretser D, Kerr J (1994) The cytology of the testis, in The Physiology of Reproduction, Knobil, E. and Neill, J.D., Editors. Raven Press: New York. p. 1177-1290
- Sharpe R (1994) Regulation of spermatogenesis, in The Physiology of Reproduction, Knobil, E. and Neill, J.D., Editors. Raven Press: New York. p. 1363-1434
- Russell LD, Griswold MD (1993) The Sertoli Cell. Clearwater, Florida: Cache River Press
- Dym M, Fawcett DW (1971) Further observations on the numbers of spermatogonia, spermatocytes, and spermatids connected by intercellular bridges in the mammalian testis. Biol Reprod. 4(2): 195-215
- Guan K, Nayernia K, Maier LS, Wagner S, Dressel R, Lee JH, Nolte J, Wolf F, Li M, Engel W, Hasenfuss G (2006) Pluripotency of spermatogonial stem cells from adult mouse testis. Nature. 440(7088): 1199-203
- Conrad S, Renninger M, Hennenlotter J, et al. (2008) Generation of pluripotent stem cells from adult human testis. Nature. 456(7220): 344-9
- Meistrich ML, van Beek M (1993) Spermatogonial stem cells. Cell and Molecular Biology of the Testis. 266-295
- Clermont Y (1972) Kinetics of spermatogenesis in mammals: seminiferous epithelium cycle and spermatogonial renewal. Physiol Rev. 52(1): 198-236
- Kanatsu-Shinohara M, Ogonuki N, Inoue K, Miki H, Ogura A, Toyokuni S, Shinohara T (2003) Long-term proliferation in culture and germline transmission of mouse male germline stem cells. Biol Reprod. 69(2): 612-6
- Nagano M, Avarbock MR, Brinster RL (1999) Pattern and kinetics of mouse donor spermatogonial stem cell colonization in recipient testes. Biol Reprod. 60(6): 1429-36
- Ogawa T, Dobrinski I, Avarbock MR, Brinster RL (2000) Transplantation of male germ line stem cells restores fertility in infertile mice. Nat Med. 6(1): 29-34
- Ogawa T, Dobrinski I, Brinster RL (1999) Recipient preparation is critical for spermatogonial transplantation in the rat. Tissue Cell. 31(5): 461-72
- Chan F, Oatley MJ, Kaucher AV, Yang QE, Bieberich CJ, Shashikant CS, Oatley JM (2014) Functional and molecular features of the Id4+ germline stem cell population in mouse testes. Genes Dev. 28(12): 1351-62
- Oatley MJ, Kaucher AV, Racicot KE, Oatley JM (2011) Inhibitor of DNA binding 4 is expressed selectively by single spermatogonia in the male germline and regulates the self-renewal of spermatogonial stem cells in mice. Biol Reprod. 85(2): 347-56
- Nagano MC, Yeh JR (2013) The identity and fate decision control of spermatogonial stem cells: where is the point of no return? Curr Top Dev Biol. 102: 61-95
- Hara K, Nakagawa T, Enomoto H, Suzuki M, Yamamoto M, Simons BD, Yoshida S (2014) Mouse spermatogenic stem cells continually interconvert between equipotent singly isolated and syncytial states. Cell Stem Cell. 14(5): 658-72
- Nakagawa T, Sharma M, Nabeshima Y, Braun RE, Yoshida S (2010) Functional hierarchy and reversibility within the murine spermatogenic stem cell compartment. Science. 328(5974): 62-7
- Clermont Y (1969) Two classes of spermatogonial stem cells in the monkey (Cercopithecus aethiops). Am J Anat. 126(1): 57-71
- van Alphen MM, van de Kant HJ, de Rooij DG (1988) Depletion of the spermatogonia from the seminiferous epithelium of the rhesus monkey after X irradiation. Radiat Res. 113(3): 473-86
- Schlatt S, Weinbauer GF (1994) Immunohistochemical localization of proliferating cell nuclear antigen as a tool to study cell proliferation in rodent and primate testes. Int J Androl. 17(4): 214-22
- Schulze C (1979) Morphological characteristics of the spermatogonial stem cells in man. Cell Tissue Res. 198(2): 191-9
- Plant TM (2010) Undifferentiated primate spermatogonia and their endocrine control. Trends Endocrinol Metab. 21(8): 488-95
- Ramaswamy S, Razack BS, Roslund RM, Suzuki H, Marshall GR, Rajkovic A, Plant TM (2014) Spermatogonial SOHLH1 nucleocytoplasmic shuttling associates with initiation of spermatogenesis in the rhesus monkey (Macaca mulatta). Mol Hum Reprod. 20(4): 350-7
- Gassei K, Ehmcke J, Dhir R, Schlatt S (2010) Magnetic activated cell sorting allows isolation of spermatogonia from adult primate testes and reveals distinct GFRa1-positive subpopulations in men. J Med Primatol. 39(2): 83-91
- Shinohara T, Orwig KE, Avarbock MR, Brinster RL (2000) Spermatogonial stem cell enrichment by multiparameter selection of mouse testis cells. Proc Natl Acad Sci U S A. 97(15): 8346-51
- Stubbs L, Stern H (1986) DNA synthesis at selective sites during pachytene in mouse spermatocytes. Chromosoma. 93(6): 529-36
- Heller CH, Clermont Y (1964) Kinetics of the Germinal Epithelium in Man. Recent Prog Horm Res. 20: 545-75
- Vrooman LA, Nagaoka SI, Hassold TJ, Hunt PA (2014) Evidence for paternal age-related alterations in meiotic chromosome dynamics in the mouse. Genetics. 196(2): 385-96
- De Kretser DM (1969) Ultrastructural features of human spermiogenesis. Z Zellforsch Mikrosk Anat. 98(4): 477-505
- Eddy EM (1999) Role of heat shock protein HSP70-2 in spermatogenesis. Rev Reprod. 4(1): 23-30
- O'Donnell L, Nicholls PK, O'Bryan MK, McLachlan RI, Stanton PG (2011) Spermiation: The process of sperm release. Spermatogenesis. 1(1): 14-35
- Hermo L, Pelletier RM, Cyr DG, Smith CE (2010) Surfing the wave, cycle, life history, and genes/proteins expressed by testicular germ cells. Part 2: changes in spermatid organelles associated with development of spermatozoa. Microsc Res Tech. 73(4): 279-319
- Hermo L, Pelletier RM, Cyr DG, Smith CE (2010) Surfing the wave, cycle, life history, and genes/proteins expressed by testicular germ cells. Part 3: developmental changes in spermatid flagellum and cytoplasmic droplet and interaction of sperm with the zona pellucida and egg plasma membrane. Microsc Res Tech. 73(4): 320-63
- Oko RJ, Jando V, Wagner CL, Kistler WS, Hermo LS (1996) Chromatin reorganization in rat spermatids during the disappearance of testis-specific histone, H1t, and the appearance of transition proteins TP1 and TP2. Biol Reprod. 54(5): 1141-57
- Steger K, Klonisch T, Gavenis K, Drabent B, Doenecke D, Bergmann M (1998) Expression of mRNA and protein of nucleoproteins during human spermiogenesis. Mol Hum Reprod. 4(10): 939-45
- Eddy EM (1998) Regulation of gene expression during spermatogenesis. Semin Cell Dev Biol. 9(4): 451-7
- Russell LD, Russell JA, MacGregor GR, Meistrich ML (1991) Linkage of manchette microtubules to the nuclear envelope and observations of the role of the manchette in nuclear shaping during spermiogenesis in rodents. Am J Anat. 192(2): 97-120
- Kierszenbaum AL, Tres LL (2004) The acrosome-acroplaxome-manchette complex and the shaping of the spermatid head. Arch Histol Cytol. 67(4): 271-84
- O'Donnell L, O'Bryan MK (2014) Microtubules and spermatogenesis. Semin Cell Dev Biol. 30: 45-54
- Fawcett DW (1975) The mammalian spermatozoon. Dev Biol. 44(2): 394-436
- Kierszenbaum AL (2002) Intramanchette transport (IMT): managing the making of the spermatid head, centrosome, and tail. Mol Reprod Dev. 63(1): 1-4
- Carrera A, Gerton GL, Moss SB (1994) The major fibrous sheath polypeptide of mouse sperm: structural and functional similarities to the A-kinase anchoring proteins. Dev Biol. 165(1): 272-84
- Fulcher KD, Mori C, Welch JE, O'Brien DA, Klapper DG, Eddy EM (1995) Characterization of Fsc1 cDNA for a mouse sperm fibrous sheath component. Biol Reprod. 52(1): 41-9
- Mandal A, Naaby-Hansen S, Wolkowicz MJ, et al. (1999) FSP95, a testis-specific 95-kilodalton fibrous sheath antigen that undergoes tyrosine phosphorylation in capacitated human spermatozoa. Biol Reprod. 61(5): 1184-97
- Mei X, Singh IS, Erlichman J, Orr GA (1997) Cloning and characterization of a testis-specific, developmentally regulated A-kinase-anchoring protein (TAKAP-80) present on the fibrous sheath of rat sperm. Eur J Biochem. 246(2): 425-32
- Miki K, Eddy EM (1998) Identification of tethering domains for protein kinase A type Ialpha regulatory subunits on sperm fibrous sheath protein FSC1. J Biol Chem. 273(51): 34384-90
- Vijayaraghavan S, Liberty GA, Mohan J, Winfrey VP, Olson GE, Carr DW (1999) Isolation and molecular characterization of AKAP110, a novel, sperm-specific protein kinase A-anchoring protein. Mol Endocrinol. 13(5): 705-17
- Kirichok Y, Navarro B, Clapham DE (2006) Whole-cell patch-clamp measurements of spermatozoa reveal an alkaline-activated Ca2+ channel. Nature. 439(7077): 737-40
- Strunker T, Goodwin N, Brenker C, Kashikar ND, Weyand I, Seifert R, Kaupp UB (2011) The CatSper channel mediates progesterone-induced Ca2+ influx in human sperm. Nature. 471(7338): 382-6
- Lishko PV, Botchkina IL, Kirichok Y (2011) Progesterone activates the principal Ca2+ channel of human sperm. Nature. 471(7338): 387-91
- Okunade GW, Miller ML, Pyne GJ, et al. (2004) Targeted ablation of plasma membrane Ca2+-ATPase (PMCA) 1 and 4 indicates a major housekeeping function for PMCA1 and a critical role in hyperactivated sperm motility and male fertility for PMCA4. J Biol Chem. 279(32): 33742-50
- O'Bryan MK, Sebire K, Meinhardt A, Edgar K, Keah HH, Hearn MT, De Kretser DM (2001) Tpx-1 is a component of the outer dense fibers and acrosome of rat spermatozoa. Mol Reprod Dev. 58(1): 116-25
- Gibbs GM, Scanlon MJ, Swarbrick J, Curtis S, Gallant E, Dulhunty AF, O'Bryan MK (2006) The cysteine-rich secretory protein domain of Tpx-1 is related to ion channel toxins and regulates ryanodine receptor Ca2+ signaling. J Biol Chem. 281(7): 4156-63
- Holstein AF (1976) Ultrastructural observations on the differentiation of spermatids in man. Andrologia. 8(2): 157-65
- Russell LD (1991) The perils of sperm release-- 'let my children go'. Int J Androl. 14(5): 307-11
- Russell L (1993) Role in spermiation, in The Sertoli cell, Russell, L.D. and Griswold, M.D., Editors. Cache River Press: Clearwater, FL. p. 269-302
- O'Donnell L (2014) Mechanisms of spermiogenesis and spermiation and how they are disturbed. Spermatogenesis. 4(2): e979623
- Leblond CP, Clermont Y (1952) Definition of the stages of the cycle of the seminiferous epithelium in the rat. Ann N Y Acad Sci. 55(4): 548-73
- Parvinen M (1982) Regulation of the seminiferous epithelium. Endocr Rev. 3(4): 404-17
- Perey B, Clermont Y, LeBlond CP (1961) The wave of the seminiferous epithelium in the rat. . Am J Anat 108: 47-77
- Regaud C (1901) Études sur la structure des tubes seminiferes et sur la spermatogenese chez les mammiferes. . Arch Anat Microsc 4: 101-156
- Clermont Y (1963) The cycle of the seminiferous epithelium in man. Am J Anat. 112: 35-51
- Johnston DS, Wright WW, Dicandeloro P, Wilson E, Kopf GS, Jelinsky SA (2008) Stage-specific gene expression is a fundamental characteristic of rat spermatogenic cells and Sertoli cells. Proc Natl Acad Sci U S A. 105(24): 8315-20
- Clouthier DE, Avarbock MR, Maika SD, Hammer RE, Brinster RL (1996) Rat spermatogenesis in mouse testis. Nature. 381(6581): 418-21
- Timmons PM, Rigby PW, Poirier F (2002) The murine seminiferous epithelial cycle is pre-figured in the Sertoli cells of the embryonic testis. Development. 129(3): 635-47
- Sugimoto R, Nabeshima Y, Yoshida S (2012) Retinoic acid metabolism links the periodical differentiation of germ cells with the cycle of Sertoli cells in mouse seminiferous epithelium. Mech Dev. 128(11-12): 610-24
- Fawcett D (1975) Ultrastructure and function of the Sertoli cell. Handbook of Physiology, Section 7, Endocrinology. Vol 5, Male Reproductive System: 21-55
- Vogl AW (1988) Changes in the distribution of microtubules in rat Sertoli cells during spermatogenesis. Anat Rec. 222(1): 34-41
- Dym M, Fawcett DW (1970) The blood-testis barrier in the rat and the physiological compartmentation of the seminiferous epithelium. Biol Reprod. 3(3): 308-26
- Setchell BP, Waites GM (1970) Changes in the permeability of the testicular capillaries and of the 'blood-testis barrier' after injection of cadmium chloride in the rat. J Endocrinol. 47(1): 81-6
- Meng J, Greenlee AR, Taub CJ, Braun RE (2011) Sertoli cell-specific deletion of the androgen receptor compromises testicular immune privilege in mice. Biol Reprod. 85(2): 254-60
- McCabe MJ, Allan CM, Foo CF, Nicholls PK, McTavish KJ, Stanton PG (2012) Androgen Initiates Sertoli Cell Tight Junction Formation in the Hypogonadal (hpg) Mouse. Biol Reprod.
- Yan HH, Mruk DD, Cheng CY (2008) Junction restructuring and spermatogenesis: the biology, regulation, and implication in male contraceptive development. Curr Top Dev Biol. 80: 57-92
- Mruk DD, Cheng CY (2015) The Mammalian Blood-Testis Barrier: Its Biology and Regulation. Endocr Rev. 36(5): 564-91
- Gow A, Southwood CM, Li JS, Pariali M, Riordan GP, Brodie SE, Danias J, Bronstein JM, Kachar B, Lazzarini RA (1999) CNS myelin and sertoli cell tight junction strands are absent in Osp/claudin-11 null mice. Cell. 99(6): 649-59
- Hall PF, Mita M (1984) Influence of follicle-stimulating hormone on glucose transport by cultured Sertoli cells. Biol Reprod. 31(5): 863-9
- Jutte NH, Jansen R, Grootegoed JA, Rommerts FF, van der Molen HJ (1983) FSH stimulation of the production of pyruvate and lactate by rat Sertoli cells may be involved in hormonal regulation of spermatogenesis. J Reprod Fertil. 68(1): 219-26
- Robinson R, Fritz IB (1979) Myoinositol biosynthesis by Sertoli cells, and levels of myoinositol biosynthetic enzymes in testis and epididymis. Can J Biochem. 57(6): 962-7
- Kaur G, Thompson LA, Dufour JM (2014) Sertoli cells--immunological sentinels of spermatogenesis. Semin Cell Dev Biol. 30: 36-44
- Rebourcet D, O'Shaughnessy PJ, Monteiro A, Milne L, Cruickshanks L, Jeffrey N, Guillou F, Freeman TC, Mitchell RT, Smith LB (2014) Sertoli cells maintain Leydig cell number and peritubular myoid cell activity in the adult mouse testis. PLoS One. 9(8): e105687
- Hedger MP, Winnall WR (2012) Regulation of activin and inhibin in the adult testis and the evidence for functional roles in spermatogenesis and immunoregulation. Mol Cell Endocrinol. 359(1-2): 30-42
- Nicholls PK, Stanton PG, Chen JL, Olcorn JS, Haverfield JT, Qian H, Walton KL, Gregorevic P, Harrison CA (2012) Activin signaling regulates Sertoli cell differentiation and function. Endocrinology. 153(12): 6065-77
- Haverfield JT, Meachem SJ, Nicholls PK, Rainczuk KE, Simpson ER, Stanton PG (2014) Differential permeability of the blood-testis barrier during reinitiation of spermatogenesis in adult male rats. Endocrinology. 155(3): 1131-44
- Yan W (2015) Gene knockouts that affect Sertoli cell function, in Sertoli cell biology, Griswold, M.D., Editor Elsevier: Waltham, MA. p. 437-469
- Chen C, Ouyang W, Grigura V, et al. (2005) ERM is required for transcriptional control of the spermatogonial stem cell niche. Nature. 436(7053): 1030-4
- Phillips BT, Gassei K, Orwig KE (2010) Spermatogonial stem cell regulation and spermatogenesis. Philos Trans R Soc Lond B Biol Sci. 365(1546): 1663-78
- Simorangkir DR, de Kretser DM, Wreford NG (1995) Increased numbers of Sertoli and germ cells in adult rat testes induced by synergistic action of transient neonatal hypothyroidism and neonatal hemicastration. J Reprod Fertil. 104(2): 207-13
- Simorangkir DR, Wreford NG, De Kretser DM (1997) Impaired germ cell development in the testes of immature rats with neonatal hypothyroidism. J Androl. 18(2): 186-93
- Haverfield JT, Stanton PG, Meachem SJ (2015) Adult Sertoli cell differentiation status in humans., in Sertoli cell biology, Griswold, M.D., Editor Elsevier: Waltham, MA. p. 81-98
- Mazaud-Guittot S, Meugnier E, Pesenti S, Wu X, Vidal H, Gow A, Le Magueresse-Battistoni B (2010) Claudin 11 deficiency in mice results in loss of the Sertoli cell epithelial phenotype in the testis. Biol Reprod. 82(1): 202-13
- Tarulli GA, Stanton PG, Loveland KL, Meyts ER, McLachlan RI, Meachem SJ (2013) A survey of Sertoli cell differentiation in men after gonadotropin suppression and in testicular cancer. Spermatogenesis. 3(1): e24014
- Matson CK, Murphy MW, Sarver AL, Griswold MD, Bardwell VJ, Zarkower D (2011) DMRT1 prevents female reprogramming in the postnatal mammalian testis. Nature. 476(7358): 101-4
- Rebourcet D, O'Shaughnessy PJ, Pitetti JL, et al. (2014) Sertoli cells control peritubular myoid cell fate and support adult Leydig cell development in the prepubertal testis. Development. 141(10): 2139-49
- Cortes D, Muller J, Skakkebaek NE (1987) Proliferation of Sertoli cells during development of the human testis assessed by stereological methods. Int J Androl. 10(4): 589-96
- Yang Q-E, Oatley JM (2015) Early postnatal interactions between Sertoli and germ cells, in Sertoli cell biology, Griswold, M.D., Editor Elsevier: Waltham, MA. p. 81-98
- Bagheri-Fam S, Argentaro A, Svingen T, Combes AN, Sinclair AH, Koopman P, Harley VR (2011) Defective survival of proliferating Sertoli cells and androgen receptor function in a mouse model of the ATR-X syndrome. Hum Mol Genet. 20(11): 2213-24
- Petersen C, Soder O (2006) The Sertoli cell--a hormonal target and 'super' nurse for germ cells that determines testicular size. Horm Res. 66(4): 153-61
- Cooke PS, Hess RA, Porcelli J, Meisami E (1991) Increased sperm production in adult rats after transient neonatal hypothyroidism. Endocrinology. 129(1): 244-8
- Boitani C, Stefanini M, Fragale A, Morena AR (1995) Activin stimulates Sertoli cell proliferation in a defined period of rat testis development. Endocrinology. 136(12): 5438-44
- Meehan T, Schlatt S, O'Bryan MK, de Kretser DM, Loveland KL (2000) Regulation of germ cell and Sertoli cell development by activin, follistatin, and FSH. Dev Biol. 220(2): 225-37
- Sheckter CB, McLachlan RI, Tenover JS, Matsumoto AM, Burger HG, de Kretser DM, Bremner WJ (1988) Stimulation of serum inhibin concentrations by gonadotropin-releasing hormone in men with idiopathic hypogonadotropic hypogonadism. J Clin Endocrinol Metab. 67(6): 1221-4
- Christensen A (1975) Leydig cells, in Handbook of Physiology, Section 7, Endocrinology. p. 57-94
- Fawcett DW, Leak LV, Heidger PM, Jr. (1970) Electron microscopic observations on the structural components of the blood-testis barrier. J Reprod Fertil Suppl. 10: 105-22
- Miller SC (1982) Localization of plutonium-241 in the testis. An interspecies comparison using light and electron microscope autoradiography. Int J Radiat Biol Relat Stud Phys Chem Med. 41(6): 633-43
- Miller SC, Bowman BM, Rowland HG (1983) Structure, cytochemistry, endocytic activity, and immunoglobulin (Fc) receptors of rat testicular interstitial-tissue macrophages. Am J Anat. 168(1): 1-13
- Martin LJ (2016) Cell interactions and genetic regulation that contribute to testicular Leydig cell development and differentiation. Mol Reprod Dev. 83(6): 470-87
- Teerds KJ, Huhtaniemi IT (2015) Morphological and functional maturation of Leydig cells: from rodent models to primates. Hum Reprod Update. 21(3): 310-28
- Lording DW, De Kretser DM (1972) Comparative ultrastructural and histochemical studies of the interstitial cells of the rat testis during fetal and postnatal development. J Reprod Fertil. 29(2): 261-9
- Pelliniemi LJ, Niemi M (1969) Fine structure of the human foetal testis. I. The interstitial tissue. Z Zellforsch Mikrosk Anat. 99(4): 507-22
- Haider SG (2004) Cell biology of Leydig cells in the testis. Int Rev Cytol. 233: 181-241
- Wen Q, Cheng CY, Liu YX (2016) Development, function and fate of fetal Leydig cells. Semin Cell Dev Biol. 59: 89-98
- Huhtaniemi I (1977) Studies on steroidogenesis and its regulation in human fetal adrenal and testis. J Steroid Biochem. 8(5): 491-7
- Prince FP (1990) Ultrastructural evidence of mature Leydig cells and Leydig cell regression in the neonatal human testis. Anat Rec. 228(4): 405-17
- Shima Y, Matsuzaki S, Miyabayashi K, Otake H, Baba T, Kato S, Huhtaniemi I, Morohashi K (2015) Fetal Leydig Cells Persist as an Androgen-Independent Subpopulation in the Postnatal Testis. Mol Endocrinol. 29(11): 1581-93
- Christensen AK, Peacock KC (1980) Increase in Leydig cell number in testes of adult rats treated chronically with an excess of human chorionic gonadotropin. Biol Reprod. 22(2): 383-91
- Prince FP (2001) The triphasic nature of Leydig cell development in humans, and comments on nomenclature. J Endocrinol. 168(2): 213-6
- O'Shaughnessy PJ, Baker PJ, Johnston H (2006) The foetal Leydig cell-- differentiation, function and regulation. Int J Androl. 29(1): 90-5; discussion 105-8
- Bitgood MJ, Shen L, McMahon AP (1996) Sertoli cell signaling by Desert hedgehog regulates the male germline. Curr Biol. 6(3): 298-304
- Brokken LJ, Adamsson A, Paranko J, Toppari J (2009) Antiandrogen exposure in utero disrupts expression of desert hedgehog and insulin-like factor 3 in the developing fetal rat testis. Endocrinology. 150(1): 445-51
- Clark AM, Garland KK, Russell LD (2000) Desert hedgehog (Dhh) gene is required in the mouse testis for formation of adult-type Leydig cells and normal development of peritubular cells and seminiferous tubules. Biol Reprod. 63(6): 1825-38
- Li L, Wang Y, Li X, Liu S, Wang G, Lin H, Zhu Q, Guo J, Chen H, Ge HS, Ge RS (2016) Regulation of development of rat stem and progenitor Leydig cells by activin. Andrology.
- Odeh HM, Kleinguetl C, Ge R, Zirkin BR, Chen H (2014) Regulation of the proliferation and differentiation of Leydig stem cells in the adult testis. Biol Reprod. 90(6): 123
- Pierucci-Alves F, Clark AM, Russell LD (2001) A developmental study of the Desert hedgehog-null mouse testis. Biol Reprod. 65(5): 1392-402
- Canto P, Soderlund D, Reyes E, Mendez JP (2004) Mutations in the desert hedgehog (DHH) gene in patients with 46,XY complete pure gonadal dysgenesis. J Clin Endocrinol Metab. 89(9): 4480-3
- Wen Q, Zheng QS, Li XX, Hu ZY, Gao F, Cheng CY, Liu YX (2014) Wt1 dictates the fate of fetal and adult Leydig cells during development in the mouse testis. Am J Physiol Endocrinol Metab. 307(12): E1131-43
- Umehara T, Kawashima I, Kawai T, Hoshino Y, Morohashi KI, Shima Y, Zeng W, Richards JS, Shimada M (2016) Neuregulin 1 Regulates Proliferation of Leydig Cells to Support Spermatogenesis and Sexual Behavior in Adult Mice. Endocrinology. 157(12): 4899-4913
- Payne AH, Hardy MP, Russell LD (1996) The Leydig Cell Illinois: Cache River Press. 1-802
- Foresta C, Bettella A, Vinanzi C, Dabrilli P, Meriggiola MC, Garolla A, Ferlin A (2004) A novel circulating hormone of testis origin in humans. J Clin Endocrinol Metab. 89(12): 5952-8
- Zimmermann S, Steding G, Emmen JM, Brinkmann AO, Nayernia K, Holstein AF, Engel W, Adham IM (1999) Targeted disruption of the Insl3 gene causes bilateral cryptorchidism. Mol Endocrinol. 13(5): 681-91
- Ivell R, Wade JD, Anand-Ivell R (2013) INSL3 as a biomarker of Leydig cell functionality. Biol Reprod. 88(6): 147
- Anand-Ivell RJ, Relan V, Balvers M, Coiffec-Dorval I, Fritsch M, Bathgate RA, Ivell R (2006) Expression of the insulin-like peptide 3 (INSL3) hormone-receptor (LGR8) system in the testis. Biol Reprod. 74(5): 945-53
- Kawamura K, Kumagai J, Sudo S, Chun SY, Pisarska M, Morita H, Toppari J, Fu P, Wade JD, Bathgate RA, Hsueh AJ (2004) Paracrine regulation of mammalian oocyte maturation and male germ cell survival. Proc Natl Acad Sci U S A. 101(19): 7323-8
- Yuan FP, Li X, Lin J, Schwabe C, Bullesbach EE, Rao CV, Lei ZM (2010) The role of RXFP2 in mediating androgen-induced inguinoscrotal testis descent in LH receptor knockout mice. Reproduction. 139(4): 759-69
- Pathirana IN, Kawate N, Bullesbach EE, Takahashi M, Hatoya S, Inaba T, Tamada H (2012) Insulin-like peptide 3 stimulates testosterone secretion in mouse Leydig cells via cAMP pathway. Regul Pept. 178(1-3): 102-6
- Johansen ML, Anand-Ivell R, Mouritsen A, Hagen CP, Mieritz MG, Soeborg T, Johannsen TH, Main KM, Andersson AM, Ivell R, Juul A (2014) Serum levels of insulin-like factor 3, anti-Mullerian hormone, inhibin B, and testosterone during pubertal transition in healthy boys: a longitudinal pilot study. Reproduction. 147(4): 529-35
- Trabado S, Maione L, Bry-Gauillard H, et al. (2014) Insulin-like peptide 3 (INSL3) in men with congenital hypogonadotropic hypogonadism/Kallmann syndrome and effects of different modalities of hormonal treatment: a single-center study of 281 patients. J Clin Endocrinol Metab. 99(2): E268-75
- Rohayem J, Fricke R, Czeloth K, Mallidis C, Wistuba J, Krallmann C, Zitzmann M, Kliesch S (2015) Age and markers of Leydig cell function, but not of Sertoli cell function predict the success of sperm retrieval in adolescents and adults with Klinefelter's syndrome. Andrology. 3(5): 868-75
- Stanley E, Lin CY, Jin S, Liu J, Sottas CM, Ge R, Zirkin BR, Chen H (2012) Identification, Proliferation, and Differentiation of Adult Leydig Stem Cells. Endocrinology. DOI 10.1210/en.2012-1417
- Baird DT, Galbraith A, Fraser IS, Newsam JE (1973) The concentration of oestrone and oestradiol-17 in spermatic venous blood in man. J Endocrinol. 57(2): 285-8
- Rochira V, Madeo B, Diazzi C, Zirilli L, Santi D, Carani C. (2016) Estrogens and Male Reproduction, in www.ENDOTEXT.org, Endocrinology of Male Reproduction, Section Editor McLachlan R.I. MDTEXT.COM,INC, : South Dartmouth,MA 02748.
- Handelsman DJ. (2016) Androgen Physiology, Pharmacology and Abuse in www.ENDOTEXT.org, Endocrinology of Male Reproduction, Section Editor McLachlan R.I. MDTEXT.COM,INC, : South Dartmouth,MA 02748.
- Hodgson YM, de Kretser DM (1984) Acute responses of Leydig cells to hCG: evidence for early hypertrophy of Leydig cells. Mol Cell Endocrinol. 35(2-3): 75-82
- Waterman MR, Simpson ER (1989) Regulation of steroid hydroxylase gene expression is multifactorial in nature. Recent Prog Horm Res. 45: 533-63; discussion 563-6
- Wu FC, Irby DC, Clarke IJ, Cummins JT, de Kretser DM (1987) Effects of gonadotropin-releasing hormone pulse-frequency modulation on luteinizing hormone, follicle-stimulating hormone and testosterone secretion in hypothalamo/pituitary-disconnected rams. Biol Reprod. 37(3): 501-10
- Beattie MC, Adekola L, Papadopoulos V, Chen H, Zirkin BR (2015) Leydig cell aging and hypogonadism. Exp Gerontol. 68: 87-91
- Veldhuis JD, Liu PY, Keenan DM, Takahashi PY (2012) Older men exhibit reduced efficacy of and heightened potency downregulation by intravenous pulses of recombinant human LH: a study in 92 healthy men. Am J Physiol Endocrinol Metab. 302(1): E117-22
- Oury F, Sumara G, Sumara O, Ferron M, Chang H, Smith CE, Hermo L, Suarez S, Roth BL, Ducy P, Karsenty G (2011) Endocrine regulation of male fertility by the skeleton. Cell. 144(5): 796-809
- Karsenty G (2012) The mutual dependence between bone and gonads. J Endocrinol. 213(2): 107-14
- Karsenty G, Oury F (2012) Biology without walls: the novel endocrinology of bone. Annu Rev Physiol. 74: 87-105
- Aoki A, Fawcett DW (1978) Is there a local feedback from the seminiferous tubules affecting activity of the Leydig cells? Biol Reprod. 19(1): 144-58
- de Kretser DM (1987) Local regulation of testicular function. Int Rev Cytol. 109: 89-112
- O'Shaughnessy PJ, Morris ID, Huhtaniemi I, Baker PJ, Abel MH (2009) Role of androgen and gonadotrophins in the development and function of the Sertoli cells and Leydig cells: data from mutant and genetically modified mice. Mol Cell Endocrinol. 306(1-2): 2-8
- De Gendt K, Atanassova N, Tan KA, et al. (2005) Development and function of the adult generation of Leydig cells in mice with Sertoli cell-selective or total ablation of the androgen receptor. Endocrinology. 146(9): 4117-26
- Hazra R, Jimenez M, Desai R, Handelsman DJ, Allan CM (2013) Sertoli cell androgen receptor expression regulates temporal fetal and adult Leydig cell differentiation, function, and population size. Endocrinology. 154(9): 3410-22
- O'Shaughnessy PJ, Hu L, Baker PJ (2008) Effect of germ cell depletion on levels of specific mRNA transcripts in mouse Sertoli cells and Leydig cells. Reproduction. 135(6): 839-50
- Jegou B, Laws AO, de Kretser DM (1984) Changes in testicular function induced by short-term exposure of the rat testis to heat: further evidence for interaction of germ cells, Sertoli cells and Leydig cells. Int J Androl. 7(3): 244-57
- Lue Y, Hikim AP, Wang C, Im M, Leung A, Swerdloff RS (2000) Testicular heat exposure enhances the suppression of spermatogenesis by testosterone in rats: the "two-hit" approach to male contraceptive development. Endocrinology. 141(4): 1414-24
- Smith LB, O'Shaughnessy PJ, Rebourcet D (2015) Cell-specific ablation in the testis: what have we learned? Andrology. 3(6): 1035-49
- Risbridger GP, Kerr JB, de Kretser DM (1981) Evaluation of Leydig cell function and gonadotropin binding in unilateral and bilateral cryptorchidism; evidence for local control of Leydig cell function by the seminiferous tubule. Biol Reprod. 24(3): 534-40
- Risbridger GP, Kerr JB, Peake RA, de Kretser DM (1981) An assessment of Leydig cell function after bilateral or unilateral efferent duct ligation: further evidence for local control of Leydig cell function. Endocrinology. 109(4): 1234-41
- Andersson AM, Jorgensen N, Frydelund-Larsen L, Rajpert-De Meyts E, Skakkebaek NE (2004) Impaired Leydig cell function in infertile men: a study of 357 idiopathic infertile men and 318 proven fertile controls. J Clin Endocrinol Metab. 89(7): 3161-7
- van den Driesche S, Kolovos P, Platts S, Drake AJ, Sharpe RM (2012) Inter-relationship between testicular dysgenesis and Leydig cell function in the masculinization programming window in the rat. PLoS One. 7(1): e30111
- Hales DB (2002) Testicular macrophage modulation of Leydig cell steroidogenesis. J Reprod Immunol. 57(1-2): 3-18
- Welsh M, Moffat L, Belling K, de Franca LR, Segatelli TM, Saunders PT, Sharpe RM, Smith LB (2012) Androgen receptor signalling in peritubular myoid cells is essential for normal differentiation and function of adult Leydig cells. Int J Androl. 35(1): 25-40
- Clermont Y (1958) Contractile elements in the limiting membrane of the seminiferous tubules of the rat. Exp Cell Res. 15(2): 438-40
- Ross MH, Long IR (1966) Contractile cells in human seminiferous tubules. Science. 153(3741): 1271-3
- Maekawa M, Kamimura K, Nagano T (1996) Peritubular myoid cells in the testis: their structure and function. Arch Histol Cytol. 59(1): 1-13
- Rossi F, Ferraresi A, Romagni P, Silvestroni L, Santiemma V (2002) Angiotensin II stimulates contraction and growth of testicular peritubular myoid cells in vitro. Endocrinology. 143(8): 3096-104
- Tripiciano A, Filippini A, Ballarini F, Palombi F (1998) Contractile response of peritubular myoid cells to prostaglandin F2alpha. Mol Cell Endocrinol. 138(1-2): 143-50
- Tripiciano A, Filippini A, Giustiniani Q, Palombi F (1996) Direct visualization of rat peritubular myoid cell contraction in response to endothelin. Biol Reprod. 55(1): 25-31
- Losinno AD, Sorrivas V, Ezquer M, Ezquer F, Lopez LA, Morales A (2016) Changes of myoid and endothelial cells in the peritubular wall during contraction of the seminiferous tubule. Cell Tissue Res. 365(2): 425-35
- de Winter JP, Vanderstichele HM, Verhoeven G, Timmerman MA, Wesseling JG, de Jong FH (1994) Peritubular myoid cells from immature rat testes secrete activin-A and express activin receptor type II in vitro. Endocrinology. 135(2): 759-67
- Gnessi L, Emidi A, Jannini EA, Carosa E, Maroder M, Arizzi M, Ulisse S, Spera G (1995) Testicular development involves the spatiotemporal control of PDGFs and PDGF receptors gene expression and action. J Cell Biol. 131(4): 1105-21
- Verhoeven G, Hoeben E, De Gendt K (2000) Peritubular cell-Sertoli cell interactions: factors involved in PmodS activity. Andrologia. 32(1): 42-5
- Welsh M, Saunders PT, Atanassova N, Sharpe RM, Smith LB (2009) Androgen action via testicular peritubular myoid cells is essential for male fertility. FASEB J. 23(12): 4218-30
- Qian Y, Liu S, Guan Y, et al. (2013) Lgr4-mediated Wnt/beta-catenin signaling in peritubular myoid cells is essential for spermatogenesis. Development. 140(8): 1751-61
- Chen LY, Brown PR, Willis WB, Eddy EM (2014) Peritubular myoid cells participate in male mouse spermatogonial stem cell maintenance. Endocrinology. 155(12): 4964-74
- Chen LY, Willis WD, Eddy EM (2016) Targeting the Gdnf Gene in peritubular myoid cells disrupts undifferentiated spermatogonial cell development. Proc Natl Acad Sci U S A. 113(7): 1829-34
- Griswold MD (2015) ed.^eds. Sertoli cell biology. 2nd ed. Elsevier: Waltham, MA
- Lin YT, Capel B (2015) Cell fate commitment during mammalian sex determination. Curr Opin Genet Dev. 32: 144-52
- Yao HH, Ungewitter E, Franco H, Capel B (2015) Establishment of fetal Sertoli cells and their role in testis morphogenesis, in Sertoli cell biology, Griswold, M.D., Editor Elsevier: Waltham, MA. p. 57-80
- Ohta K, Yamamoto M, Lin Y, Hogg N, Akiyama H, Behringer RR, Yamazaki Y (2012) Male differentiation of germ cells induced by embryonic age-specific Sertoli cells in mice. Biol Reprod. 86(4): 112
- Van Haaster LH, De Jong FH, Docter R, De Rooij DG (1992) The effect of hypothyroidism on Sertoli cell proliferation and differentiation and hormone levels during testicular development in the rat. Endocrinology. 131(3): 1574-6
- Hazra R, Corcoran L, Robson M, McTavish KJ, Upton D, Handelsman DJ, Allan CM (2013) Temporal role of Sertoli cell androgen receptor expression in spermatogenic development. Mol Endocrinol. 27(1): 12-24
- Meachem SJ, McLachlan RI, de Kretser DM, Robertson DM, Wreford NG (1996) Neonatal exposure of rats to recombinant follicle stimulating hormone increases adult Sertoli and spermatogenic cell numbers. Biol Reprod. 54(1): 36-44
- Fahrioglu U, Murphy MW, Zarkower D, Bardwell VJ (2007) mRNA expression analysis and the molecular basis of neonatal testis defects in Dmrt1 mutant mice. Sex Dev. 1(1): 42-58
- Welborn JP, Davis MG, Ebers SD, Stodden GR, Hayashi K, Cheatwood JL, Rao MK, MacLean JA, 2nd (2015) Rhox8 Ablation in the Sertoli Cells Using a Tissue-Specific RNAi Approach Results in Impaired Male Fertility in Mice. Biol Reprod. 93(1): 8
- Hess RA, Vogl AW (2015) Sertoli cell anatomy and cytoskeleton, in Sertoli cell biology, Griswold, M.D., Editor Elsevier: Waltham, MA. p. 1-56
- Zimmermann C, Stevant I, Borel C, Conne B, Pitetti JL, Calvel P, Kaessmann H, Jegou B, Chalmel F, Nef S (2015) Research resource: the dynamic transcriptional profile of sertoli cells during the progression of spermatogenesis. Mol Endocrinol. 29(4): 627-42
- Hogarth CA (2015) Retinoic acid metabolism, signalling and function in the adult testis, in Sertoli cell biology, Griswold, M.D., Editor Elsevier: Waltham, MA. p. 247-272
- Vernet N, Dennefeld C, Rochette-Egly C, Oulad-Abdelghani M, Chambon P, Ghyselinck NB, Mark M (2006) Retinoic acid metabolism and signaling pathways in the adult and developing mouse testis. Endocrinology. 147(1): 96-110
- Hogarth CA, Arnold S, Kent T, Mitchell D, Isoherranen N, Griswold MD (2015) Processive pulses of retinoic acid propel asynchronous and continuous murine sperm production. Biol Reprod. 92(2): 37
- Kent T, Arnold SL, Fasnacht R, Rowsey R, Mitchell D, Hogarth CA, Isoherranen N, Griswold MD (2016) ALDH Enzyme Expression Is Independent of the Spermatogenic Cycle, and Their Inhibition Causes Misregulation of Murine Spermatogenic Processes. Biol Reprod. 94(1): 12
- Vernet N, Dennefeld C, Klopfenstein M, Ruiz A, Bok D, Ghyselinck NB, Mark M (2008) Retinoid X receptor beta (RXRB) expression in Sertoli cells controls cholesterol homeostasis and spermiation. Reproduction. 136(5): 619-26
- Hasegawa K, Saga Y (2012) Retinoic acid signaling in Sertoli cells regulates organization of the blood-testis barrier through cyclical changes in gene expression. Development. 139(23): 4347-55
- Nicholls PK, Harrison CA, Rainczuk KE, Wayne Vogl A, Stanton PG (2013) Retinoic acid promotes Sertoli cell differentiation and antagonises activin-induced proliferation. Mol Cell Endocrinol. 377(1-2): 33-43
- O'Shaughnessy PJ (2014) Hormonal control of germ cell development and spermatogenesis. Semin Cell Dev Biol. 29: 55-65
- Smith LB, Walker WH, O'Donnell L (2015) Hormonal regulation of spermatogenesis through Sertoli cells by androgens and estrogens, in Sertoli cell biology, Griswold, M.D., Editor Elsevier: Waltham, MA. p. 175-200
- O'Donnell L, Meachem SJ, Stanton PG, McLachlan RI (2006) Endocrine regulation of spermatogenesis, in Knobil and Neill's Physiology of Reproduction, Neill, J.D., Editor Elsevier: San Diego, CA. p. 1017-1069
- Ruwanpura SM, McLachlan RI, Meachem SJ (2010) Hormonal regulation of male germ cell development. J Endocrinol. 205(2): 117-31
- Pitetti JL, Calvel P, Romero Y, Conne B, Truong V, Papaioannou MD, Schaad O, Docquier M, Herrera PL, Wilhelm D, Nef S (2013) Insulin and IGF1 receptors are essential for XX and XY gonadal differentiation and adrenal development in mice. PLoS Genet. 9(1): e1003160
- Rodriguez I, Ody C, Araki K, Garcia I, Vassalli P (1997) An early and massive wave of germinal cell apoptosis is required for the development of functional spermatogenesis. EMBO J. 16(9): 2262-70
- Wreford NG, Rajendra Kumar T, Matzuk MM, de Kretser DM (2001) Analysis of the testicular phenotype of the follicle-stimulating hormone beta-subunit knockout and the activin type II receptor knockout mice by stereological analysis. Endocrinology. 142(7): 2916-20
- Grover A, Sairam MR, Smith CE, Hermo L (2004) Structural and functional modifications of Sertoli cells in the testis of adult follicle-stimulating hormone receptor knockout mice. Biol Reprod. 71(1): 117-29
- Matthiesson KL, McLachlan RI, O'Donnell L, Frydenberg M, Robertson DM, Stanton PG, Meachem SJ (2006) The relative roles of follicle-stimulating hormone and luteinizing hormone in maintaining spermatogonial maturation and spermiation in normal men. J Clin Endocrinol Metab. 91(10): 3962-9
- Nieschlag E, Simoni M, Gromoll J, Weinbauer GF (1999) Role of FSH in the regulation of spermatogenesis: clinical aspects. Clin Endocrinol (Oxf). 51(2): 139-46
- Simoni M, Weinbauer GF, Gromoll J, Nieschlag E (1999) Role of FSH in male gonadal function. Ann Endocrinol (Paris). 60(2): 102-6
- Walker WH (2010) Non-classical actions of testosterone and spermatogenesis. Philos Trans R Soc Lond B Biol Sci. 365(1546): 1557-69
- Toocheck C, Clister T, Shupe J, Crum C, Ravindranathan P, Lee TK, Ahn JM, Raj GV, Sukhwani M, Orwig KE, Walker WH (2016) Mouse Spermatogenesis Requires Classical and Nonclassical Testosterone Signaling. Biol Reprod. 94(1): 11
- Chang C, Chen YT, Yeh SD, Xu Q, Wang RS, Guillou F, Lardy H, Yeh S (2004) Infertility with defective spermatogenesis and hypotestosteronemia in male mice lacking the androgen receptor in Sertoli cells. Proc Natl Acad Sci U S A. 101(18): 6876-81
- De Gendt K, Swinnen JV, Saunders PT, et al. (2004) A Sertoli cell-selective knockout of the androgen receptor causes spermatogenic arrest in meiosis. Proc Natl Acad Sci U S A. 101(5): 1327-32
- De Gendt K, Verhoeven G, Amieux PS, Wilkinson MF (2014) Genome-wide identification of AR-regulated genes translated in Sertoli cells in vivo using the RiboTag approach. Mol Endocrinol. 28(4): 575-91
- Abel MH, Baker PJ, Charlton HM, Monteiro A, Verhoeven G, De Gendt K, Guillou F, O'Shaughnessy PJ (2008) Spermatogenesis and Sertoli cell activity in mice lacking Sertoli cell receptors for follicle-stimulating hormone and androgen. Endocrinology. 149(7): 3279-85
- Saito K, O'Donnell L, McLachlan RI, Robertson DM (2000) Spermiation failure is a major contributor to early spermatogenic suppression caused by hormone withdrawal in adult rats. Endocrinology. 141(8): 2779-85
- Walker WH, Cheng J (2005) FSH and testosterone signaling in Sertoli cells. Reproduction. 130(1): 15-28
- Nicholls PK, Harrison CA, Walton KL, McLachlan RI, O'Donnell L, Stanton PG (2011) Hormonal regulation of sertoli cell micro-RNAs at spermiation. Endocrinology. 152(4): 1670-83
- Song HW, Wilkinson MF (2014) Transcriptional control of spermatogonial maintenance and differentiation. Semin Cell Dev Biol. 30: 14-26
- Manku G, Culty M (2015) Mammalian gonocyte and spermatogonia differentiation: recent advances and remaining challenges. Reproduction. 149(3): R139-57
- de Rooij DG (2009) The spermatogonial stem cell niche. Microsc Res Tech. 72(8): 580-5
- Oatley MJ, Racicot KE, Oatley JM (2011) Sertoli cells dictate spermatogonial stem cell niches in the mouse testis. Biol Reprod. 84(4): 639-45
- Giuili G, Tomljenovic A, Labrecque N, Oulad-Abdelghani M, Rassoulzadegan M, Cuzin F (2002) Murine spermatogonial stem cells: targeted transgene expression and purification in an active state. EMBO Rep. 3(8): 753-9
- Meng X, Lindahl M, Hyvonen ME, et al. (2000) Regulation of cell fate decision of undifferentiated spermatogonia by GDNF. Science. 287(5457): 1489-93
- Nagano M, Ryu BY, Brinster CJ, Avarbock MR, Brinster RL (2003) Maintenance of mouse male germ line stem cells in vitro. Biol Reprod. 68(6): 2207-14
- Shinohara T, Avarbock MR, Brinster RL (1999) beta1- and alpha6-integrin are surface markers on mouse spermatogonial stem cells. Proc Natl Acad Sci U S A. 96(10): 5504-9
- Yomogida K, Yagura Y, Tadokoro Y, Nishimune Y (2003) Dramatic expansion of germinal stem cells by ectopically expressed human glial cell line-derived neurotrophic factor in mouse Sertoli cells. Biol Reprod. 69(4): 1303-7
- Oatley JM, Oatley MJ, Avarbock MR, Tobias JW, Brinster RL (2009) Colony stimulating factor 1 is an extrinsic stimulator of mouse spermatogonial stem cell self-renewal. Development. 136(7): 1191-9
- DeFalco T, Potter SJ, Williams AV, Waller B, Kan MJ, Capel B (2015) Macrophages Contribute to the Spermatogonial Niche in the Adult Testis. Cell Rep. 12(7): 1107-19
- Loveland KL, Schlatt S (1997) Stem cell factor and c-kit in the mammalian testis: lessons originating from Mother Nature's gene knockouts. J Endocrinol. 153(3): 337-44
- Vincent S, Segretain D, Nishikawa S, Nishikawa SI, Sage J, Cuzin F, Rassoulzadegan M (1998) Stage-specific expression of the Kit receptor and its ligand (KL) during male gametogenesis in the mouse: a Kit-KL interaction critical for meiosis. Development. 125(22): 4585-93
- Blume-Jensen P, Jiang G, Hyman R, Lee KF, O'Gorman S, Hunter T (2000) Kit/stem cell factor receptor-induced activation of phosphatidylinositol 3'-kinase is essential for male fertility. Nat Genet. 24(2): 157-62
- Hogarth CA, Griswold MD (2010) The key role of vitamin A in spermatogenesis. J Clin Invest. 120(4): 956-62
- Whitmore LS, Ye P (2015) Dissecting Germ Cell Metabolism through Network Modeling. PLoS One. 10(9): e0137607
- Griswold MD (2015) The initiation of spermatogenesis and the cycle of the seminiferous
epithelium, in Sertoli Cell Biology, Griswold, M.D., Editor Elsevier: Waltham, MA. p. 233-246
- 238.
Ikami K, Tokue M, Sugimoto R, Noda C, Kobayashi S, Hara K, Yoshida S (2015) Hierarchical differentiation competence in response to retinoic acid ensures stem cell maintenance during mouse spermatogenesis. Development. 142(9): 1582-92
- 239.
Busada JT, Chappell VA, Niedenberger BA, Kaye EP, Keiper BD, Hogarth CA, Geyer CB (2015) Retinoic acid regulates Kit translation during spermatogonial differentiation in the mouse. Dev Biol. 397(1): 140-9
- 240.
Busada JT, Niedenberger BA, Velte EK, Keiper BD, Geyer CB (2015) Mammalian target of rapamycin complex 1 (mTORC1) Is required for mouse spermatogonial differentiation in vivo. Dev Biol. DBIO15155
- 241.
McLachlan RI, O'Donnell L, Meachem SJ, Stanton PG, de Kretser DM, Pratis K, Robertson DM (2002) Identification of specific sites of hormonal regulation in spermatogenesis in rats, monkeys, and man. Recent Prog Horm Res. 57: 149-79
- 242.
Schlatt S, Ehmcke J (2014) Regulation of spermatogenesis: an evolutionary biologist's perspective. Semin Cell Dev Biol. 29: 2-16
- 243.
Haywood M, Spaliviero J, Jimemez M, King NJ, Handelsman DJ, Allan CM (2003) Sertoli and germ cell development in hypogonadal (hpg) mice expressing transgenic follicle-stimulating hormone alone or in combination with testosterone. Endocrinology. 144(2): 509-17
- 244.
Shetty G, Wilson G, Huhtaniemi I, Boettger-Tong H, Meistrich ML (2001) Testosterone inhibits spermatogonial differentiation in juvenile spermatogonial depletion mice. Endocrinology. 142(7): 2789-95
- 245.
Marshall GR, Zorub DS, Plant TM (1995) Follicle-stimulating hormone amplifies the population of differentiated spermatogonia in the hypophysectomized testosterone-replaced adult rhesus monkey (Macaca mulatta). Endocrinology. 136(8): 3504-11
- 246.
Weinbauer GF, Behre HM, Fingscheidt U, Nieschlag E (1991) Human follicle-stimulating hormone exerts a stimulatory effect on spermatogenesis, testicular size, and serum inhibin levels in the gonadotropin-releasing hormone antagonist-treated nonhuman primate (Macaca fascicularis). Endocrinology. 129(4): 1831-9
- 247.
De Rooij DG (2015) The spermatogonial stem cell niche in mammals, in Sertoli Cell Biology, Griswold, M.D., Editor Elsevier: Waltham, MA. p. 99-122
- 248.
Tanaka T, Kanatsu-Shinohara M, Lei Z, Rao CV, Shinohara T (2016) The Luteinizing Hormone-Testosterone Pathway Regulates Mouse Spermatogonial Stem Cell Self-Renewal by Suppressing WNT5A Expression in Sertoli Cells. Stem Cell Reports. 7(2): 279-91
- 249.
Anderson EL, Baltus AE, Roepers-Gajadien HL, Hassold TJ, de Rooij DG, van Pelt AM, Page DC (2008) Stra8 and its inducer, retinoic acid, regulate meiotic initiation in both spermatogenesis and oogenesis in mice. Proc Natl Acad Sci U S A. 105(39): 14976-80
- 250.
Mark M, Jacobs H, Oulad-Abdelghani M, Dennefeld C, Feret B, Vernet N, Codreanu CA, Chambon P, Ghyselinck NB (2008) STRA8-deficient spermatocytes initiate, but fail to complete, meiosis and undergo premature chromosome condensation. J Cell Sci. 121(Pt 19): 3233-42
- 251.
Koubova J, Hu YC, Bhattacharyya T, Soh YQ, Gill ME, Goodheart ML, Hogarth CA, Griswold MD, Page DC (2014) Retinoic acid activates two pathways required for meiosis in mice. PLoS Genet. 10(8): e1004541
- 252.
Abby E, Tourpin S, Ribeiro J, et al. (2016) Implementation of meiosis prophase I programme requires a conserved retinoid-independent stabilizer of meiotic transcripts. Nat Commun. 7: 10324
- 253.
Hermo L, Pelletier RM, Cyr DG, Smith CE (2010) Surfing the wave, cycle, life history, and genes/proteins expressed by testicular germ cells. Part 1: background to spermatogenesis, spermatogonia, and spermatocytes. Microsc Res Tech. 73(4): 241-78
- 254.
Morelli MA, Cohen PE (2005) Not all germ cells are created equal: aspects of sexual dimorphism in mammalian meiosis. Reproduction. 130(6): 761-81
- 255.
Sanderson ML, Hassold TJ, Carrell DT (2008) Proteins involved in meiotic recombination: a role in male infertility? Syst Biol Reprod Med. 54(2): 57-74
- 256.
Baker SM, Bronner CE, Zhang L, et al. (1995) Male mice defective in the DNA mismatch repair gene PMS2 exhibit abnormal chromosome synapsis in meiosis. Cell. 82(2): 309-19
- 257.
Zhu D, Dix DJ, Eddy EM (1997) HSP70-2 is required for CDC2 kinase activity in meiosis I of mouse spermatocytes. Development. 124(15): 3007-14
- 258.
Alekseev OM, Richardson RT, O'Rand MG (2009) Linker histones stimulate HSPA2 ATPase activity through NASP binding and inhibit CDC2/Cyclin B1 complex formation during meiosis in the mouse. Biol Reprod. 81(4): 739-48
- 259.
Eto K, Shiotsuki M, Abe S (2013) Nociceptin induces Rec8 phosphorylation and meiosis in postnatal murine testes. Endocrinology. 154(8): 2891-9
- 260.
Eto K (2015) Nociceptin and meiosis during spermatogenesis in postnatal testes. Vitam Horm. 97: 167-86
- 261.
Bolcun-Filas E, Bannister LA, Barash A, Schimenti KJ, Hartford SA, Eppig JJ, Handel MA, Shen L, Schimenti JC (2011) A-MYB (MYBL1) transcription factor is a master regulator of male meiosis. Development. 138(15): 3319-30
- 262.
O'Hara L, Smith LB (2015) Androgen receptor roles in spermatogenesis and infertility. Best Pract Res Clin Endocrinol Metab. 29(4): 595-605
- 263.
Handelsman DJ (2011) Hormonal regulation of spermatogenesis: insights from constructing genetic models. Reprod Fertil Dev. 23(4): 507-19
- 264.
El Shennawy A, Gates RJ, Russell LD (1998) Hormonal regulation of spermatogenesis in the hypophysectomized rat: cell viability after hormonal replacement in adults after intermediate periods of hypophysectomy. J Androl. 19(3): 320-34; discussion 341-2
- 265.
Chalmel F, Rolland AD, Niederhauser-Wiederkehr C, Chung SS, Demougin P, Gattiker A, Moore J, Patard JJ, Wolgemuth DJ, Jegou B, Primig M (2007) The conserved transcriptome in human and rodent male gametogenesis. Proc Natl Acad Sci U S A. 104(20): 8346-51
- 266.
Elliott DJ, Grellscheid SN (2006) Alternative RNA splicing regulation in the testis. Reproduction. 132(6): 811-9
- 267.
Foulkes NS, Mellstrom B, Benusiglio E, Sassone-Corsi P (1992) Developmental switch of CREM function during spermatogenesis: from antagonist to activator. Nature. 355(6355): 80-4
- 268.
Mandel CR, Bai Y, Tong L (2008) Protein factors in pre-mRNA 3'-end processing. Cell Mol Life Sci. 65(7-8): 1099-122
- 269.
MacDonald CC, McMahon KW (2010) Tissue-specific mechanisms of alternative polyadenylation: testis, brain, and beyond. Wiley Interdiscip Rev RNA. 1(3): 494-501
- 270.
Li W, Park JY, Zheng D, Hoque M, Yehia G, Tian B (2016) Alternative cleavage and polyadenylation in spermatogenesis connects chromatin regulation with post-transcriptional control. BMC Biol. 14(1): 6
- 271.
Hogeveen KN, Sassone-Corsi P (2006) Regulation of gene expression in post-meiotic male germ cells: CREM-signalling pathways and male fertility. Hum Fertil (Camb). 9(2): 73-9
- 272.
Macho B, Brancorsini S, Fimia GM, Setou M, Hirokawa N, Sassone-Corsi P (2002) CREM-dependent transcription in male germ cells controlled by a kinesin. Science. 298(5602): 2388-90
- 273.
Zhang D, Penttila TL, Morris PL, Teichmann M, Roeder RG (2001) Spermiogenesis deficiency in mice lacking the Trf2 gene. Science. 292(5519): 1153-5
- 274.
Wu Y, Hu X, Li Z, et al. (2016) Transcription Factor RFX2 Is a Key Regulator of Mouse Spermiogenesis. Sci Rep. 6: 20435
- 275.
Kleene KC (2003) Patterns, mechanisms, and functions of translation regulation in mammalian spermatogenic cells. Cytogenet Genome Res. 103(3-4): 217-24
- 276.
Cullinane DL, Chowdhury TA, Kleene KC (2015) Mechanisms of translational repression of the Smcp mRNA in round spermatids. Reproduction. 149(1): 43-54
- 277.
Kleene KC (2016) Position-dependent interactions of Y-box protein 2 (YBX2) with mRNA enable mRNA storage in round spermatids by repressing mRNA translation and blocking translation-dependent mRNA decay. Mol Reprod Dev.
- 278.
Ren D, Navarro B, Perez G, Jackson AC, Hsu S, Shi Q, Tilly JL, Clapham DE (2001) A sperm ion channel required for sperm motility and male fertility. Nature. 413(6856): 603-9
- 279.
Escudier E, Duquesnoy P, Papon JF, Amselem S (2009) Ciliary defects and genetics of primary ciliary dyskinesia. Paediatr Respir Rev. 10(2): 51-4
- 280.
Chung SS, Wang X, Wolgemuth DJ (2009) Expression of retinoic acid receptor alpha in the germline is essential for proper cellular association and spermiogenesis during spermatogenesis. Development. 136(12): 2091-100
- 281.
Holdcraft RW, Braun RE (2004) Androgen receptor function is required in Sertoli cells for the terminal differentiation of haploid spermatids. Development. 131(2): 459-67
- 282.
O'Donnell L, McLachlan RI, Wreford NG, Robertson DM (1994) Testosterone promotes the conversion of round spermatids between stages VII and VIII of the rat spermatogenic cycle. Endocrinology. 135(6): 2608-14
- 283.
McLachlan RI, O'Donnell L, Stanton PG, Balourdos G, Frydenberg M, de Kretser DM, Robertson DM (2002) Effects of testosterone plus medroxyprogesterone acetate on semen quality, reproductive hormones, and germ cell populations in normal young men. J Clin Endocrinol Metab. 87(2): 546-56
- 284.
Heller CG, Clermont Y (1963) Spermatogenesis in man: an estimate of its duration. Science. 140(3563): 184-6
- 285.
Soumillon M, Necsulea A, Weier M, et al. (2013) Cellular source and mechanisms of high transcriptome complexity in the mammalian testis. Cell Rep. 3(6): 2179-90
- 286.
Luo LF, Hou CC, Yang WX (2016) Small non-coding RNAs and their associated proteins in spermatogenesis. Gene. 578(2): 141-57
- 287.
Siomi MC, Sato K, Pezic D, Aravin AA (2011) PIWI-interacting small RNAs: the vanguard of genome defence. Nat Rev Mol Cell Biol. 12(4): 246-58
- 288.
Fu Q, Wang PJ (2014) Mammalian piRNAs: Biogenesis, function, and mysteries. Spermatogenesis. 4: e27889
- 289.
Wang L, Xu C (2015) Role of microRNAs in mammalian spermatogenesis and testicular germ cell tumors. Reproduction. 149(3): R127-37
- 290.
de Mateo S, Sassone-Corsi P (2014) Regulation of spermatogenesis by small non-coding RNAs: role of the germ granule. Semin Cell Dev Biol. 29: 84-92
- 291.
Yadav RP, Kotaja N (2014) Small RNAs in spermatogenesis. Mol Cell Endocrinol. 382(1): 498-508
- 292.
Hogg K, Western PS (2015) Refurbishing the germline epigenome: Out with the old, in with the new. Semin Cell Dev Biol. 45: 104-13
- 293.
Jurka J, Kapitonov VV, Kohany O, Jurka MV (2007) Repetitive sequences in complex genomes: structure and evolution. Annu Rev Genomics Hum Genet. 8: 241-59
- 294.
Rayan NA, Del Rosario RC, Prabhakar S (2016) Massive contribution of transposable elements to mammalian regulatory sequences. Semin Cell Dev Biol.
- 295.
Bourc'his D, Bestor TH (2004) Meiotic catastrophe and retrotransposon reactivation in male germ cells lacking Dnmt3L. Nature. 431(7004): 96-9
- 296.
Pastor WA, Stroud H, Nee K, et al. (2014) MORC1 represses transposable elements in the mouse male germline. Nat Commun. 5: 5795
- 297.
Kuramochi-Miyagawa S, Watanabe T, Gotoh K, et al. (2008) DNA methylation of retrotransposon genes is regulated by Piwi family members MILI and MIWI2 in murine fetal testes. Genes Dev. 22(7): 908-17
- 298.
Aravin AA, Sachidanandam R, Girard A, Fejes-Toth K, Hannon GJ (2007) Developmentally regulated piRNA clusters implicate MILI in transposon control. Science. 316(5825): 744-7
- 299.
Carmell MA, Girard A, van de Kant HJ, Bourc'his D, Bestor TH, de Rooij DG, Hannon GJ (2007) MIWI2 is essential for spermatogenesis and repression of transposons in the mouse male germline. Dev Cell. 12(4): 503-14
- 300.
Werner A, Piatek MJ, Mattick JS (2015) Transpositional shuffling and quality control in male germ cells to enhance evolution of complex organisms. Ann N Y Acad Sci. 1341: 156-63
- 301.
Ashe A, Sapetschnig A, Weick EM, et al. (2012) piRNAs can trigger a multigenerational epigenetic memory in the germline of C. elegans. Cell. 150(1): 88-99
- 302.
Schuster A, Skinner MK, Yan W (2016) Ancestral vinclozolin exposure alters the epigenetic transgenerational inheritance of sperm small noncoding RNAs. Environ Epigenet. 2(1)
- 303.
Meikar O, Vagin VV, Chalmel F, et al. (2014) An atlas of chromatoid body components. RNA. 20(4): 483-95
- 304.
Meikar O, Da Ros M, Korhonen H, Kotaja N (2011) Chromatoid body and small RNAs in male germ cells. Reproduction. 142(2): 195-209
- 305.
Yao C, Liu Y, Sun M, Niu M, Yuan Q, Hai Y, Guo Y, Chen Z, Hou J, He Z (2015) MicroRNAs and DNA methylation as epigenetic regulators of mitosis, meiosis and spermiogenesis. Reproduction. 150(1): R25-34
- 306.
Shirakawa T, Yaman-Deveci R, Tomizawa S, et al. (2013) An epigenetic switch is crucial for spermatogonia to exit the undifferentiated state toward a Kit-positive identity. Development. 140(17): 3565-76
- 307.
Papaioannou MD, Nef S (2010) microRNAs in the testis: building up male fertility. J Androl. 31(1): 26-33
- 308.
Winter J, Jung S, Keller S, Gregory RI, Diederichs S (2009) Many roads to maturity: microRNA biogenesis pathways and their regulation. Nat Cell Biol. 11(3): 228-34
- 309.
Wu Q, Song R, Ortogero N, Zheng H, Evanoff R, Small CL, Griswold MD, Namekawa SH, Royo H, Turner JM, Yan W (2012) The RNase III Enzyme DROSHA Is Essential for MicroRNA Production and Spermatogenesis. J Biol Chem. 287(30): 25173-90
- 310.
Ro S, Park C, Sanders KM, McCarrey JR, Yan W (2007) Cloning and expression profiling of testis-expressed microRNAs. Dev Biol. 311(2): 592-602
- 311.
Han T, Manoharan AP, Harkins TT, Bouffard P, Fitzpatrick C, Chu DS, Thierry-Mieg D, Thierry-Mieg J, Kim JK (2009) 26G endo-siRNAs regulate spermatogenic and zygotic gene expression in Caenorhabditis elegans. Proc Natl Acad Sci U S A. 106(44): 18674-9
- 312.
Pavelec DM, Lachowiec J, Duchaine TF, Smith HE, Kennedy S (2009) Requirement for the ERI/DICER complex in endogenous RNA interference and sperm development in Caenorhabditis elegans. Genetics. 183(4): 1283-95
- 313.
Song R, Hennig GW, Wu Q, Jose C, Zheng H, Yan W (2011) Male germ cells express abundant endogenous siRNAs. Proc Natl Acad Sci U S A. 108(32): 13159-64
- 314.
Ortogero N, Schuster AS, Oliver DK, et al. (2014) A novel class of somatic small RNAs similar to germ cell pachytene PIWI-interacting small RNAs. J Biol Chem. 289(47): 32824-34
- 315.
Lim SL, Qu ZP, Kortschak RD, et al. (2015) HENMT1 and piRNA Stability Are Required for Adult Male Germ Cell Transposon Repression and to Define the Spermatogenic Program in the Mouse. PLoS Genet. 11(10): e1005620
- 316.
Reuter M, Berninger P, Chuma S, Shah H, Hosokawa M, Funaya C, Antony C, Sachidanandam R, Pillai RS (2011) Miwi catalysis is required for piRNA amplification-independent LINE1 transposon silencing. Nature. 480(7376): 264-7
- 317.
Di Giacomo M, Comazzetto S, Saini H, De Fazio S, Carrieri C, Morgan M, Vasiliauskaite L, Benes V, Enright AJ, O'Carroll D (2013) Multiple epigenetic mechanisms and the piRNA pathway enforce LINE1 silencing during adult spermatogenesis. Mol Cell. 50(4): 601-8
- 318.
Pantano L, Jodar M, Bak M, Ballesca JL, Tommerup N, Oliva R, Vavouri T (2015) The small RNA content of human sperm reveals pseudogene-derived piRNAs complementary to protein-coding genes. RNA. 21(6): 1085-95
- 319.
Goh WS, Falciatori I, Tam OH, Burgess R, Meikar O, Kotaja N, Hammell M, Hannon GJ (2015) piRNA-directed cleavage of meiotic transcripts regulates spermatogenesis. Genes Dev. 29(10): 1032-44
- 320.
Watanabe T, Cheng EC, Zhong M, Lin H (2015) Retrotransposons and pseudogenes regulate mRNAs and lncRNAs via the piRNA pathway in the germline. Genome Res. 25(3): 368-80
- 321.
Zhang P, Kang JY, Gou LT, et al. (2015) MIWI and piRNA-mediated cleavage of messenger RNAs in mouse testes. Cell Res. 25(2): 193-207
- 322.
Luk AC, Chan WY, Rennert OM, Lee TL (2014) Long noncoding RNAs in spermatogenesis: insights from recent high-throughput transcriptome studies. Reproduction. 147(5): R131-41
- 323.
Zhang C, Gao L, Xu EY (2016) LncRNA, a new component of expanding RNA-protein regulatory network important for animal sperm development. Semin Cell Dev Biol.
- 324.
Lin X, Han M, Cheng L, Chen J, Zhang Z, Shen T, Wang M, Wen B, Ni T, Han C (2016) Expression dynamics, relationships, and transcriptional regulations of diverse transcripts in mouse spermatogenic cells. RNA Biol. 13(10): 1011-1024
- 325.
Liang M, Li W, Tian H, Hu T, Wang L, Lin Y, Li Y, Huang H, Sun F (2014) Sequential expression of long noncoding RNA as mRNA gene expression in specific stages of mouse spermatogenesis. Sci Rep. 4: 5966
- 326.
Wen K, Yang L, Xiong T, et al. (2016) Critical roles of long noncoding RNAs in Drosophila spermatogenesis. Genome Res. 26(9): 1233-44
- 327.
Li L, Wang M, Wu X, Geng L, Xue Y, Wei X, Jia Y (2016) A long non-coding RNA interacts with Gfra1 and maintains survival of mouse spermatogonial stem cells. Cell Death Dis. 7: e2140
- 328.
Stewart KR, Veselovska L, Kelsey G (2016) Establishment and functions of DNA methylation in the germline. Epigenomics. 8(10): 1399-1413
- 329.
Ly L, Chan D, Trasler JM (2015) Developmental windows of susceptibility for epigenetic inheritance through the male germline. Semin Cell Dev Biol. 43: 96-105
- 330.
Soubry A, Hoyo C, Jirtle RL, Murphy SK (2014) A paternal environmental legacy: evidence for epigenetic inheritance through the male germ line. Bioessays. 36(4): 359-71
- 331.
Wei Y, Schatten H, Sun QY (2015) Environmental epigenetic inheritance through gametes and implications for human reproduction. Hum Reprod Update. 21(2): 194-208
- 332.
Skinner MK (2014) Endocrine disruptor induction of epigenetic transgenerational inheritance of disease. Mol Cell Endocrinol. 398(1-2): 4-12
- 333.
Skinner MK (2016) Endocrine disruptors in 2015: Epigenetic transgenerational inheritance. Nat Rev Endocrinol. 12(2): 68-70
- 334.
Anway MD, Rekow SS, Skinner MK (2008) Transgenerational epigenetic programming of the embryonic testis transcriptome. Genomics. 91(1): 30-40
- 335.
Anway MD, Cupp AS, Uzumcu M, Skinner MK (2005) Epigenetic transgenerational actions of endocrine disruptors and male fertility. Science. 308(5727): 1466-9
- 336.
Ng SF, Lin RC, Laybutt DR, Barres R, Owens JA, Morris MJ (2010) Chronic high-fat diet in fathers programs beta-cell dysfunction in female rat offspring. Nature. 467(7318): 963-6
- 337.
Fullston T, Ohlsson Teague EM, Palmer NO, DeBlasio MJ, Mitchell M, Corbett M, Print CG, Owens JA, Lane M (2013) Paternal obesity initiates metabolic disturbances in two generations of mice with incomplete penetrance to the F2 generation and alters the transcriptional profile of testis and sperm microRNA content. FASEB J. 27(10): 4226-43
- 338.
Lambrot R, Xu C, Saint-Phar S, Chountalos G, Cohen T, Paquet M, Suderman M, Hallett M, Kimmins S (2013) Low paternal dietary folate alters the mouse sperm epigenome and is associated with negative pregnancy outcomes. Nat Commun. 4: 2889
- 339.
Cui X, Jing X, Wu X, Yan M, Li Q, Shen Y, Wang Z (2016) DNA methylation in spermatogenesis and male infertility. Exp Ther Med. 12(4): 1973-1979
- 340.
Rando OJ (2012) Daddy issues: paternal effects on phenotype. Cell. 151(4): 702-8
- 341.
Daxinger L, Whitelaw E (2012) Understanding transgenerational epigenetic inheritance via the gametes in mammals. Nat Rev Genet. 13(3): 153-62
- 342.
Conine CC, Moresco JJ, Gu W, Shirayama M, Conte D, Jr., Yates JR, 3rd, Mello CC (2013) Argonautes promote male fertility and provide a paternal memory of germline gene expression in C. elegans. Cell. 155(7): 1532-44
- 343.
Yuan S, Schuster A, Tang C, Yu T, Ortogero N, Bao J, Zheng H, Yan W (2016) Sperm-borne miRNAs and endo-siRNAs are important for fertilization and preimplantation embryonic development. Development. 143(4): 635-47
- 344.
Gapp K, Jawaid A, Sarkies P, Bohacek J, Pelczar P, Prados J, Farinelli L, Miska E, Mansuy IM (2014) Implication of sperm RNAs in transgenerational inheritance of the effects of early trauma in mice. Nat Neurosci. 17(5): 667-9
- 345.
Sharma U, Conine CC, Shea JM, et al. (2016) Biogenesis and function of tRNA fragments during sperm maturation and fertilization in mammals. Science. 351(6271): 391-6
- 346.
Hayes F, Dwyer A, Pitteloud N. (2013) Hypogonadotropic hypogondism (HH) and gonadotropin therapy, in www
.ENDOTEXT.org, Endocrinology of Male Reproduction, Section Editor McLachlan R.I. MDTEXTCOM Inc.: South Dartmouth, MA - 347.
Plant TM (2008) Hypothalamic control of the pituitary-gonadal axis in higher primates: key advances over the last two decades. J Neuroendocrinol. 20(6): 719-26
- 348.
Pinilla L, Aguilar E, Dieguez C, Millar RP, Tena-Sempere M (2012) Kisspeptins and reproduction: physiological roles and regulatory mechanisms. Physiol Rev. 92(3): 1235-316
- 349.
Mittelman-Smith MA, Williams H, Krajewski-Hall SJ, Lai J, Ciofi P, McMullen NT, Rance NE (2012) Arcuate kisspeptin/neurokinin B/dynorphin (KNDy) neurons mediate the estrogen suppression of gonadotropin secretion and body weight. Endocrinology. 153(6): 2800-12
- 350.
Tsutsui K, Ubuka T, Bentley GE, Kriegsfeld LJ (2012) Gonadotropin-inhibitory hormone (GnIH): discovery, progress and prospect. Gen Comp Endocrinol. 177(3): 305-14
- 351.
Clarke IJ (2011) Control of GnRH secretion: one step back. Front Neuroendocrinol. 32(3): 367-75
- 352.
Phillips DJ, de Kretser DM (1998) Follistatin: a multifunctional regulatory protein. Front Neuroendocrinol. 19(4): 287-322
- 353.
Jin JM, Yang WX (2014) Molecular regulation of hypothalamus-pituitary-gonads axis in males. Gene. 551(1): 15-25
- 354.
Hashimoto O, Nakamura T, Shoji H, Shimasaki S, Hayashi Y, Sugino H (1997) A novel role of follistatin, an activin-binding protein, in the inhibition of activin action in rat pituitary cells. Endocytotic degradation of activin and its acceleration by follistatin associated with cell-surface heparan sulfate. J Biol Chem. 272(21): 13835-42
- 355.
Sherins RJ, Loriaux DL (1973) Studies of the role of sex steroids in the feedback control of FSH concentrations in men. J Clin Endocrinol Metab. 36(5): 886-93
- 356.
Naftolin F, Ryan KJ, Petro Z (1971) Aromatization of androstenedione by the diencephalon. J Clin Endocrinol Metab. 33(2): 368-70
- 357.
Santen RJ (1975) Is aromatization of testosterone to estradiol required for inhibition of luteinizing hormone secretion in men? J Clin Invest. 56(6): 1555-63
- 358.
Santen RJ, Bardin CW (1973) Episodic luteinizing hormone secretion in man. Pulse analysis, clinical interpretation, physiologic mechanisms. J Clin Invest. 52(10): 2617-28
- 359.
Hayes FJ, Seminara SB, Decruz S, Boepple PA, Crowley WF, Jr. (2000) Aromatase inhibition in the human male reveals a hypothalamic site of estrogen feedback. J Clin Endocrinol Metab. 85(9): 3027-35
- 360.
Tilbrook AJ, de Kretser DM, Cummins JT, Clarke IJ (1991) The negative feedback effects of testicular steroids are predominantly at the hypothalamus in the ram. Endocrinology. 129(6): 3080-92
- 361.
Decker MH, Loriaux DL, Cutler GB, Jr. (1981) A seminiferous tubular factor is not obligatory for regulation of plasma follicle-stimulating hormone in the rat. Endocrinology. 108(3): 1035-9
- 362.
McCullagh DR (1932) Dual Endocrine Activity of the Testes. Science. 76(1957): 19-20
- 363.
de Kretser DM, Robertson DM (1989) The isolation and physiology of inhibin and related proteins. Biol Reprod. 40(1): 33-47
- 364.
Forage RG, Ring JM, Brown RW, McInerney BV, Cobon GS, Gregson RP, Robertson DM, Morgan FJ, Hearn MT, Findlay JK, et al. (1986) Cloning and sequence analysis of cDNA species coding for the two subunits of inhibin from bovine follicular fluid. Proc Natl Acad Sci U S A. 83(10): 3091-5
- 365.
Ling N, Ying SY, Ueno N, Esch F, Denoroy L, Guillemin R (1985) Isolation and partial characterization of a Mr 32,000 protein with inhibin activity from porcine follicular fluid. Proc Natl Acad Sci U S A. 82(21): 7217-21
- 366.
Mason AJ, Hayflick JS, Ling N, Esch F, Ueno N, Ying SY, Guillemin R, Niall H, Seeburg PH (1985) Complementary DNA sequences of ovarian follicular fluid inhibin show precursor structure and homology with transforming growth factor-beta. Nature. 318(6047): 659-63
- 367.
Robertson DM, Foulds LM, Leversha L, Morgan FJ, Hearn MT, Burger HG, Wettenhall RE, de Kretser DM (1985) Isolation of inhibin from bovine follicular fluid. Biochem Biophys Res Commun. 126(1): 220-6
- 368.
Ling N, Ying SY, Ueno N, Shimasaki S, Esch F, Hotta M, Guillemin R (1986) Pituitary FSH is released by a heterodimer of the beta-subunits from the two forms of inhibin. Nature. 321(6072): 779-82
- 369.
Vale W, Rivier J, Vaughan J, McClintock R, Corrigan A, Woo W, Karr D, Spiess J (1986) Purification and characterization of an FSH releasing protein from porcine ovarian follicular fluid. Nature. 321(6072): 776-9
- 370.
Robertson DM, Klein R, de Vos FL, McLachlan RI, Wettenhall RE, Hearn MT, Burger HG, de Kretser DM (1987) The isolation of polypeptides with FSH suppressing activity from bovine follicular fluid which are structurally different to inhibin. Biochem Biophys Res Commun. 149(2): 744-9
- 371.
Shimasaki S, Koga M, Esch F, Mercado M, Cooksey K, Koba A, Ling N (1988) Porcine follistatin gene structure supports two forms of mature follistatin produced by alternative splicing. Biochem Biophys Res Commun. 152(2): 717-23
- 372.
Ueno N, Ling N, Ying SY, Esch F, Shimasaki S, Guillemin R (1987) Isolation and partial characterization of follistatin: a single-chain Mr 35,000 monomeric protein that inhibits the release of follicle-stimulating hormone. Proc Natl Acad Sci U S A. 84(23): 8282-6
- 373.
Nakamura T, Takio K, Eto Y, Shibai H, Titani K, Sugino H (1990) Activin-binding protein from rat ovary is follistatin. Science. 247(4944): 836-8
- 374.
Rea MA, Marshall GR, Weinbauer GF, Nieschlag E (1986) Testosterone maintains pituitary and serum FSH and spermatogenesis in gonadotrophin-releasing hormone antagonist-suppressed rats. J Endocrinol. 108(1): 101-7
- 375.
Sun YT, Irby DC, Robertson DM, de Kretser DM (1989) The effects of exogenously administered testosterone on spermatogenesis in intact and hypophysectomized rats. Endocrinology. 125(2): 1000-10
- 376.
Jackson CM, Morris ID (1977) Gonadotrophin levels in male rats following impairment of Leydig cell function by ethylene dimethanesulphonate. Andrologia. 9(1): 29-35
- 377.
De Kretser DM, O'Leary PC, Irby DC, Risbridger GP (1989) Inhibin secretion is influenced by Leydig cells: evidence from studies using the cytotoxin ethane dimethane sulphonate (EDS). Int J Androl. 12(4): 273-80
- 378.
O'Leary P, Jackson AE, Averill S, de Kretser DM (1986) The effects of ethane dimethane sulphonate (EDS) on bilaterally cryptorchid rat testes. Mol Cell Endocrinol. 45(2-3): 183-90
- 379.
Le Gac F, de Kretser DM (1982) Inhibin production by Sertoli cell cultures. Mol Cell Endocrinol. 28(3): 487-98
- 380.
Steinberger A, Steinberger E (1976) Secretion of an FSH-inhibiting factor by cultured Sertoli cells. Endocrinology. 99(3): 918-21
- 381.
Klaij IA, Timmerman MA, Blok LJ, Grootegoed JA, de Jong FH (1992) Regulation of inhibin beta B-subunit mRNA expression in rat Sertoli cells: consequences for the production of bioactive and immunoreactive inhibin. Mol Cell Endocrinol. 85(3): 237-46
- 382.
Sharpe RM, Turner KJ, McKinnell C, Groome NP, Atanassova N, Millar MR, Buchanan DL, Cooke PS (1999) Inhibin B levels in plasma of the male rat from birth to adulthood: effect of experimental manipulation of Sertoli cell number. J Androl. 20(1): 94-101
- 383.
Anawalt BD, Bebb RA, Matsumoto AM, Groome NP, Illingworth PJ, McNeilly AS, Bremner WJ (1996) Serum inhibin B levels reflect Sertoli cell function in normal men and men with testicular dysfunction. J Clin Endocrinol Metab. 81(9): 3341-5
- 384.
Anderson RA, Wallace EM, Groome NP, Bellis AJ, Wu FC (1997) Physiological relationships between inhibin B, follicle stimulating hormone secretion and spermatogenesis in normal men and response to gonadotrophin suppression by exogenous testosterone. Hum Reprod. 12(4): 746-51
- 385.
Krummen LA, Toppari J, Kim WH, Morelos BS, Ahmad N, Swerdloff RS, Ling N, Shimasaki S, Esch F, Bhasin S (1989) Regulation of testicular inhibin subunit messenger ribonucleic acid levels in vivo: effects of hypophysectomy and selective follicle-stimulating hormone replacement. Endocrinology. 125(3): 1630-7
- 386.
Wallace EM, Groome NP, Riley SC, Parker AC, Wu FC (1997) Effects of chemotherapy-induced testicular damage on inhibin, gonadotropin, and testosterone secretion: a prospective longitudinal study. J Clin Endocrinol Metab. 82(9): 3111-5
- 387.
Risbridger GP, Clements J, Robertson DM, Drummond AE, Muir J, Burger HG, de Kretser DM (1989) Immuno- and bioactive inhibin and inhibin alpha-subunit expression in rat Leydig cell cultures. Mol Cell Endocrinol. 66(1): 119-22
- 388.
McLachlan RI, Matsumoto AM, Burger HG, de Kretser DM, Bremner WJ (1988) Relative roles of follicle-stimulating hormone and luteinizing hormone in the control of inhibin secretion in normal men. J Clin Invest. 82(3): 880-4
- 389.
Tena-Sempere M, Kero J, Rannikko A, Yan W, Huhtaniemi I (1999) The pattern of inhibin/activin alpha- and betaB-subunit messenger ribonucleic acid expression in rat testis after selective Leydig cell destruction by ethylene dimethane sulfonate. Endocrinology. 140(12): 5761-70
- 390.
Matthiesson KL, Robertson DM, Burger HG, McLachlan RI (2003) Response of serum inhibin B and pro-alphaC levels to gonadotrophic stimulation in normal men before and after steroidal contraceptive treatment. Hum Reprod. 18(4): 734-43
- 391.
de Winter JP, Vanderstichele HM, Timmerman MA, Blok LJ, Themmen AP, de Jong FH (1993) Activin is produced by rat Sertoli cells in vitro and can act as an autocrine regulator of Sertoli cell function. Endocrinology. 132(3): 975-82
- 392.
Lee W, Mason AJ, Schwall R, Szonyi E, Mather JP (1989) Secretion of activin by interstitial cells in the testis. Science. 243(4889): 396-8
- 393.
McFarlane JR, Foulds LM, Pisciotta A, Robertson DM, de Kretser DM (1996) Measurement of activin in biological fluids by radioimmunoassay, utilizing dissociating agents to remove the interference of follistatin. Eur J Endocrinol. 134(4): 481-9
- 394.
Mather JP, Attie KM, Woodruff TK, Rice GC, Phillips DM (1990) Activin stimulates spermatogonial proliferation in germ-Sertoli cell cocultures from immature rat testis. Endocrinology. 127(6): 3206-14
- 395.
Archambeault DR, Yao HH (2010) Activin A, a product of fetal Leydig cells, is a unique paracrine regulator of Sertoli cell proliferation and fetal testis cord expansion. Proc Natl Acad Sci U S A. 107(23): 10526-31
- 396.
Buzzard JJ, Farnworth PG, De Kretser DM, O'Connor AE, Wreford NG, Morrison JR (2003) Proliferative phase sertoli cells display a developmentally regulated response to activin in vitro. Endocrinology. 144(2): 474-83
- 397.
Mendis SH, Meachem SJ, Sarraj MA, Loveland KL (2011) Activin A balances Sertoli and germ cell proliferation in the fetal mouse testis. Biol Reprod. 84(2): 379-91
- 398.
de Winter JP, Themmen AP, Hoogerbrugge JW, Klaij IA, Grootegoed JA, de Jong FH (1992) Activin receptor mRNA expression in rat testicular cell types. Mol Cell Endocrinol. 83(1): R1-8
- 399.
Meinhardt A, O'Bryan MK, McFarlane JR, Loveland KL, Mallidis C, Foulds LM, Phillips DJ, de Kretser DM (1998) Localization of follistatin in the rat testis. J Reprod Fertil. 112(2): 233-41
- 400.
Michel U, Albiston A, Findlay JK (1990) Rat follistatin: gonadal and extragonadal expression and evidence for alternative splicing. Biochem Biophys Res Commun. 173(1): 401-7
- 401.
Tilbrook AJ, de Kretser DM, Dunshea FR, Klein R, Robertson DM, Clarke IJ, Maddocks S (1996) The testis is not the major source of circulating follistatin in the ram. J Endocrinol. 149(1): 55-63
- 402.
Phillips DJ, Hedger MP, McFarlane JR, Klein R, Clarke IJ, Tilbrook AJ, Nash AD, de Kretser DM (1996) Follistatin concentrations in male sheep increase following sham castration/castration or injection of interleukin-1 beta. J Endocrinol. 151(1): 119-24
- 403.
Dubey AK, Zeleznik AJ, Plant TM (1987) In the rhesus monkey (Macaca mulatta), the negative feedback regulation of follicle-stimulating hormone secretion by an action of testicular hormone directly at the level of the anterior pituitary gland cannot be accounted for by either testosterone or estradiol. Endocrinology. 121(6): 2229-37
- 404.
Ramaswamy S, Pohl CR, McNeilly AS, Winters SJ, Plant TM (1998) The time course of follicle-stimulating hormone suppression by recombinant human inhibin A in the adult male rhesus monkey (Macaca mulatta). Endocrinology. 139(8): 3409-15
- 405.
Robertson DM, Prisk M, McMaster JW, Irby DC, Findlay JK, de Kretser DM (1991) Serum FSH-suppressing activity of human recombinant inhibin A in male and female rats. J Reprod Fertil. 91(1): 321-8
- 406.
Tilbrook AJ, de Kretser DM, Clarke IJ (1993) Human recombinant inhibin A and testosterone act directly at the pituitary to suppress plasma concentrations of FSH in castrated rams. J Endocrinol. 138(2): 181-9
- 407.
Tilbrook AJ, De Kretser DM, Clarke IJ (1993) Human recombinant inhibin A suppresses plasma follicle-stimulating hormone to intact levels but has no effect on luteinizing hormone in castrated rams. Biol Reprod. 49(4): 779-88
- 408.
Roberts V, Meunier H, Vaughan J, Rivier J, Rivier C, Vale W, Sawchenko P (1989) Production and regulation of inhibin subunits in pituitary gonadotropes. Endocrinology. 124(1): 552-4
- 409.
Corrigan AZ, Bilezikjian LM, Carroll RS, Bald LN, Schmelzer CH, Fendly BM, Mason AJ, Chin WW, Schwall RH, Vale W (1991) Evidence for an autocrine role of activin B within rat anterior pituitary cultures. Endocrinology. 128(3): 1682-4
- 410.
Gospodarowicz D, Lau K (1989) Pituitary follicular cells secrete both vascular endothelial growth factor and follistatin. Biochem Biophys Res Commun. 165(1): 292-8
- 411.
Kogawa K, Nakamura T, Sugino K, Takio K, Titani K, Sugino H (1991) Activin-binding protein is present in pituitary. Endocrinology. 128(3): 1434-40
- 412.
Bilezikjian LM, Corrigan AZ, Blount AL, Vale WW (1996) Pituitary follistatin and inhibin subunit messenger ribonucleic acid levels are differentially regulated by local and hormonal factors. Endocrinology. 137(10): 4277-84
- 413.
Bilezikjian LM, Vaughan JM, Vale WW (1993) Characterization and the regulation of inhibin/activin subunit proteins of cultured rat anterior pituitary cells. Endocrinology. 133(6): 2545-53
- 414.
Gonzales GF, Risbridger GP, de Krester DM (1989) In vivo and in vitro production of inhibin by cryptorchid testes from adult rats. Endocrinology. 124(4): 1661-8
- 415.
Weinbauer GF, Bartlett JM, Fingscheidt U, Tsonis CG, de Kretser DM, Nieschlag E (1989) Evidence for a major role of inhibin in the feedback control of FSH in the male rat. J Reprod Fertil. 85(2): 355-62
- 416.
Jensen TK, Andersson AM, Hjollund NH, et al. (1997) Inhibin B as a serum marker of spermatogenesis: correlation to differences in sperm concentration and follicle-stimulating hormone levels. A study of 349 Danish men. J Clin Endocrinol Metab. 82(12): 4059-63
- 417.
Matzuk MM, Kumar TR, Bradley A (1995) Different phenotypes for mice deficient in either activins or activin receptor type II. Nature. 374(6520): 356-60
- 418.
Singh J, O'Neill C, Handelsman DJ (1995) Induction of spermatogenesis by androgens in gonadotropin-deficient (hpg) mice. Endocrinology. 136(12): 5311-21
- 419.
O’Donnell L, McLachlan RI (2012) The role of testosterone in spermatogenesis, in Testosterone: action, deficiency, substitution, Nieschlag, E., Behre, H.M., and Nieschlag, S., Editors. Cambridge University Press: New York, USA. p. 123-153
- 420.
Huang HF, Marshall GR, Rosenberg R, Nieschlag E (1987) Restoration of spermatogenesis by high levels of testosterone in hypophysectomized rats after long-term regression. Acta Endocrinol (Copenh). 116(4): 433-44
- 421.
Grino PB, Griffin JE, Wilson JD (1990) Testosterone at high concentrations interacts with the human androgen receptor similarly to dihydrotestosterone. Endocrinology. 126(2): 1165-72
- 422.
Handelsman DJ, Spaliviero JA, Simpson JM, Allan CM, Singh J (1999) Spermatogenesis without gonadotropins: maintenance has a lower testosterone threshold than initiation. Endocrinology. 140(9): 3938-46
- 423.
Zhang FP, Pakarainen T, Poutanen M, Toppari J, Huhtaniemi I (2003) The low gonadotropin-independent constitutive production of testicular testosterone is sufficient to maintain spermatogenesis. Proc Natl Acad Sci U S A. 100(23): 13692-7
- 424.
Bremner WJ, Millar MR, Sharpe RM, Saunders PT (1994) Immunohistochemical localization of androgen receptors in the rat testis: evidence for stage-dependent expression and regulation by androgens. Endocrinology. 135(3): 1227-34
- 425.
Van Roijen JH, Van Assen S, Van Der Kwast TH, De Rooij DG, Boersma WJ, Vreeburg JT, Weber RF (1995) Androgen receptor immunoexpression in the testes of subfertile men. J Androl. 16(6): 510-6
- 426.
Welsh M, Sharpe RM, Moffat L, Atanassova N, Saunders PT, Kilter S, Bergh A, Smith LB (2010) Androgen action via testicular arteriole smooth muscle cells is important for Leydig cell function, vasomotion and testicular fluid dynamics. PLoS One. 5(10): e13632
- 427.
Johnston DS, Russell LD, Friel PJ, Griswold MD (2001) Murine germ cells do not require functional androgen receptors to complete spermatogenesis following spermatogonial stem cell transplantation. Endocrinology. 142(6): 2405-8
- 428.
Tsai MY, Yeh SD, Wang RS, Yeh S, Zhang C, Lin HY, Tzeng CR, Chang C (2006) Differential effects of spermatogenesis and fertility in mice lacking androgen receptor in individual testis cells. Proc Natl Acad Sci U S A. 103(50): 18975-80
- 429.
Lim P, Robson M, Spaliviero J, McTavish KJ, Jimenez M, Zajac JD, Handelsman DJ, Allan CM (2009) Sertoli cell androgen receptor DNA binding domain is essential for the completion of spermatogenesis. Endocrinology. 150(10): 4755-65
- 430.
O'Donnell L, McLachlan RI, Wreford NG, de Kretser DM, Robertson DM (1996) Testosterone withdrawal promotes stage-specific detachment of round spermatids from the rat seminiferous epithelium. Biol Reprod. 55(4): 895-901
- 431.
O'Donnell L, Pratis K, Stanton PG, Robertson DM, McLachlan RI (1999) Testosterone-dependent restoration of spermatogenesis in adult rats is impaired by a 5alpha-reductase inhibitor. J Androl. 20(1): 109-17
- 432.
Meng J, Holdcraft RW, Shima JE, Griswold MD, Braun RE (2005) Androgens regulate the permeability of the blood-testis barrier. Proc Natl Acad Sci U S A. 102(46): 16696-700
- 433.
McCabe MJ, Allan CM, Foo CF, Nicholls PK, McTavish KJ, Stanton PG (2012) Androgen Initiates Sertoli Cell Tight Junction Formation in the Hypogonadal (hpg) Mouse. Biol Reprod. 87(2): 38
- 434.
Willems A, Batlouni SR, Esnal A, Swinnen JV, Saunders PT, Sharpe RM, Franca LR, De Gendt K, Verhoeven G (2010) Selective ablation of the androgen receptor in mouse Sertoli cells affects Sertoli cell maturation, barrier formation and cytoskeletal development. PLoS One. 5(11): e14168
- 435.
Zhengwei Y, Wreford NG, Royce P, de Kretser DM, McLachlan RI (1998) Stereological evaluation of human spermatogenesis after suppression by testosterone treatment: heterogeneous pattern of spermatogenic impairment. J Clin Endocrinol Metab. 83(4): 1284-91
- 436.
Weinbauer GF, Schlatt S, Walter V, Nieschlag E (2001) Testosterone-induced inhibition of spermatogenesis is more closely related to suppression of FSH than to testicular androgen levels in the cynomolgus monkey model (Macaca fascicularis). J Endocrinol. 168(1): 25-38
- 437.
Narula A, Gu YQ, O'Donnell L, Stanton PG, Robertson DM, McLachlan RI, Bremner WJ (2002) Variability in sperm suppression during testosterone administration to adult monkeys is related to follicle stimulating hormone suppression and not to intratesticular androgens. J Clin Endocrinol Metab. 87(7): 3399-406
- 438.
Allan CM, Handelsman DJ (2005) Transgenic models for exploring gonadotropin biology in the male. Endocrine. 26(3): 235-39
- 439.
Heckert L, Griswold MD (1993) Expression of the FSH receptor in the testis. Recent Prog Horm Res. 48: 61-77
- 440.
Rannikko A, Penttila TL, Zhang FP, Toppari J, Parvinen M, Huhtaniemi I (1996) Stage-specific expression of the FSH receptor gene in the prepubertal and adult rat seminiferous epithelium. J Endocrinol. 151(1): 29-35
- 441.
Allan CM, Garcia A, Spaliviero J, Zhang FP, Jimenez M, Huhtaniemi I, Handelsman DJ (2004) Complete Sertoli cell proliferation induced by follicle-stimulating hormone (FSH) independently of luteinizing hormone activity: evidence from genetic models of isolated FSH action. Endocrinology. 145(4): 1587-93
- 442.
O'Donnell L, Narula A, Balourdos G, Gu YQ, Wreford NG, Robertson DM, Bremner WJ, McLachlan RI (2001) Impairment of spermatogonial development and spermiation after testosterone-induced gonadotropin suppression in adult monkeys (Macaca fascicularis). J Clin Endocrinol Metab. 86(4): 1814-22
- 443.
McLachlan RI, O'Donnell L, Meachem SJ, Stanton PG, de K, Pratis K, Robertson DM (2002) Hormonal regulation of spermatogenesis in primates and man: insights for development of the male hormonal contraceptive. J Androl. 23(2): 149-62
- 444.
Meachem SJ, Wreford NG, Stanton PG, Robertson DM, McLachlan RI (1998) Follicle-stimulating hormone is required for the initial phase of spermatogenic restoration in adult rats following gonadotropin suppression. J Androl. 19(6): 725-35
- 445.
Matsumoto AM, Karpas AE, Paulsen CA, Bremner WJ (1983) Reinitiation of sperm production in gonadotropin-suppressed normal men by administration of follicle-stimulating hormone. J Clin Invest. 72(3): 1005-15
- 446.
Matsumoto AM, Paulsen CA, Bremner WJ (1984) Stimulation of sperm production by human luteinizing hormone in gonadotropin-suppressed normal men. J Clin Endocrinol Metab. 59(5): 882-7
- 447.
Bremner WJ, Matsumoto AM, Sussman AM, Paulsen CA (1981) Follicle-stimulating hormone and human spermatogenesis. J Clin Invest. 68(4): 1044-52
- 448.
Kumar R (2013) Medical management of non-obstructive azoospermia. Clinics (Sao Paulo). 68 Suppl 1: 75-9
- 449.
McLachlan RI (2013) Approach to the patient with oligozoospermia. J Clin Endocrinol Metab. 98(3): 873-80
- 450.
Handelsman DJ. (2015) Male contraception, in www
.ENDOTEXT.org, Endocrinology of Male Reproduction, Section Editor McLachlan R.I. MDTEXTCOM Inc.: South Dartmouth, MA
- ABSTRACT
- CLINICAL SUMMARY
- GENERAL ANATOMY OF THE MALE REPRODUCTIVE SYSTEM
- AN OVERVIEW OF SPERMATOGENESIS
- THE ROLE OF SERTOLI CELLS IN SPERMATOGENESIS
- LEYDIG CELLS AND STEROIDOGENESIS
- ROLE OF PERITUBULAR MYOID CELLS
- THE REGULATION OF SPERMATOGENESIS
- THE HYPOTHALAMIC-PITUITARY-TESTIS AXIS
- SUMMARY OF THE ENDOCRINE REGULATION OF SPERM PRODUCTION: CLINICAL CONSIDERATIONS
- REFERENCES
- Review Endocrinology of male infertility.[Br Med Bull. 1979]Review Endocrinology of male infertility.de Kretser DM. Br Med Bull. 1979 May; 35(2):187-92.
- The testicular transcriptome associated with spermatogonia differentiation initiated by gonadotrophin stimulation in the juvenile rhesus monkey (Macaca mulatta).[Hum Reprod. 2017]The testicular transcriptome associated with spermatogonia differentiation initiated by gonadotrophin stimulation in the juvenile rhesus monkey (Macaca mulatta).Ramaswamy S, Walker WH, Aliberti P, Sethi R, Marshall GR, Smith A, Nourashrafeddin S, Belgorosky A, Chandran UR, Hedger MP, et al. Hum Reprod. 2017 Oct 1; 32(10):2088-2100.
- Age-related presence of spermatogonia in patients with Klinefelter syndrome: a systematic review and meta-analysis.[Hum Reprod Update. 2020]Age-related presence of spermatogonia in patients with Klinefelter syndrome: a systematic review and meta-analysis.Deebel NA, Galdon G, Zarandi NP, Stogner-Underwood K, Howards S, Lovato J, Kogan S, Atala A, Lue Y, Sadri-Ardekani H. Hum Reprod Update. 2020 Jan 1; 26(1):58-72.
- Ethanolic extract of Moringa oleifera leaves alleviate cyclophosphamide-induced testicular toxicity by improving endocrine function and modulating cell specific gene expression in mouse testis.[J Ethnopharmacol. 2020]Ethanolic extract of Moringa oleifera leaves alleviate cyclophosphamide-induced testicular toxicity by improving endocrine function and modulating cell specific gene expression in mouse testis.Nayak G, Rao A, Mullick P, Mutalik S, Kalthur SG, Adiga SK, Kalthur G. J Ethnopharmacol. 2020 Sep 15; 259:112922. Epub 2020 May 15.
- Review Regulation of testicular function in the stallion: an intricate network of endocrine, paracrine and autocrine systems.[Anim Reprod Sci. 2008]Review Regulation of testicular function in the stallion: an intricate network of endocrine, paracrine and autocrine systems.Roser JF. Anim Reprod Sci. 2008 Sep; 107(3-4):179-96. Epub 2008 May 9.
- Endocrinology of the Male Reproductive System and Spermatogenesis - EndotextEndocrinology of the Male Reproductive System and Spermatogenesis - Endotext
Your browsing activity is empty.
Activity recording is turned off.
See more...