INTRODUCTION
A major aspect of reproductive capacity in women is its cyclical activity, a feature strikingly reflected in the growth and development of dominant follicles. Normally, the human ovaries produce a single dominant follicle that results in a single ovulation each menstrual cycle. The dominant follicle is responsible for the production of estradiol during the follicular phase of the cycle. After ovulation, the dominant follicle transforms into the corpus luteum, which secretes large amounts of progesterone during the luteal phase of the menstrual cycle. The estradiol and progesterone act on the uterus to prepare it for implantation of the human embryo. Therefore, to understand the menstrual cycle and female fertility, it is necessary to understand the life cycle of the dominant follicle and how it is controlled. Here, the structure/function relationships that underlie folliculogenesis, ovulation, and luteogenesis will be discussed. Attention will be focused on the human.
FOLLICULOGENESIS
Folliculogenesis begins with the recruitment of a primordial follicle into the pool of growing follicles and ends with either ovulation or death by atresia. In women, folliculogenesis is a very long process, requiring almost one year for a primordial follicle to grow and develop to the ovulatory stage. Folliculogenesis can be divided into two phases. The first phase, termed the preantral or gonadotropin-independent phase, is characterized by the growth and differentiation of the oocyte. The second, termed the antral or gonadotropin-dependent phase, is characterized by the tremendous increase of the size of the follicle itself (up to approximately 25-30 mm). The preantral phase is controlled mainly by locally produced growth factors through autocrine/paracrine mechanisms. The second phase is regulated by FSH and LH as well as by growth factors. There is great interest in the growth factors because they can stimulate cell proliferation and modulate gonadotropin action. The challenge now facing the field is to determine how ovary growth factor pathways negatively or positively regulate folliculogenesis, ovulation, and luteogenesis.
CHRONOLOGY
The timetable for human folliculogenesis is illustrated in Figure 1. In each menstrual cycle, the dominant follicle that ovulates originates from a primordial follicle that was recruited almost one year earlier (1). The preantral or Class 1 phase is divided into three major stages: the primordial, primary, and secondary follicle stages. Altogether, the development of a primordial to a full-grown secondary follicle requires about 290 days or about 10 regular menstrual cycles. The antral phase is typically divided into four stages: the small (Class 2, 3, 4, 5), medium (Class 6), large (Class 7), and preovulatory (Class 8) Graafian follicle stages. After antrum formation occurs at the Class 3 stage (~0.4mm in diameter), the rate of follicular growth accelerates. The time interval between antrum formation and the development of a 20 mm preovulatory follicle is about 60 days or about 2 menstrual cycles. A dominant follicle is selected from a cohort of class 5 follicles at the end of the luteal phase of the cycle (1). About 15 to 20 days are therefore required for a dominant follicle to grow to the preovulatory stage. Atresia can occur after the Class 1 or secondary follicle stage, with the highest incidence occurring in the pool of small and medium (Class 5, 6, and 7) Graafian follicles (Fig. 1).
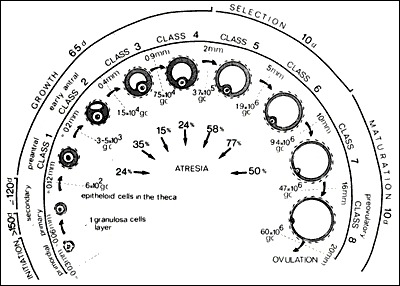
Figure 1
The timetable of normal folliculogenesis in women. Class 1: the preantral or gonadotropin-independent period. It takes ~290 days for a recruited primordial follicle to grow to a fully-grown secondary follicle. Class 3-8: the antral (Graafian) or gonadotropin-dependent period. From cavitation or beginning antrum formation, it takes ~60 days to pass through the small (Class 2-4) medium (Class 5, 6) and large (Class 7) and preovulatory (stage 8) Graafian follicle stages. A dominant follicle is selected from a cohort of class 5 follicles. Once selected, it requires ~20 days for a dominant follicle to reach the ovulatory stage. Atresia can occur in developing follicles after the secondary stage. Number of granulosa cells (gc); days (d). (Gougeon A: Dynamics of follicular growth in the human: A model from preliminary results. Hum Reprod 1:81, 1986. Reproduced with permission from Oxford University Press.)
THE PROCESS
The process of folliculogenesis occurs within the cortex of the ovary (Fig. 2). Folliculogenesis can be regarded as a process of attaining successively higher levels of organization by means of cell proliferation and cytodifferentiation. It includes four major developmental events: 1) primordial follicle recruitment; 2) preantral follicle development; 3) selection and growth of the antral follicle; and 4) follicle atresia.
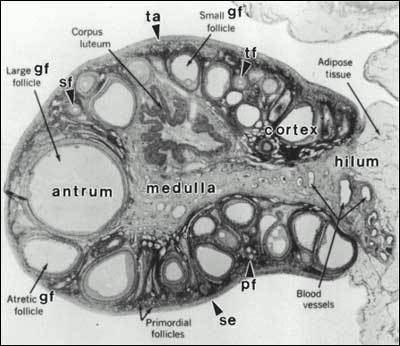
Figure 2
The adult ovary can be subdivided into three regions: the cortex, medulla, and hilum regions. The cortex consists of the surface epithelium (se), tunica albuginea (ta), ovarian follicles (primordial, primary (pf), secondary (sf), small, medium, large Graafian follicle (gf)) and corpora lutea (cl). The medulla consists of large blood vessels and nerves. The hilum contains large spiral arteries and the hilum or ovary Leydig cells. (Bloom W, Fawcett DW: A Textbook of Histology. Philadelphia, WB Saunders Company, 1975)
The Primordial-to-Primary Follicle Transition
Primordial follicles are considered the fundamental reproductive units of the ovary because they give rise to all dominant follicles, and therefore to all menstrual cycles. The entry of an arrested primordial follicle into the pool of growing follicles is termed recruitment or primordial follicle activation. To understand recruitment, it is necessary to understand the structure-function relationships of the primordial follicle.
The primordial follicle
Histologically, a primordial follicle contains a small primary oocyte (~ 25µm in diameter) arrested in the dictyate stage of meiosis, a single layer of flattened or squamous granulosa cells closely apposed to the oocyte, and a basal lamina (Fig. 3). By virtue of the basal lamina, the granulosa cells and oocyte exist within a microenvironment in which direct contact with other cells does not occur. Primordial follicles do not have an independent blood supply and thus have limited access to the endocrine system (2).

Figure 3.A
human primordial follicle. The oocyte with its germinal vesicle (GV) or nucleus is surrounded by a single layer of squamous granulosa cells (GC), both of which are enclosed within a basal lamina. The diameter of a human primordial follicle is ~30 µm. (Erickson GF: The Ovary: Basic Principles and Concepts. In Felig P, Baxter JD, Frohman L (eds.): Endocrinology and Metabolism. New York, McGraw-Hill, 1995. Reproduced with permission from the McGraw-Hill Companies.)
Based on work in mouse models, folliculogenesis specific basic helix-loop-helix (FIGLA) was identified as an oocyte-specific transcription factor critical for formation of primordial follicles at birth (3). In the absence of this protein, oocytes in newborn mice do not survive, resulting in female sterility. Forkhead box L2 (Foxl2) is a transcription factor expressed in pre-granulosa cells that is required to promote organization of somatic cells around primordial oocytes to form primordial follicles (4). Premature ovarian failure in women is associated with mutations in both FIGLA and FOXL2, suggesting that these proteins are also important in human folliculogenesis (5-7).
All primordial follicles (oocytes) are formed in the human fetus between the sixth and the ninth month of gestation (Fig. 4). As a result, all oocytes capable of participating in reproduction during a woman's life are present in the ovaries at birth. The number of oocytes or primordial follicles in a woman's ovaries constitutes her ovarian reserve. The idea that oocytes and follicles can arise de novo from stem cells after birth and thereby contribute to a woman’s ovarian reserve was proposed based on experiments in the mouse; however this theory is not generally accepted (8-10).
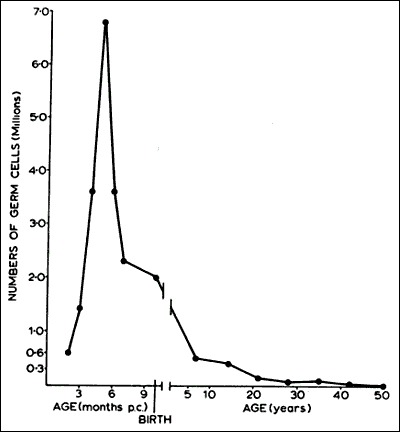
Figure 4
In human females, all primordial follicles are formed in the fetus between 6 and 9 months' gestation. During this period, there occurs a marked loss of oocytes due to apoptosis. The number of primordial follicles decreases progressively as a consequence of recruitment, until very few if any are present after the menopause at ~50 years of age. (Baker TG: Radiosensitivity of mammalian oocytes with particular reference to the human female. Am J Obstet Gynecol 110:746, 1971. Reproduced with permission from Mosby, Inc.)
Recruitment/Primordial Follicle Activation
Some primordial follicles are recruited to grow soon after their formation in the fetus. A change in shape from squamous to cuboidal, and the acquisition of mitotic potential in the granulosa cells are histological hallmarks of recruitment (Fig. 5). These changes are followed by growth of the oocyte. The process of recruitment continues at a relatively constant rate during the first three decades of a woman's life. At the same time, the rate of loss of non-growing follicles to atresia is continuously accelerating. Consequently, there occurs an overall loss of ovarian reserve that results in decreased fecundity by age 30 and a marked decrease by age 35 (11, 12).
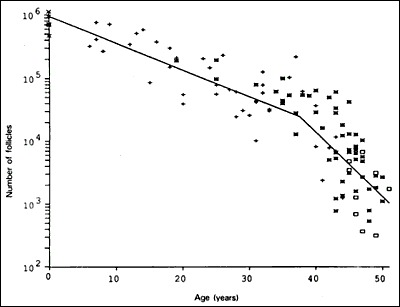
Figure 5
Photomicrographs of the early stages of human preantral folliculogenesis. A) Primordial follicle; arrow, squamous granulosa cell. B) Recruitment showing the primordial-to-primary transition; arrow, cuboidal granulosa cell. C) Primary follicle with multiple cuboidal granulosa cells. D) fully grown primary follicle at the primary-to-secondary transition stage; arrow, formation of a secondary layer of granulosa cells. All photos are 40x.
Paracrine communication between the oocyte, its associated granulosa cells, adjacent thecal/interstitial cells, and the surrounding follicles all combine to control primordial follicle recruitment. This communication is largely mediated by secreted growth factors including several members of the transforming growth factor-beta (TGF) superfamily. A schematic representing aspects of paracrine control of recruitment is shown in Figure 6.

Figure 6
Regulation of primordial follicle activation. Primordial follicle activation is driven by the collective actions of primordial follicles themselves, surrounding mesenchymal cells, surrounding follicles, and endocrine factors. The model shown is based mainly on experimental evidence from rodent model systems. PTEN, Foxo3a, and SDF-1 generated by primordial oocytes restrain their own activation. Primordial oocytes secrete PDGF and bFGF that stimulate pre-granulosa cells to increase secretion of KL and promote recruitment of mesenchymal cells to the follicle. KL secreted by pregranulosa cells promotes oocyte growth and follicle activation, and promotes recruitment of mesenchymal cells. KGF, BMP-4, and BMP-7 secreted by surrounding mesenchymal cells stimulate follicle activation. AMH and possibly SDF-1 secreted from surrounding growing follicles negatively regulate primordial follicle activation. Insulin from the circulation may promote follicle activation. In primary follicles, granulosa cells continue to secrete KL, which further promotes follicle activation. Oocytes of primary follicles secrete GDF-9 and BMP-15, which promote granulosa cell proliferation, KL expression and theca formation. PTEN, phosphatase and tensin homolog; Foxo3a; forkhead box O3A; SDF-1, stromal derived factor-1; PDGF, platelet-derived growth factor; bFGF, basic fibroblast growth factor; KL, kit ligand; KGF, keratinocyte growth factor; BMP, bone morphogenetic protein; AMH, anti-Mullerian hormone; GDF-9, growth differentiation factor-9.
Evidence from the mouse suggests that the oocyte itself is largely responsible for controlling the signaling pathways that regulate recruitment and the rate of follicular growth (13), and at least two major signaling pathways are involved. First, phosphatidyl inositol 3-OH-kinase (PI3K)-AKT-Foxo3a signaling is required to restrain follicle activation (14). Evidence for this is provided by studies of PTEN (phosphatase and tensin homolog), a lipid phosphatase that inhibits signaling by the PI3K pathway. Loss of PTEN in oocytes causes global activation of primordial follicles, suggesting that PTEN-mediated inhibition of PI3K signaling is required for preventing follicle activation (15). Similarly, mice null for Foxo3a, a transcription factor produced in both oocytes and granulosa cells, undergo global primordial follicle activation postnatally (16). The mammalian target of rapamycin (mTOR) signaling pathway also regulates follicle activation. A complex of the tuberous sclerosis proteins, TSC1 and TSC2, tonically inhibits mTOR signaling in oocytes, preventing activation of primordial follicles (17). In addition to these two signaling pathways, stromal derived factor-1 (SDF-1), a chemokine secreted by oocytes, appears to act in an autocrine/paracrine fashion to inhibit follicle activation (18).
Many oocyte and granulosa cell proteins have now been identified as critical for primordial follicle survival and recruitment. Oocyte-specific proteins include spermatogenesis and oogenesis specific basic helix-loop-helix 1 (Sohlh1), Sohlh2, and FIGLA, which are helix-loop-helix transcription factors that appear to promote primordial follicle survival and activation by regulating expression of critical downstream genes (3, 19-21). Similarly, the homeobox transcription factors LHX8 and NOBOX control the expression of several oocyte genes that regulate follicle development, including the TGF family members growth differentiation factor-9 (GDF-9) and bone morphogenetic protein-15 (BMP-15). Oocyte-derived GDF-9 and BMP-15 promote granulosa cell proliferation, but do not appear to do so until the primary follicle stage. Platelet derived growth factor (PDGF) and basic fibroblast growth factor (bFGF) are oocyte-secreted factors that positively regulate follicle activation and increase granulosa cell expression and secretion of kit ligand. Granulosa cell-derived kit ligand interacts with the c-kit tyrosine kinase receptor on the oocyte to promote oocyte growth and is required for primordial follicle activation (22, 23). There is evidence that kit ligand-mediated activation of signaling via the oocyte kit receptor leads to inactivation of Foxo3a and loss of this restraint as follicle activation occurs (24, 25).
Several factors derived from outside of the immediate oocyte-granulosa cell follicle unit also regulate the primordial to primary follicle transition. Keratinocyte growth factor (KGF, also known as fibroblast growth factor-7), BMP-4, and BMP-7 are secreted by stromal cells surrounding the primordial follicle and promote the primordial to primary follicle transition (26-28). Adjacent follicles negatively regulate follicle activation via intra-ovarian paracrine mechanisms. Anti-Müllerian hormone (AMH) secreted by granulosa cells of growing follicles negatively regulates recruitment (18, 29, 30).
The endocrine system may also modulate primordial follicle activation. There is evidence from the rat that insulin promotes primordial follicle activation (31). Pituitary gonadotropins are not absolutely required for primordial follicle activation because follicular growth to the primary follicle stage occurs in hypophysectomized animals (32). Furthermore, human ovarian xenografts transplanted into mice lacking pituitary gonadotropins develop follicles with up to two layers of granulosa cells (33). However, it is possible that FSH or other pituitary factors indirectly facilitate primordial follicle activation, and studies of hypophysectomized rhesus monkeys demonstrate a role for pituitary factors in germ cell survival (32).
The Primary Follicle
A primary follicle is defined by the presence of one or more cuboidal granulosa cells that are arranged in a single layer surrounding the oocyte (Fig. 5, 7). The major developmental events that occur in the primary follicle include FSH receptor expression and oocyte growth and differentiation.

Figure 7
Diagram illustrating the major histological changes that accompany the gonadotropin-independent period of preantral folliculogenesis (Erickson, Gregory F. Normal ovarian function. Clin Obstet Gynecol 21:31, 1978. Reproduced with permission from Lippincott-Raven Publishers.)
FSH receptor expression
Granulosa cells begin to express FSH receptors at the primary follicle stage (34). Stimulators of FSH receptor expression include FSH itself, activin, cyclic AMP, and TGF(35) (Fig. 8). Although follicle recruitment and the initial stages of follicle growth are independent of gonadotropins, FSH is required for primary follicle development to the preantral stage (33). In animals, high levels of plasma FSH accelerate primary follicle development (36). This raises the possibility that the age-related monotropic rise in FSH in women might affect events in the primary follicle.
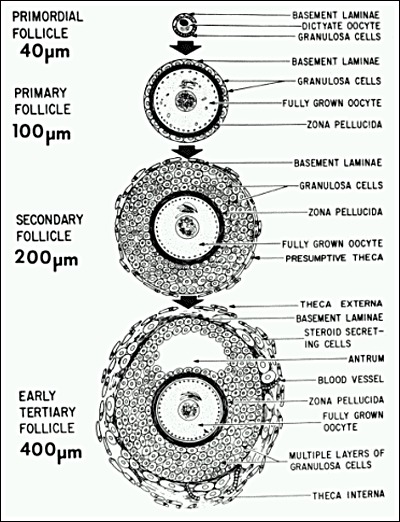
Figure 8
The early differentiation of the granulosa cells during preantral folliculogenesis involves the expression of FSH receptors. Animal studies support the concept that this process involves an activin autocrine/paracrine mechanism. (Erickson GF: Dissociation of Endocrine and Gametogenic Ovarian Function. In Lobo, R. (ed.): Perimenopause. Serono Symposia, Springer-Verlaag, 1997. Reproduced with permission from Springer-Verlag, New York.)
Oocyte growth and differentiation
Primary follicle development is accompanied by striking changes in the oocyte. During the preantral period, the oocyte increases in diameter from ~25 µm to ~120 µm and develops its surrounding extracellular matrix, the zona pellucida (ZP). This enormous growth occurs as a consequence of the reactivation of the oocyte genome (37). During the growth phase, the oocyte is highly transcriptionally active because it must generate sufficient proteins and mRNA transcripts to support its own growth as well as future critical processes of oocyte maturation, fertilization and early embryo development. Some oocyte transcripts are immediately translated and the resulting proteins contribute to ongoing oocyte growth and differentiation, while others required for future developmental processes are stored for later translation.
Mouse models have helped identify several oocyte-specific proteins important for oocyte growth. The transcription factors FIGLA and NOBOX serve as central regulators of the zona pellucida (ZP) proteins and the growth factors GDF-9 and BMP-15 (38, 39). GDF-9 is of particular interest because studies in rodents have demonstrated that GDF-9 plays an important role in stimulating granulosa cell proliferation and theca development (40). The general concept to emerge from this work is that novel growth factors produced by the oocyte play a crucial role in regulating preantral folliculogenesis via effects on the surrounding granulosa and theca cells (41, 42). Human oocytes express high levels of GDF-9 and BMP-15, and GDF-9 stimulates growth of human preantral follicles in vitro (43, 44). It follows, therefore, that preantral folliculogenesis will likely be determined by these oocyte growth factors. Interestingly, a dysregulation of oocyte GDF-9 expression has been implicated in polycystic ovarian syndrome (PCOS) in women (43), and a mutation of BMP-15 is associated with premature ovarian failure (45).
The kit ligand-c-kit tyrosine kinase receptor signaling pathway is critically important for oocyte growth and follicle development. Kit ligand generated by granulosa cells is required for oocyte growth (46). In addition, kit ligand is important for organizing theca cells around the growing follicle. Several growth factors appear to influence follicular growth by stimulating granulosa cells to increase expression of kit ligand. Kit ligand then stimulates oocyte growth and resulting additional production of growth factors. This results in a “feed-forward” mechanism of paracrine interactions supporting further development of follicles that have initiated the process.
Oocyte-granulosa cell connections
An important event in primary follicle development is the development of intimate intercellular connections between the oocyte and granulosa cells (47, 48). Both the oocyte and granulosa cells elaborate numerous cytoplasmic projections and microvilli that interdigitate with each other to create an extremely large surface area for diffusion (49). In addition, some of the follicle cell microvilli and cytoplasmic projections physically penetrate deeply into the oocyte via invagination of the oocyte plasma membrane, occasionally reaching close to the nuclear membrane. Cell-cell contacts comprised of adhesive junctions and gap junctions are established in these regions. Gap junctions, which are intercellular channels composed of proteins called connexins, directly couple adjacent cells allowing the diffusion of ions, metabolites, and signaling molecules (50).
Connexin 37 (Cx37) is the predominant gap junction protein synthesized by the oocyte after follicle recruitment, whereas granulosa cells mainly synthesize Cx43 (51, 52). Therefore heterotypic gap junctions comprised of Cx37 and Cx43 are formed between the oocyte and granulosa cells, whereas granulosa cells form homotypic Cx43 gap junctions between each other. The importance of oocyte-granulosa cell gap junctions was documented in rodents by the demonstration that ovaries of Cx37 deficient mice display a profound defect in oocyte and follicle growth resulting in failed folliculogenesis and female infertility (51). The underlying mechanism for these findings is that regulatory and nutrient molecules required for oocyte growth and acquisition of the potential to resume meiosis pass through these gap junctions from the granulosa cells to the oocyte (47, 53).
The Secondary Follicle
As preantral folliculogenesis continues, the structure of the follicle begins to change (Figs. 7 and 9). The major changes to occur during secondary follicle development include the accumulation of increased numbers of granulosa cells that form multiple layers around the oocyte, and the acquisition of a theca. The development of a primary to a fully grown secondary follicle results from an active autocrine/paracrine regulatory process that involves growth factors produced by the oocyte.

Figure 9. A
typical healthy secondary follicle. It contains a fully grown oocyte surrounded by the zona pellucida, 5 to 8 layers of granulosa cells, a basal lamina, a theca interna and externa with numerous blood vessels. (Bloom W, Fawcett DW In A Textbook of Histology. WB Saunders Company, Philadelphia 1975. With permission from Arnold.)
The primary-to-secondary transition
Secondary follicle development begins with the acquisition of a second layer of granulosa cells. This step is termed the primary-to-secondary follicle transition. It involves a change in the arrangement of the granulosa cells from a simple cuboidal epithelium to a stratified or pseudostratified columnar epithelium (Fig. 7). Experiments in animals have established that the primary/secondary stage is a critical regulated step in the process of folliculogenesis, e.g., follicle growth and development stop at the primary stage in mice and sheep in the absence of GDF-9 and BMP-15, respectively (54). These experiments have led to the concept that oocyte-derived GDF-9 and BMP-15 are obligatory for the primary/secondary transition, presumably through their ability to stimulate granulosa cell proliferation and/or their pattern of arrangement. Homotypic Cx43 gap junctions continue to develop between the granulosa cells as multiple layers form, resulting in an integrated and functional electrophysiological syncytium of communicating cells. Interestingly, folliculogenesis arrests at the primary/secondary transition in Cx43-deficient mice (55). These results imply that Cx43 coupling plays an indispensable role in the mechanisms controlling the formation of a secondary follicle.
Theca development
Secondary follicle development is also characterized by thecal development (56). At or about the time of the primary/secondary transition, several layers of stromal-like cells appear around the basal lamina. In the rat, some of these cells express a novel functional marker for differentiated theca cells, namely BMP-4 (57). This is an important finding because it indicates that the theca develops very early in folliculogenesis, i.e., at the primary/secondary transition stage. As secondary follicle development proceeds, two primary layers of theca appear; an inner theca interna that differentiates in the theca interstitial cells and an outer theca externa that differentiates into smooth muscle cells (56). Theca development is also accompanied by the neoformation of numerous small blood vessels, presumably through angiogenesis. Consequently, blood now circulates around the follicle, bringing nutrients and gonadotropins to, and waste and secretory products from, the developing follicle. At the completion of the preantral phase of folliculogenesis, a fully grown secondary follicle contains five distinct but interacting structural units: a fully grown oocyte surrounded by a zona pellucida, approximately 9 layers of granulosa cells, a basal lamina, a theca interna, a theca externa and a capillary net in the theca tissue (Fig. 9).
Oocyte meiotic competence
When the oocyte completes its growth during preantral folliculogenesis, it will spontaneously resume meiosis if removed from the follicle environment (58). However, fully-grown oocytes rarely resume meiosis during folliculogenesis. This has led to the concept that there exists a meiotic inhibiting mechanism controlled locally by the follicle cells. There is extensive evidence that cyclic AMP has a critical role in inhibiting meiosis resumption (59). For many years it was thought that cAMP entered oocytes from granulosa cells via gap junctions and that this process was disrupted when the oocyte was removed from the follicle, allowing meiotic maturation to occur. However, experiments in both rodent and human indicate that meiotic arrest is maintained by cAMP generated within the oocyte itself, and that transit of cAMP into the oocyte via gap junctions is not required (60-62). The new model is that a constitutively active G protein-coupled receptor, GPR3, persistently stimulates Gs protein to activate oocyte transmembrane adenylyl cyclases to generate high levels of cAMP within the oocyte (60, 61). There is now good evidence that cGMP, rather than cAMP, transits from cumulus cells to the oocyte across gap junctions (63). Within the oocyte, cGMP inhibits the function of phosphodiesterase 3A (PDE3A), preventing PDE3A-mediated cleavage of cAMP and in this way helping to maintain meiotic arrest. The cGMP is tonically generated in cumulus cells in response to estradiol-mediated production of natriuretic peptide precursor type C (NPPC) in mural granulosa cells (64). Secreted cleavage products of NPPC activate the guanylyl cyclase natriuretic peptide receptor 2 (NPR2) on cumulus cells, stimulating production of cGMP that crosses gap junctions into the oocyte.
The Antral Follicle
An antral follicle is characterized by a cavity or “antrum” containing fluid termed follicular fluid. Follicular fluid is a plasma exudate conditioned by secretory products from the oocyte and granulosa cells (65). It is the medium in which the granulosa cells and oocyte reside and through which regulatory molecules must pass on their way to and from this microenvironment. The onset of antrum development is characterized by the appearance of a fluid filled cavity at one pole of the oocyte (Fig. 10). In laboratory animals, two proteins expressed by the follicle itself are essential for antrum formation, namely granulosa-derived kit ligand and oocyte Cx37 (23, 51). If either of these proteins is absent, then no antral follicles develop and the female is infertile.

Figure 10
Photomicrograph of an early tertiary follicle 0.4 mm in diameter at the cavitation or early antrum stage. Oocyte (ooc); zona pellucida (ZP); granulosa cells (GC); basal lamina (BL); theca interna (TI); theca externa (TE); granulosa mitosis (arrowheads). (Revised from Bloom W, Fawcett DW In A Textbook of Histology. Philadelphia, WB Saunders Company, Philadelphia 1975. With permission from Arnold.)
Architecture
After the antrum forms, the basic plan of the antral follicle is established, and all the various cell types are present in their proper position awaiting the stimuli that lead to gradual growth and development (Fig. 11). An antral follicle is a member of the heterogeneous family of relatively large follicles that in human ovaries measure 0.4 to ~25 mm in diameter (66). The structure and organization of antral follicles remains essentially the same despite enormous growth and regardless of the stage of the menstrual cycle. The overall size of an antral follicle is determined largely by the size of the antrum, which in turn is determined by the volume of follicular fluid. Depending on follicle size, the volume of follicular fluid varies between 0.02 to 7 ml (Fig. 12). The proliferation of the follicle cells also contributes to follicle size. In a dominant follicle, the granulosa and theca cells proliferate extensively (as much as 100-fold) concomitant with the antrum becoming filled with follicular fluid (Fig. 13). Thus, increased follicular fluid accumulation and cell proliferation are responsible for the tremendous growth of the dominant follicle during the follicular phase of the cycle. It is the cessation of follicular fluid formation and mitosis that limits the size of the atretic follicle. An atretic follicle usually fails to develop beyond the small to the medium stage (1-10 mm). The relative abundance of antral follicles and their sizes vary as a function of age and the menstrual cycle. The total number of antral follicles present in a woman’s ovaries early in the menstrual cycle appears to be an indicator of her ovarian reserve (67). This “antral follicle count” can be determined by ultrasound and has been used clinically in infertile women to determine appropriate treatment protocols.

Figure 11
Diagram of the architecture of a typical Class 5 Graafian follicle. (Erickson GF: Primary cultures of ovarian cells in serum-free medium as models of hormone-dependent differentiation. Mol Cell Endocrinol 29:21, 1983. Reprinted with permission from Elsevier)

Figure 12
Changes in the number of granulosa cells and volume of follicular fluid in human Graafian follicles throughout the course of folliculogenesis. Note that the dominant follicle at ovulation measures ~25mm in diameter and contains ~50 million granulosa cells and 7 ml of follicular fluid. (McNatty KP: Hormonal correlates of follicular development in the human ovary. Aust J Biol Sci 34:249, 1981. Reproduced with permission from CSIRO Publishing.)
The theca externa (Fig. 13) consists of concentrically arranged smooth muscle cells, which are innervated by autonomic nerves (66). The physiological significance of the theca externa is unknown. The theca interna contains a population of large epithelioid cells termed the theca interstitial cells (Fig. 13). They have the ultrastructural characteristics typical of active steroid producing cells, i.e., the cytoplasm is filled with lipid droplets, smooth endoplasmic reticulum and mitochondria with tubular cristae (56). The theca interstitial cells possess receptors for LH and insulin. In response to LH and insulin stimulation, they produce high levels of androgens, most notably androstenedione (68). The theca interna is richly vascularized by a loose capillary network that surrounds the antral follicle during its growth.

Figure 13
Drawing of the wall of a Graafian follicle. (Bloom W, Fawcett DW In A Textbook of Histology. WB Saunders Company, Philadelphia 1975. With permission from Arnold.)
In the antral follicle, the granulosa cells and oocyte are distributed as a mass of precisely shaped and precisely positioned cells. This spatial organization gives rise to distinct subtypes of granulosa cells: the membrana, the periantral area, and the cumulus oophorus (Fig. 14). All the granulosa cells express FSH receptors during antral follicle development; however, each group of granulosa cells is influenced by its position to express a specific differentiated state in response to FSH stimulation. For example, the membrana granulosa cells express P450AROM and LH receptor whereas the periantral and cumulus cells do not (66).

Figure 14
An oocyte morphogen gradient influences granulosa cell phenotypes. The model shown is based mainly on experimental evidence from rodent model systems. Protein morphogens including GDF-9 and BMP-15 are secreted by the oocyte, resulting in a concentration gradient that diminishes with distance from the oocyte. Because granulosa cell differentiation is dictated by the morphogen concentration, position in the follicle relative to the oocyte is a critical determinant of the final phenotype. Additional modulation of granulosa cell phenotype is accomplished by other factors from the follicle itself and from the endocrine system, including activin, inhibin, and steroid hormones. Granulosa cells differentiate into three distinct phenotypes based on position within the follicle – cumulus, periantral, and membrana granulosa cells. Each granulosa cell type exhibits a distinct response to FSH stimulation. Some examples of the many genes differentially expressed in the various follicle compartments are listed at the right. Abbreviations: Cox-2, cyclooxygenase 2; FSH, follicle-stimulating hormone; HAS2, hyaluronic acid synthase 2; IGF-I, insulin-like growth factor I; PTX, pentraxin; TNFAIP6, tumor necrosis factor-induced protein 6; LH, luteinizing hormone; P450SCC, P450 side chain cleavage; P450AROM, P450 aromatase; u-PA, urokinase-type plasminogen activator. (Revised from Erickson GF, Shimasaki S: The role of the oocyte in folliculogenesis. Trends Endocrinol Metab 11:193, 2000.)
The way in which the granulosa cells differentiate in the antral follicles appears to be controlled by a morphogen gradient emanating from the oocyte (66). Studies with laboratory animals have demonstrated that growth factors produced by the oocyte act directly in granulosa cells to inhibit FSH-dependent cytodifferentiation (54, 69). As an antral follicle develops, oocyte morphogens including GDF-9 and BMP-15 function as gradient signals for the generation of distinct classes of functionally different granulosa cells depending on the positions of the granulosa cells relative to the oocyte. These differences become critically important as the follicle cells and oocyte prepare for ovulation.
Selection
In normal cycling women, the dominant follicle is selected from a cohort of class 5 follicles at the end of the luteal phase of the menstrual cycle (1). The rate of granulosa mitosis appears to increase sharply (~2 fold) in all cohort follicles after the mid-luteal phase, suggesting that luteolysis contributes somehow to an increase in mitosis in the granulosa cells in the pool of small antral follicles. The first indication that selection has occurred is that the granulosa cells continue dividing at a relatively fast rate in one cohort follicle while proliferation slows in the granulosa of the other cohort follicles. This effect is observed about the time of menses. Thereafter, the mitotic rate of the granulosa and theca cells remains high through the rest of antral follicle development. As the follicular phase proceeds, the selected “dominant” follicle grows rapidly, reaching 6.9 ± 0.5 mm at cycle days 1 to 5, 13.7 ± 1.2 mm at days 6 to 10, and 18.8 ± 0.5 mm at days 11 to 14. Conversely, growth proceeds more slowly in the other antral follicles of the cohort.
The underlying mechanism of selection involves the secondary rise in plasma FSH. During the menstrual cycle, the secondary FSH rise in women begins a few days before plasma progesterone falls to basal levels at the end of luteal phase. FSH levels remain elevated through the first week of the follicular phase of the cycle (Fig. 15). Increased and sustained levels of circulating FSH are obligatory for selection and female fertility. Decreased estradiol and inhibin A production by the corpus luteum (CL) are the major causes for the secondary rise in FSH and dominant follicle selection (Fig. 15).

Figure 15
The luteal-follicular transition in women. Data are mean (± SEM) for daily inhibin A, inhibin B, FSH, estradiol, and progesterone levels in the luteal-follicular transition of normal cycling women (n=5). Data are centered to the day of menses in cycle 2. (Welt CK, Martin KA, Taylor AE, et al: Frequency modulation of follicle-stimulating hormone (FSH) during the luteal-follicular transition: evidence for FSH control of inhibin B in normal women. J Clin Endocrinol Metab 82:2645, 1997. Reproduced with permission from The Endocrine Society.)
The secondary rise in FSH leads to a progressive increase in the follicular fluid levels of FSH in the microenvironment of the dominant follicle. In healthy class 5 to 8 follicles, the mean concentration of follicular fluid FSH increases from ~1.3 mIU/mL (~58 ng/ml) to ~3.2 mIU/ml (~143 ng/ml) through the follicular phase (66). By contrast, the follicular fluid levels of FSH are low or undetectable in the non-dominant cohort follicles. The entry of FSH into follicular fluid provides an obligatory induction for selection. An unanswered question in reproductive medicine concerns the mechanism whereby one cohort follicle has the capacity to concentrate (sequester?) high levels of FSH into its microenvironment.
Atresia
In mammals, 99.9% of the follicles (oocytes) die by atresia. A fundamental property of atresia is the activation of apoptosis in the oocyte and granulosa cells. Apoptosis is a complex process involving signaling pathways coupled to programmed cell death (70). It can be initiated externally (extrinsic pathway) by ligand binding to cell surface “death receptor” signaling such as that induced by tumor necrosis factor (TNF) or Fas ligand. Intrinsic (intracellular) cell death pathways are mediated by alterations in mitochondrial outer membrane permeability that cause release of pro-apoptotic factors into the cytoplasm, and are typically controlled by B cell/lymphoma-2 (Bcl-2) family proteins. Both pathways result in activation of caspases, a family of cysteine aspartate-specific proteases, as the final mediators of programmed cell death.
Follicle atresia is controlled by a balance between pro-survival factors that promote cell proliferation, follicle growth and differentiation and pro-apoptotic factors that promote cell death. Follicle atresia and oocyte loss in adults appears to be initiated by apoptosis in the granulosa cells, unlike the massive loss of oocytes during fetal development that occurs via apoptosis within the oocyte (71). Both extrinsic and intrinsic cell death pathways appear to control apoptosis in granulosa cells.
The importance of FSH in supporting follicle growth after antrum formation and in preventing apoptosis has led to the concept that FSH is a survival factor for antral follicles (72). Aspects of the downstream signaling pathway induced by FSH that are important for follicle survival have been determined recently. FSH activates the PI3K signaling pathway in granulosa cells, causing phosphorylation of Akt (protein kinase B) that leads to an increase in cell survival proteins including members of the IAP (inhibitor of apoptosis) family and resulting in inhibition of the intrinsic cell death pathway. Oocyte-secreted factors also inhibit granulosa cell apoptosis. There is evidence from the rat that GDF-9 serves as a pro-survival factor in preantral follicles by activating the PI3K signaling pathway (73). In the bovine, oocyte-secreted BMP-15 and BMP-6 are important for maintaining cumulus cell survival (71).
At least three ligands of the tumor necrosis factor (TNF) family have roles in ovarian follicle atresia: TNF-alpha, Fas ligand, and TRAIL (TNF-related apoptosis-inducing ligand) (72). Other intra-ovarian pro-apoptotic factors include Apaf-1 (apoptotic protease-activating factor-1), nodal, a TGFfamly member found in granulosa cells of apoptotic follicles, and the p53 stress response gene (74-76). Prohibitin is a ubiquitous mitochondrial membrane protein that may mediate p53-induced apoptosis in granulosa cells (77, 78).
FSH ACTION IN THE GRANULOSA CELLS
FSH plays an obligatory role in the mechanisms of selection and dominant follicle development, and no other ligand by itself posses such regulatory activity. The primary mechanism by which FSH controls selection is by stimulating FSH receptor-mediated signal transduction pathways in the granulosa cells. Although LH is not essential for selection, it is certainly important in regulating dominant follicle formation through its capacity to stimulate the expression of the aromatase substrate, androstendione. To understand dominant follicle development during the cycle, one must understand the actions of FSH and LH in the granulosa and theca interstitial cells, respectively.
FSH Signaling
The FSH receptor is part of a large family of receptors known as seven-transmembrane receptors (7TMRs) that regulate the heterotrimeric G proteins (79). It is most closely related to the thyrotropin (TSH) and lutropin/choriogonadotropin (LH/CG) receptors, which together with the FSH receptor comprise the three best-characterized glycoprotein hormone receptors. The human FSH receptor contains 678 amino acids (MR 76,465). It is organized into three domains: 1) a large extracellular NH2-terminal ligand binding domain with six potential N-linked glycosylation sites and a cluster of cysteines at the junction between the extracellular and transmembrane domains, 2) the transmembrane spanning domain composed of seven hydrophobic alpha helices that anchor the receptor to the plasma membrane, known as the “heptahelical domain”, and 3) the intracellular COOH-terminal domain that has a relatively high proportion of serine and threonine residues. The regulated phosphorylation of the amino acids in the intracellular domain plays a role in desensitization and downregulation of the FSH receptor by facilitating arrestin association with the receptor and subsequent receptor internalization (80).
Traditional models of 7TMR signaling held that a ligand bound to the extracellular domain, causing a conformational change in the transmembrane region that led to activation of a single intracellular heterotrimeric G protein associated with the receptor via the COOH-terminus, i.e., a “one ligand/one receptor/one response” model. Recent work, however, has modified that view to include possible homodimeric and heterodimeric interactions between 7TMRs, including the FSH receptor. The new model proposes that receptor dimerization occurs and can modulate the intracellular response to ligand activation and the efficiency of receptor internalization (81). Indeed, there is evidence that “negative cooperativity” can occur between homodimerized glycoprotein receptors such that ligand binding to one receptor can diminish the ability of a second ligand to activate the second receptor in the dimer (82). This additional level of complexity in 7TMR signaling may help explain the variety of cellular responses to different amounts and combinations of glycoprotein hormones.
The importance of the heptahelical domain in controlling levels of FSH receptor functional activity has been documented recently. Several different mutations in the heptahelical domain were identified in women with spontaneous ovarian hyperstimulation syndrome (83). These mutations result in some constitutive activity in the absence of ligand and also higher sensitivity of the receptor to related glycoprotein ligands such as chorionic gonadotropin. This work led to the identification of FSH receptor polymorphisms that may be associated with a predisposition to more severe iatrogenic ovarian hyperstimulation syndrome during infertility treatment (84).
The primary and most extensively studied FSH signaling cascade in granulosa cells involves stimulation of cyclic AMP (cAMP) production and subsequent activation of cAMP-dependent protein kinase (PKA) (Fig. 16). The signaling pathway is initiated when FSH binds to its receptor and induces a conformational change in the transmembrane portion of the receptor. This change leads to activation of the heterotrimeric G protein, Gs, by exchange of GTP for the GDP bound to the alpha subunit. The active Gs-GTP subunit dissociates from the G subunit complex and interacts with adenylate cyclase to generate cAMP. Cyclic AMP subsequently binds to the regulatory subunits of PKA, causing dissociation into a regulatory subunit dimer and two free catalytic subunits. The catalytic subunits phosphorylate serine and threonine residues of target proteins, including the transcription factors CREB and CREM. After phosphorylation, these transcription factors can bind to upstream DNA regulatory elements called cAMP response elements (CRE) where they act to regulate gene activity. FSH control of differential gene activity via the cAMP/PKA pathway in granulosa cells is largely responsible for the process of dominant follicle growth and development to the preovulatory stage.

Figure 16
Diagram of the FSH signal transduction pathway in granulosa cells of a dominant follicle. FSH interacts with a receptor protein that has seven transmembrane spanning domains. The binding event is transduced into an intracellular signal via the heterotrimeric G proteins. The active aGstimulating (aGs-GTP) protein interacts with its effector protein, adenylate cyclase, to initiate cAMP formation. cAMP binds to and activates protein kinase A, which in turn phosphorylates substrate proteins that stimulate transcription of the genes encoding P450AROM and LH receptor as well as activate mitosis and follicular fluid formation. (Revised from Erickson, GF: Polycystic Ovary Syndrome: Normal and Abnormal Steroidogenesis. In Schats R. and Schoemaker J (eds): Ovarian Endocrinopathies: Proceedings of the 8th Reinier deGraaf Symposium. Parthenon Publishing, 1994)
Recent work has indicated that FSH activates signaling pathways in addition to the primary cAMP/PKA pathway (85). As mentioned previously, FSH signals via the PI3K pathway to activate pro-survival factors in granulosa cells. FSH receptor signaling can also activate Src family tyrosine kinases that modulate the activity of several downstream signaling pathways including those involving epidermal growth factor receptor tyrosine kinase, the low molecular weight G protein RAS, extracellular related kinases (ERK1/2), p38 mitogen-activated protein kinase, and PI3K (86, 87). Crosstalk between these multiple signaling pathways generates the final downstream phenotypic responses of granulosa cells to FSH signals.
Stimulation of Mitosis
A sustained period of rapid proliferation of the granulosa cells is characteristic of the developing dominant follicle. During the follicular phase of the menstrual cycle, the number of granulosa cells increases from about 1 x 106 cells at follicle selection to over 50 x 106 cells at the preovulatory stage (Fig. 12). In women, FSH is a key stimulator of granulosa cell proliferation (88). In addition, several locally produced polypeptides and growth factors influence granulosa cell proliferation, in part by modulating FSH action. For example, oocyte-derived GDF-9, BMP-15 and BMP-6, and granulosa/theca cell-derived activin, TGF isoforms, and estradiol stimulate granulosa cell proliferation, whereas locally produced AMH inhibits proliferation (89, 90). Therefore FSH probably acts with growth factors to regulate proliferation of human granulosa cells.
Expression of Aromatase
As the dominant follicle grows, the granulosa cells acquire the potential to produce large amounts of estradiol. The FSH-mediated induction of P450AROM ( CYP19 gene) expression in the granulosa cells is causal to the acquisition of the estrogen potential of the follicle (91). P450AROM is detected when a follicle reaches ~1 mm in diameter or the class 2 stage, and it is seen only in the dominant follicle. P450AROM activity increases progressively, reaching very high levels in the granulosa cells of the preovulatory follicle in the late follicular phase (92-94). The type I 17-hydroxysteroid dehydrogenase (17-HSD) is constitutively expressed in granulosa cells in follicles from the primary to the preovulatory stage (95-97). By virtue of the expression of P450AROM and 17-HSD, the granulosa cells become highly active in converting theca-derived androstenedine to estradiol. It is the progressive increase in the level of P450AROM gene expression that makes it possible for the dominant follicle to secrete the increasing amounts of in estradiol during days 7 to 12 of the menstrual cycle.
Luteinization Potential
During the follicular phase, the granulosa cells also acquire the potential to produce increasing amounts of progesterone. Several operative processes are involved in the acquisition of luteinization potential. To begin with, the continued stimulation of the granulosa cells by FSH is involved in this progressive process. When luteinization actually occurs, granulosa cells express large amounts of steroidogenic acute regulatory protein (StAR), P450 side chain cleavage (P450SCC) and 3-hydroxysteroid dehydrogenase (3-HSD). Despite the fact that a progressive increase in the potential for luteinization occurs, this process remains suppressed until just prior to ovulation. It is clear from studies in animals that the inhibition is caused by oocyte-derived luteinization inhibitors present in follicular fluid (66, 98). These luteinization inhibitors include GDF-9, BMP-6, and BMP-15. Thus, it seems likely that the potential of the antral follicle to luteinize is determined by FSH action on the granulosa cells; however, the process is inhibited by oocyte-derived luteinization inhibitors that operate to specifically repress the expression of StAR, P450SCC and 3-HSD in the granulosa cells during folliculogenesis.
LH Receptor Expression
Competence of the preovulatory follicle to respond to the inductive stimulus of the LH surge by undergoing ovulation involves the expression of LH receptors in the granulosa cells. FSH plays a vital role in LH receptor induction in the granulosa cells. Similar to StAR, P450SCC and 3-HSD, the expression of LH receptors remain suppressed until late in the follicular phase of the cycle. There is compelling evidence in laboratory animals that oocyte-derived inhibitors inhibit FSH-induced LH receptors in granulosa cells (66, 69). The interpretation is that the oocyte is responsible for inhibiting the expression of granulosa LH receptors in the developing antral follicle until the onset of the preovulatory stage.
LH ACTION IN THECA INTERSTITIAL CELLS
LH Signaling
The human LH receptor is a glycoprotein of 675 amino acids with a predicted molecular mass of about 75 kDa. The mature form of the receptor found on the cell surface, however, has an apparent Mr of 85-95 kDa because it undergoes glycosylation during transit to the cell surface (99). Conversion of the immature to the mature form of the receptor occurs slowly, and this process appears to be regulated as a mechanism of controlling expression of the mature receptor on the cell surface (99). The LH receptor is a G protein-coupled 7TMR structurally very similar to the FSH receptor. It has a long extracellular leucine rich ligand binding domain, a heptahelical transmembrane domain, and an intracellular domain responsible for G protein interactions. The intracellular domain contains consensus sites for protein kinase C (PKC) phosphorylation. A number of truncated forms of the LH receptor have been identified in which the transmembrane domain is absent. Accordingly, the truncated LH receptors may be extracellular, perhaps being secreted from the cells. We do not know if these shorter variants of LH receptor actually bind LH, but if they do they could affect the levels of free LH and thereby modulate cellular responses to LH signals.
Like the FSH receptor, LH receptors are coupled to G proteins (Fig. 17). LH binds to its receptor with high affinity; and the binding event initiates a conformational change in the receptor that in turn activates the G proteins. Downstream effects of LH receptor activation, like FSH receptor activation, are mainly mediated by the GS/adenylate cyclase/cAMP/PKA pathway, resulting in phosphorylation of target proteins including CREB and CREM that modulate gene transcription. There is also evidence that other G proteins, including Gi and Gq, may be coupled to the LH receptor and mediate LH-induced activation of phospholipase C and its downstream signaling pathways (99). In any case, the second messenger molecules in the LH signaling pathway are involved in the activation of the genes in the biochemical pathway that eventually lead to androstenedione biosynthesis (Fig. 17).

Figure 17
Diagram showing the regulatory mechanisms of androgen production by theca interstitial cells. The principal endocrine regulators of androstenedione production are LH, insulin, and lipoproteins. The LH receptor/cAMP/PKA signaling pathway leads to the induction of specific genes (broken lines) in the androstenedione biosynthetic pathway. Insulin receptor/protein tyrosine kinase (PTK) signaling can cause marked increases in this response. Lipoproteins are potent stimulators of theca androgen production by virtue of their ability to increase intracellular cholesterol which in turn is transferred to P450C22 via StAR. (Erickson, GF. Normal regulation of ovarian androgen production. Semin Reprod Endocrinol 11:307, 1993. Reproduced with permission from Thieme Medical Publishers.)
Stimulation of Androgen Production
At about the time of antrum formation, the theca interstitial cells begin to express their differentiated state. This event involves the expression of a battery of proteins, including LH receptors, insulin receptors, lipoprotein (HDL, LDL) receptors, StAR, P450SCC, 3-HSD, and P450c17. By virtue of the expression of these genes, the theca interstitial cells have the capacity to produce androstenedione (Fig. 17). It is significant that the theca of all antral follicles (class 1 to 8) express this differentiated state. This implies all antral follicles have the potential to produce androstenedione, a concept supported by the presence of high levels (~1 ng/ml) of androstenedione in follicular fluid in developing antral follicles (100). LH is the most important effector of theca interstitial cytodifferentiation, but insulin and lipoproteins can act in synergy with LH to amplify this process.
A variety of other regulatory ligands and growth factors have been identified that modulate mammalian theca androgen production, including insulin, IGF-I, lipoprotein, activin, inhibin, GDF-9, and BMP-4 (101-107). Except for insulin, the significance of these regulatory molecules is unclear. Insulin receptors are expressed in human theca cells and the ability of the insulin receptor signal transduction pathways to stimulate androstenedione production has been demonstrated. Insulin by itself can increase androstenedione production, and importantly insulin can synergize with LH to further increase androgen biosynthesis (Fig. 17). The idea that insulin has functional significance is demonstrated by the fact that hyperinsulinemia can result in hyperandrogenism in some women. Observations in rodents indicate that LDL and HDL also stimulate steroidogenesis by human theca interstitial cells (TIC), and importantly they can cooperate with LH to cause further increases (Fig. 17). Whether increased androgen production by LDL and/or HDL has any physiological meaning is not clear. Nonetheless, it is noteworthy that HDL is the most potent stimulator of theca androgen production known so far. Finally, activin and inhibin can inhibit and stimulate, respectively, androgen production by human TIC in vitro. Recent studies have found that GDF-9 and BMP-4 interact with cultured human theca cells to inhibit androgen biosynthesis. How any of these observations fit into human physiology and pathophysiology is unknown.
TWO-CELL TWO-GONADOTROPIN CONCEPT
The physiological mechanism by which the dominant follicle produces estradiol is called "the two-cell two-gonadotropin concept" (Fig. 18). The delivery of LH to the theca interstitial cell leads to the synthesis and secretion of androstenedione. The amount of androgen secretion will reflect the presence within the theca of other regulatory molecules including insulin, IGF-I, lipoproteins, activin, and inhibin. Some androstenedione diffuses into the follicular fluid where it accumulates at very high concentrations. In response to the P450AROM induced in the granulosa cells by FSH stimulation, androstenedione is aromatized to estrone, which then is converted to estradiol by 17-HSD.

Figure 18
Diagram showing the "Two Gonadotropin-Two Cell Concept" of follicle estrogen production. (Erickson, GF: Normal ovarian function. Clin Obstet Gynecol 21:31, 1978. Reproduced with permission from Lippincott-Raven Publishers.)
OVULATION
On or about the fourteenth day of an idealized 28-day menstrual cycle, the preovulatory follicle releases a mature egg enclosed within a cumulus complex for possible fertilization. This process, termed ovulation (Fig. 19), requires the collective actions of the endocrine system, immune signals, and intraovarian paracrine factors. The distinct cellular compartments in the preovulatory follicle — the oocyte, cumulus granulosa cells, mural granulosa cells, and theca cells — have dramatically different but strictly coordinated responses to the hormonal and other signals controlling ovulation.
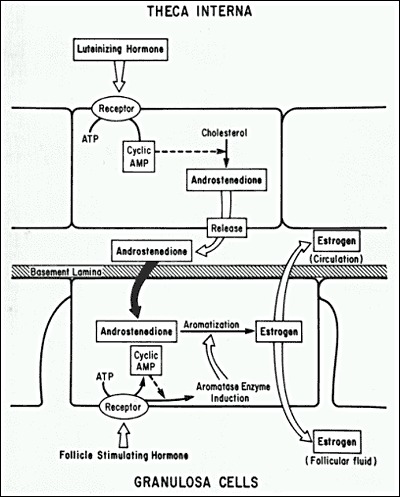
Figure 19
Photomicrograph of ovulation of a mature egg-cumulus complex through the stigma. (Hartman CG, Leathem JH In Conference on Physiological Mechanisms Concerned with Conception. Pergamon Press, New York 1959)
MURAL GRANULOSA CELLS: DIFFERENTIATION
During antral follicle development, suppression of LH receptor expression in cumulus granulosa cells by oocyte morphogen gradients results in much higher expression of LH receptors in the mural granulosa cells. For this reason, the mural granulosa cells serve as the follicle compartment largely responsible for transducing the ovulatory LH signal. As mentioned above, LH activates the Gs/cAMP/PKA signaling pathway to induce transcriptional responses but also activates other signaling pathways including ERK and mitogen-activated protein kianse (MAPK). In response to the LH surge, these signaling pathways rapidly and dramatically induce changes in the mural granulosa cell gene expression profile. In particular, the transcription factors CREB, Sp1 and Sp3 are generated and direct transcription of additional transcriptional regulators including the progesterone receptor (PR), early growth response-1 (Egr-1), and CAAT-enhancer binding protein (C/EBP) (108). Peroxisome proliferator-activator receptor (PPAR), a PR-regulated member of the nuclear receptor superfamily, is actively transcribed in mural granulosa cells after the LH surge and is required for ovulation in the mouse model (109). This finding is of particular interest because of evidence that PPAR abnormalities may contribute to the PCOS phenotype in women (110). Together, these transcriptional regulators drive the production of a cohort of proteins critical for ovulation (Fig. 20).

Figure 20
Signaling pathways and mediators of ovulation. Ovulatory surges of FSH and LH stimulate cells from multiple follicle compartments to transduce signals that collectively lead to ovulation. LH signaling is transduced by thecal and mural granulosa cells, whereas FSH signaling is transduced by mural granulosa and cumulus cells. Thecal cells (or leukocytes within the theca layer) secrete IL-1, a cytokine that stimulates cumulus cell expansion, and InsL3, a peptide that may trigger a reduction in oocyte cAMP levels. Mural granulosa cells secrete EGF-like ligands, growth factors that transduce the ovulatory response to the cumulus cells. In addition, they secrete versican and AdamTS-1, both of which become components of the cumulus cell matrix. Inter--trypsin inhibitor from the bloodstream similarly relocates to form part of the cumulus cell matrix. PGE2 generated by cumulus cells acts in an autocrine fashion to promote cAMP production that enhances ovulatory signaling cascades. Oocyte morphogens including GDF-9, BMP-15, and BMP-6, along with multiple other signaling molecules generated both within and outside the follicle, modulate cumulus cell, mural granulosa cell, and theca cell responses to ovulatory signals. IL-1, interleukin-1; InsL3, insulin-like peptide 3; Egf-L, EGF-like ligands; II, inter--trypsin inhibitor; PGE2, prostaglandin E2.
Although numerous proteins generated in the mural granulosa cells are probably required for successful ovulation, a few that have key defined roles are outlined here. The protease ADAMTS-1 is synthesized in mural granulosa cells but is secreted and becomes localized within the cumulus cell complex where it functions to cleave extracellular matrix proteins (111). One of its substrates, versican, is also generated in the mural granulosa cells but relocates to the cumulus cell complex in the periovulatory period. The epidermal growth factor (EGF)-like ligands, amphiregulin, betacellulin, and epiregulin, are synthesized as transmembrane mural granulosa cell proteins but the extracellular portion is cleaved and subsequently these factors transduce the ovulatory signal to the cumulus complex via EGF receptors located on the cumulus cells (112). The phosphodiesterase PDE4D is the major isoform found in mural granulosa cells and is responsible for degrading cAMP so that premature luteinization does not occur.
CUMULUS CELLS: EXPANSION
Cumulus cells undergo a distinct differentiation response to the LH surge as compared to the mural granulosa cells, in part because they express much lower levels of the LH receptor. Instead of responding directly to LH, the cumulus cells receive the ovulatory stimulus indirectly via diffusible factors, including the EGF-like ligands, from the mural granulosa cells. In addition, FSH signaling via cumulus cell FSH receptors coupled to the cAMP/PKA and PI3K signaling pathways appears to facilitate expression of genes important for ovulation. Prostaglandins also are key mediators of ovulation. In particular, prostaglandin E2 (PGE2) is rapidly generated by cumulus cells via induction of cyclooxygenase-2 (COX-2) gene expression. PGE2 then acts in an autocrine fashion to stimulate its cognate 7TMR to signal via the Gs/adenylate cyclase/cAMP pathway to augment expression of additional ovulatory mediators, including the EGF-like ligands (113). The importance of prostaglandins in human ovulation was demonstrated by two groups who found that COX-2 inhibitors delay ovulation in women (114, 115)(Fig. 20).
Diffusible factors from both theca cells and the oocyte also contribute to the final program of cumulus cell gene expression during ovulation. Interleukin-1 is released from cells, possibly leukocytes, within the theca compartment and acts in a paracrine fashion to induce cumulus cells to synthesize components of its extracellular matrix. The oocyte is responsible for secreting TGF family growth factors, including GDF-9 and BMP-15, that support differentiation of the cumulus cell phenotype and are permissive for the cumulus cell response to ovulatory stimuli (13). Together, input from the endocrine system and various follicle compartments results in the elaboration of a unique extracellular matrix between the cumulus cells in a process called “mucification” or “cumulus expansion” that is critical for successful ovulation and fertilization (Fig. 21).
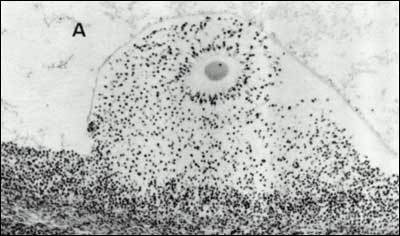
Figure 21
Photomicrograph showing the effects of the LH/FSH surge on egg-cumulus expansion in situ. The oocyte has resumed meiosis, the corona radiata is radiating from the zona pellucida, and the cumulus, (but not the membrana and periantral), granulosa cells have lost their attachments and appear as individual cells dispersed within the proteoglycan matrix, due to mucification. (Testart J, Thebault A, Frydman R, Papiernik E: Oocyte and Cumulus Oophorus Changes Inside the Human Follicle Cultured with Gonadotropins: In Hafez ESE, Semm K (eds) In Vitro Fertilization and Embryo Transfer. Alan R. Liss Inc., 1982 with permission from Jacques Testart)
The cumulus cell matrix is comprised largely of long chains of hyaluronan generated by the activity of hyaluronan synthase 2. The hyaluronan chains are linked by proteins derived from distinct compartments: TNF-induced protein 6 from cumulus cells, versican from mural granulosa cells, and the heavy chains of inter--trypsin inhibitor derived from the serum. Other components of the cumulus cell matrix include pentraxin 3, which is required for matrix stability and retention after ovulation, and ADAMTS-1, a protease involved in matrix remodeling (111, 116). The viscoelastic properties of the cumulus cell matrix are central to successful ovulation, ovum pickup by the fallopian tube, and penetration by the sperm to reach the egg proper.
OOCYTE: MEIOTIC MATURATION
After a prolonged resting state, the oocyte in the preovulatory follicle resumes meiosis during the ovulation sequence. The oocyte nucleus, known as the “germinal vesicle”, undergoes a series of changes that involve germinal vesicle breakdown (GVBD), emission of the first polar body, and progression of meiosis to the second meiotic metaphase. Meiosis is arrested here and proceeds no further unless the ovulated egg is fertilized. Meiotic maturation is a vital event in ovulation because it is obligatory for normal fertilization.
As discussed earlier, new data from the mouse model indicates that high concentrations of intra-oocyte cAMP generated by the constitutive activity of GPR3 inhibit meiotic maturation (60, 117). At the same time, cGMP crosses gap junctions from cumulus cells into the oocyte and prevents oocyte PDE3A from degrading the cAMP (63). In response to the ovulatory LH surge, LH-receptor mediated signaling is activated in mural granulosa cells. The resulting increase in cAMP levels in these cells causes a reduction in cGMP production and initiates MAPK-mediated phosphorylation of connexin proteins, causing closure of the gap junctions (118). When cGMP stops entering the oocyte, PDE3A becomes active and cleaves cAMP, resulting in a reduction of PKA activity and meiosis resumption. There is also evidence that LH-mediated secretion of EGF ligands activates EGF receptor signaling in mural and cumulus granulosa cells and promotes meiotic maturation, possibly by effects on gap junctions (119). These pathways provide redundant mechanisms to ensure that resumption of meiosis occurs as the follicle prepares for ovulation.
THECA LAYER REMODELING/STIGMA FORMATION
Perhaps the most dramatic change during ovulation concerns the formation of a hole in the ovary surface, known as the macula pellucida or stigma, through which the egg and cumulus mass exit the follicle. Stigma formation involves a combination of cell apoptosis, cell migration, and proteolytic digestion of extracellular matrix layers (Fig. 22). LH is directly involved in stigma formation. In response to the LH surge, the preovulatory follicle produces progesterone and prostaglandin, both of which are obligatory for the stigma to develop in laboratory animals. The most compelling data to support this concept comes from gene "knockout" experiments showing ovulation defects in female mice lacking PR, COX-2, or PPAR (109, 120-122). Mice carrying a null mutation of the PR gene develop mature preovulatory follicles that undergo cumulus expansion in response to the gonadotropin surge; however, none ovulate because of the absence of stigma formation. Similarly, targeted disruption of COX-2 or PPAR produces anovulation and infertility in female mice because stigma formation is compromised. There is some evidence that COX-2-derived fatty acid metabolites serve as activating ligands for PPAR, which then induces expression of other ovulatory mediators including endothelin-2, interleukin-6, and cGMP-dependent protein kinase II (109). Additional signals related to theca cell remodeling are mediated by nerve growth factor (NGF) and one of its receptors, the tyrosine kinase receptor TrkA. NGF and TrkA expression are induced in theca cells in response to the LH surge (123). NGF/TrkA interactions lead to a loss of intercellular communication by disrupting gap junctions between theca cells and result in increased migratory behavior.
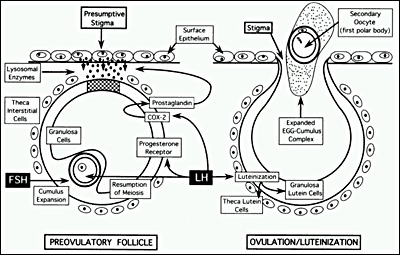
Figure 22
Diagram of the cellular mechanisms by which the preovulatory surge of FSH and LH causes ovulation. (Erickson GF: The Ovary: Basic Principles and Concepts: In Felig P, Baxter JD, Broadus AE, Froman LA, (eds): Endocrinology and Metabolism, 3rd edition. New York, McGraw Hill, 1995)
In addition to structural remodeling, the theca layer undergoes rapid alterations in the vascular supply in response to the ovulatory LH signal. Vascular endothelial growth factor (VEGF) and its downstream signaling pathways are required for follicle angiogenesis during antral follicle growth, and there is evidence in the primate that VEGF-mediated vascular remodeling is important for follicle rupture. VEGF promotes vascular permeability, allowing more efficient delivery of blood-borne factors including LH and FSH, and immune cells to the follicle. Indeed, elevated levels of VEGF, with consequent increases in vascular permeability, have been linked to iatrogenic ovarian hyperstimulation syndrome (124). There is also evidence for important functions of the angiopoietin-TEK receptor system in regulating vascular remodeling during the ovulation cascade (125). Immune cells, including macrophages and leukocytes, are delivered to the theca layer by the vasculature and release cytokines, proteases, and free radicals that promote additional remodeling of the follicle wall. These immune-mediated and other signaling pathways converge to generate a cascade of proteolytic and remodeling events that lead to controlled degradation of the ovarian stromal matrix overlying the follicle and subsequent stigma formation and the release of the mature egg-cumulus complex (Fig. 22).
LUTEINIZATION
After ovulation, the follicle wall develops into the corpus luteum (Fig. 23). The corpus luteum is a large endocrine gland that produces large amounts of progesterone and estradiol during the first week of the luteal phase of the cycle. There is a fibrin clot where the antrum and liquor folliculi were located, into which loose connective tissue and blood cells invade. Cells that make up the corpus luteum are contributed by the membrana granulosa, theca interna, theca externa, and invading blood tissue (Fig. 24).
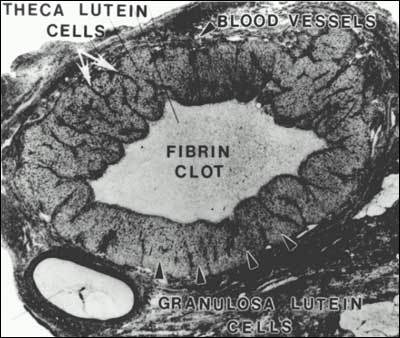
Figure 23
Photomicrograph of a human corpus luteum. (Bloom W, Fawcett DW (eds) in A Textbook of Histology. WB Saunders Co., Philadelphia 1975 with permission from Arnold)
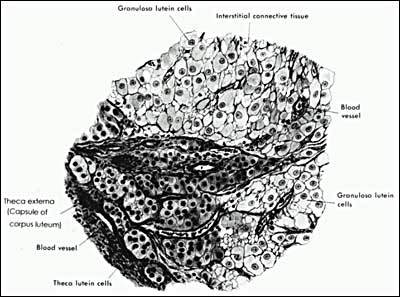
Figure 24
Drawing illustrating the histology of the human corpus luteum. (Bloom W, Fawcett DW (eds.) In A Textbook of Histology. WB Saunders Co., Philadelphia 1975)
After ovulation, the granulosa cells attain a large size, approximately 35 µm in diameter. These cells, now called granulosa-lutein cells, have an ultrastructure typical of differentiated steroidogenic cells; they contain abundant smooth endoplasmic reticulum, tubular cristae in the mitochondria, and large clusters of lipid droplets containing cholesterol esters in the cytoplasm (126). Immediately preceeding and following ovulation, the granulosa-lutein cells express large amounts of StAR, P450SCC, 3-HSD, and P450AROM. Accordingly they have a high capacity to produce progesterone and estradiol. During the process of luteinization, LH is required for maintaining steroidogenesis by the granulosa-lutein cells. There is also evidence that lipoproteins have potent luteotrophic effects on progesterone production (127).
The theca-interstitial cells also are incorporated into the corpus luteum, becoming the theca-lutein cells (Fig. 24). They can be distinguished from granulosa-lutein cells because they are smaller (approximately 15 µm in diameter) and stain more darkly. Theca-lutein cells also exhibit the ultrastructure of active steroid-secreting cells. They strongly express the enzymes in the androgen biosynthetic pathway and produce androstenedione. By virtue of this tissue specific pattern of expression of P450c17 and P450AROM it can be concluded that the two-cell-two-gonadotropin mechanism for estradiol synthesis operates in the human corpus luteum.
If implantation does not occur, the corpus luteum degenerates by a process called luteolysis. The death of the human corpus luteum becomes apparent histologically 8 days after ovulation. The first sign is the shrinkage of the granulosa-lutein cells. By contrast, the theca-lutein cells appear selectively hyperstimulated during early luteolysis. After day 23 of the menstrual cycle, apoptotic cells are present in the corpus luteum. Eventually luteolysis destroys all the cells in the corpus luteum. Histologically, all that remains is a nodule of dense connective tissue called the corpus albicans. Two questions, differing in kind, arise. One concerns the nature of the luteolytic process responsible for the cessation of steroidogenesis and the activation of the apoptotic or cell death pathways. The other concerns the nature of the hCG luteotropic process responsible for the rescue of the corpus luteum of the cycle into the corpus luteum of pregnancy. Apart from some very limited data, the answers to these fundamental questions remain a mystery in women.
CONCLUDING REMARKS
The central conclusion of this extensive literature base is that FSH and LH play a crucial role in controlling folliculogenesis, ovulation, and luteinization in female mammals, including women. In addition to the gonadotropins, the physiological roles for growth factors in regulating FSH- and LH-dependent signaling, observed experimentally mainly in rodents and other domestic animals, suggest potential clinical relevance in fertility and infertility in women. At present, we have precious little knowledge about the physiological role of growth factor-dependent mechanisms operating during the normal menstrual cycle. We can hope that finding the links between growth factor-mediated events in human ovary physiology and pathophysiology could help in the design novel therapeutic strategies for improving women’s health.
ACKNOWLEDGEMENTS
The authors thank Audrey Williams for creating original artwork. This work was supported by the Intramural Research Program of the National Institutes of Health, National Institutes of Environmental Health Sciences, Z01-ES1 02985.
References
1. Gougeon A 1996 Regulation of ovarian follicular development in primates: facts and hypotheses. Endocr Rev 17:121-155
2. Reynolds SRM 1950 The vasculature of the ovary and ovarian function. Recent Prog Horm Res 5:65
3. Soyal SM, Amleh A, Dean J 2000 FIGalpha, a germ cell-specific transcription factor required for ovarian follicle formation. Development 127:4645-4654
4. Uda M, Ottolenghi C, Crisponi L, Garcia JE, Deiana M, Kimber W, Forabosco A, Cao A, Schlessinger D, Pilia G 2004 Foxl2 disruption causes mouse ovarian failure by pervasive blockage of follicle development. Hum Mol Genet 13:1171-1181
5. Harris SE, Chand AL, Winship IM, Gersak K, Aittomaki K, Shelling AN 2002 Identification of novel mutations in FOXL2 associated with premature ovarian failure. Mol Hum Reprod 8:729-733
6. Udar N, Yellore V, Chalukya M, Yelchits S, Silva-Garcia R, Small K 2003 Comparative analysis of the FOXL2 gene and characterization of mutations in BPES patients. Hum Mutat 22:222-228
7. Zhao H, Chen ZJ, Qin Y, Shi Y, Wang S, Choi Y, Simpson JL, Rajkovic A 2008 Transcription factor FIGLA is mutated in patients with premature ovarian failure. Am J Hum Genet 82:1342-1348
8. Johnson J, Canning J, Kaneko T, Pru JK, Tilly JL 2004 Germline stem cells and follicular renewal in the postnatal mammalian ovary. Nature 428:145-150
9. Telfer EE, Gosden RG, Byskov AG, Spears N, Albertini D, Andersen CY, Anderson R, Braw-Tal R, Clarke H, Gougeon A, McLaughlin E, McLaren A, McNatty K, Schatten G, Silber S, Tsafriri A 2005 On regenerating the ovary and generating controversy. Cell 122:821-822
10. Kerr JB, Duckett R, Myers M, Britt KL, Mladenovska T, Findlay JK 2006 Quantification of healthy follicles in the neonatal and adult mouse ovary: evidence for maintenance of primordial follicle supply. Reproduction 132:95-109
11. Hansen KR, Knowlton NS, Thyer AC, Charleston JS, Soules MR, Klein NA 2008 A new model of reproductive aging: the decline in ovarian non-growing follicle number from birth to menopause. Hum Reprod 23:699-708
12. Schwartz D, Mayaux MJ 1982 Female fecundity as a function of age: results of artificial insemination in 2193 nulliparous women with azoospermic husbands. Federation CECOS. N Engl J Med 306:404-406
13. Eppig JJ, Wigglesworth K, Pendola FL 2002 The mammalian oocyte orchestrates the rate of ovarian follicular development. Proc Natl Acad Sci U S A 99:2890-2894
14. Sullivan SD, Castrillon DH 2011 Insights into primary ovarian insufficiency through genetically engineered mouse models. Semin Reprod Med 29:283-298
15. Reddy P, Liu L, Adhikari D, Jagarlamudi K, Rajareddy S, Shen Y, Du C, Tang W, Hamalainen T, Peng SL, Lan ZJ, Cooney AJ, Huhtaniemi I, Liu K 2008 Oocyte-specific deletion of Pten causes premature activation of the primordial follicle pool. Science 319:611-613
16. Castrillon DH, Miao L, Kollipara R, Horner JW, DePinho RA 2003 Suppression of ovarian follicle activation in mice by the transcription factor Foxo3a. Science 301:215-218
17. Adhikari D, Flohr G, Gorre N, Shen Y, Yang H, Lundin E, Lan Z, Gambello MJ, Liu K 2009 Disruption of Tsc2 in oocytes leads to overactivation of the entire pool of primordial follicles. Mol Hum Reprod 15:765-770
18. Holt JE, Jackson A, Roman SD, Aitken RJ, Koopman P, McLaughlin EA 2006 CXCR4/SDF1 interaction inhibits the primordial to primary follicle transition in the neonatal mouse ovary. Dev Biol 293:449-460
19. Choi YH, Toyoda Y 1998 Cyclodextrin removes cholesterol from mouse sperm and induces capacitation in a protein-free medium. Biol Reprod 59:1328-1333
20. Pangas SA, Choi Y, Ballow DJ, Zhao Y, Westphal H, Matzuk MM, Rajkovic A 2006 Oogenesis requires germ cell-specific transcriptional regulators Sohlh1 and Lhx8. Proc Natl Acad Sci U S A 103:8090-8095
21. Toyoda S, Miyazaki T, Miyazaki S, Yoshimura T, Yamamoto M, Tashiro F, Yamato E, Miyazaki J 2009 Sohlh2 affects differentiation of KIT positive oocytes and spermatogonia. Dev Biol 325:238-248
22. Parrott JA, Skinner MK 1999 Kit-ligand/stem cell factor induces primordial follicle development and initiates folliculogenesis. Endocrinology 140:4262-4271
23. Yoshida H, Takakura N, Kataoka H, Kunisada T, Okamura H, Nishikawa SI 1997 Stepwise requirement of c-kit tyrosine kinase in mouse ovarian follicle development. Dev Biol 184:122-137
24. Reddy P, Shen L, Ren C, Boman K, Lundin E, Ottander U, Lindgren P, Liu YX, Sun QY, Liu K 2005 Activation of Akt (PKB) and suppression of FKHRL1 in mouse and rat oocytes by stem cell factor during follicular activation and development. Dev Biol 281:160-170
25. Liu K, Rajareddy S, Liu L, Jagarlamudi K, Boman K, Selstam G, Reddy P 2006 Control of mammalian oocyte growth and early follicular development by the oocyte PI3 kinase pathway: new roles for an old timer. Dev Biol 299:1-11
26. Kezele P, Nilsson EE, Skinner MK 2005 Keratinocyte growth factor acts as a mesenchymal factor that promotes ovarian primordial to primary follicle transition. Biol Reprod 73:967-973
27. Nilsson EE, Skinner MK 2003 Bone morphogenetic protein-4 acts as an ovarian follicle survival factor and promotes primordial follicle development. Biol Reprod 69:1265-1272
28. Lee KF, Chow JF, Xu JS, Chan ST, Ip SM, Yeung WS 2001 A comparative study of gene expression in murine embryos developed in vivo, cultured in vitro, and cocultured with human oviductal cells using messenger ribonucleic acid differential display. Biol Reprod 64:910-917
29. Durlinger AL, Kramer P, Karels B, de Jong FH, Uilenbroek JT, Grootegoed JA, Themmen AP 1999 Control of primordial follicle recruitment by anti-Mullerian hormone in the mouse ovary. Endocrinology 140:5789-5796
30. Carlsson IB, Scott JE, Visser JA, Ritvos O, Themmen AP, Hovatta O 2006 Anti-Mullerian hormone inhibits initiation of growth of human primordial ovarian follicles in vitro. Hum Reprod 21:2223-2227
31. Kezele PR, Nilsson EE, Skinner MK 2002 Insulin but not insulin-like growth factor-1 promotes the primordial to primary follicle transition. Mol Cell Endocrinol 192:37-43
32. Gulyas BJ, Hodgen GD, Tullner WW, Ross GT 1977 Effects of fetal or maternal hypophysectomy on endocrine organs and body weight in infant rhesus monkeys (Macaca mulatta): with particular emphasis on oogenesis. Biol Reprod 16:216-227
33. Oktay K, Newton H, Mullan J, Gosden RG 1998 Development of human primordial follicles to antral stages in SCID/hpg mice stimulated with follicle stimulating hormone. Hum Reprod 13:1133-1138
34. Oktay K, Briggs D, Gosden RG 1997 Ontogeny of follicle-stimulating hormone receptor gene expression in isolated human ovarian follicles. J Clin Endocrinol Metab 82:3748-3751
35. Findlay JK, Drummond AE 1999 Regulation of the FSH Receptor in the Ovary. Trends Endocrinol Metab 10:183-188
36. Fortune JE, Cushman RA, Wahl CM, Kito S 2000 The primordial to primary follicle transition. Mol Cell Endocrinol 163:53-60
37. Bachvarova R, Cohen EM, De Leon V, Tokunaga K, Sakiyama S, Paynton BV 1989 Amounts and modulation of actin mRNAs in mouse oocytes and embryos. Development 106:561-565
38. Choi Y, Rajkovic A 2006 Characterization of NOBOX DNA binding specificity and its regulation of Gdf9 and Pou5f1 promoters. J Biol Chem 281:35747-35756
39. Joshi S, Davies H, Sims LP, Levy SE, Dean J 2007 Ovarian gene expression in the absence of FIGLA, an oocyte-specific transcription factor. BMC Dev Biol 7:67
40. Shimasaki S, Moore RK, Otsuka F, Erickson GF 2004 The bone morphogenetic protein system in mammalian reproduction. Endocr Rev 25:72-101
41. Vanderhyden B 2002 Molecular basis of ovarian development and function. Front Biosci 7:d2006-2022
42. Gilchrist RB, Lane M, Thompson JG 2008 Oocyte-secreted factors: regulators of cumulus cell function and oocyte quality. Hum Reprod Update 14:159-177
43. Teixeira Filho FL, Baracat EC, Lee TH, Suh CS, Matsui M, Chang RJ, Shimasaki S, Erickson GF 2002 Aberrant expression of growth differentiation factor-9 in oocytes of women with polycystic ovary syndrome. J Clin Endocrinol Metab 87:1337-1344
44. Hreinsson JG, Scott JE, Rasmussen C, Swahn ML, Hsueh AJ, Hovatta O 2002 Growth differentiation factor-9 promotes the growth, development, and survival of human ovarian follicles in organ culture. J Clin Endocrinol Metab 87:316-321
45. Di Pasquale E, Beck-Peccoz P, Persani L 2004 Hypergonadotropic ovarian failure associated with an inherited mutation of human bone morphogenetic protein-15 (BMP15) gene. Am J Hum Genet 75:106-111
46. Packer AI, Hsu YC, Besmer P, Bachvarova RF 1994 The ligand of the c-kit receptor promotes oocyte growth. Dev Biol 161:194-205
47. Eppig JJ 1994 Oocyte-somatic cell communication in the ovarian follicles of mammals. Sem Devel Biol 5:51-59
48. Albertini DF, Combelles CM, Benecchi E, Carabatsos MJ 2001 Cellular basis for paracrine regulation of ovarian follicle development. Reproduction 121:647-653
49. Makabe S, Naguro T, Stallone T 2006 Oocyte-follicle cell interactions during ovarian follicle development, as seen by high resolution scanning and transmission electron microscopy in humans. Microsc Res Tech 69:436-449
50. Bruzzone R, White TW, Paul DL 1996 Connections with connexins: the molecular basis of direct intercellular signaling. Eur J Biochem 238:1-27
51. Simon AM, Goodenough DA, Li E, Paul DL 1997 Female infertility in mice lacking connexin 37. Nature 385:525-529
52. Grazul-Bilska AT, Reynolds LP, Redmer DA 1997 Gap junctions in the ovaries. Biol Reprod 57:947-957
53. Carabatsos MJ, Sellitto C, Goodenough DA, Albertini DF 2000 Oocyte-granulosa cell heterologous gap junctions are required for the coordination of nuclear and cytoplasmic meiotic competence. Dev Biol 226:167-179.
54. Chang H, Brown CW, Matzuk MM 2002 Genetic analysis of the mammalian transforming growth factor-beta superfamily. Endocr Rev 23:787-823
55. Juneja SC, Barr KJ, Enders GC, Kidder GM 1999 Defects in the germ line and gonads of mice lacking connexin43. Biol Reprod 60:1263-1270
56. Erickson GF, Magoffin DA, Dyer CA, Hofeditz C 1985 The ovarian androgen producing cells: a review of structure/function relationships. Endocr Rev 6:371-399
57. Erickson GF, Shimasaki S 2003 The spatiotemporal expression pattern of the bone morphogenetic protein family in rat ovary cell types during the estrous cycle. Reprod Biol Endocrinol 1:9
58. Pincus G, Enzmann EV 1935 The comparative behavior of mammalian eggs in vivo and in vitro: I. The activation of ovarian eggs. J Exp Med 62:665-675
59. Conti M, Andersen CB, Richard F, Mehats C, Chun SY, Horner K, Jin C, Tsafriri A 2002 Role of cyclic nucleotide signaling in oocyte maturation. Mol Cell Endocrinol 187:153-159
60. Mehlmann LM 2005 Oocyte-specific expression of Gpr3 is required for the maintenance of meiotic arrest in mouse oocytes. Dev Biol 288:397-404
61. DiLuigi A, Weitzman VN, Pace MC, Siano LJ, Maier D, Mehlmann LM 2008 Meiotic arrest in human oocytes is maintained by a Gs signaling pathway. Biol Reprod 78:667-672
62. Vaccari S, Horner K, Mehlmann LM, Conti M 2008 Generation of mouse oocytes defective in cAMP synthesis and degradation: endogenous cyclic AMP is essential for meiotic arrest. Dev Biol 316:124-134
63. Norris RP, Ratzan WJ, Freudzon M, Mehlmann LM, Krall J, Movsesian MA, Wang H, Ke H, Nikolaev VO, Jaffe LA 2009 Cyclic GMP from the surrounding somatic cells regulates cyclic AMP and meiosis in the mouse oocyte. Development 136:1869-1878
64. Zhang M, Su YQ, Sugiura K, Xia G, Eppig JJ 2010 Granulosa cell ligand NPPC and its receptor NPR2 maintain meiotic arrest in mouse oocytes. Science 330:366-369
65. Edwards RG 1974 Follicular fluid. J Reprod Fertil 37:189-219
66. Erickson GF, Shimasaki S 2000 The role of the oocyte in folliculogenesis. Trends Endocrinol Metab 11:193-198
67. Hendriks DJ, Mol BW, Bancsi LF, Te Velde ER, Broekmans FJ 2005 Antral follicle count in the prediction of poor ovarian response and pregnancy after in vitro fertilization: a meta-analysis and comparison with basal follicle-stimulating hormone level. Fertil Steril 83:291-301
68. Erickson GF 1993 Normal regulation of ovarian androgen production. Semin Reprod Endocrinol 11:307-312
69. Eppig JJ 2001 Oocyte control of ovarian follicular development and function in mammals. Reproduction 122:829-838
70. Danial NN, Korsmeyer SJ 2004 Cell death: critical control points. Cell 116:205-219
71. Hussein MR 2005 Apoptosis in the ovary: molecular mechanisms. Hum Reprod Update 11:162-177
72. Craig J, Orisaka M, Wang H, Orisaka S, Thompson W, Zhu C, Kotsuji F, Tsang BK 2007 Gonadotropin and intra-ovarian signals regulating follicle development and atresia: the delicate balance between life and death. Front Biosci 12:3628-3639
73. Orisaka M, Orisaka S, Jiang JY, Craig J, Wang Y, Kotsuji F, Tsang BK 2006 Growth differentiation factor 9 is antiapoptotic during follicular development from preantral to early antral stage. Mol Endocrinol 20:2456-2468
74. Robles R, Tao XJ, Trbovich AM, Maravel DV, Nahum R, Perez GI, Tilly KI, Tilly JL 1999 Localization, regulation and possible consequences of apoptotic protease-activating factor-1 (Apaf-1) expression in granulosa cells of the mouse ovary. Endocrinology 140:2641-2644
75. Wang H, Jiang JY, Zhu C, Peng C, Tsang BK 2006 Role and regulation of nodal/activin receptor-like kinase 7 signaling pathway in the control of ovarian follicular atresia. Mol Endocrinol 20:2469-2482
76. Kim JM, Yoon YD, Tsang BK 1999 Involvement of the Fas/Fas ligand system in p53-mediated granulosa cell apoptosis during follicular development and atresia. Endocrinology 140:2307-2317
77. Thompson WE, Asselin E, Branch A, Stiles JK, Sutovsky P, Lai L, Im GS, Prather RS, Isom SC, Rucker E, 3rd, Tsang BK 2004 Regulation of prohibitin expression during follicular development and atresia in the mammalian ovary. Biol Reprod 71:282-290
78. Chowdhury I, Xu W, Stiles JK, Zeleznik A, Yao X, Matthews R, Thomas K, Thompson WE 2007 Apoptosis of rat granulosa cells after staurosporine and serum withdrawal is suppressed by adenovirus-directed overexpression of prohibitin. Endocrinology 148:206-217
79. Simoni M, Gromoll J, Nieschlag E 1997 The follicle-stimulating hormone receptor: biochemistry, molecular biology, physiology, and pathophysiology. Endocr Rev 18:739-773
80. Menon KM, Clouser CL, Nair AK 2005 Gonadotropin receptors: role of post-translational modifications and post-transcriptional regulation. Endocrine 26:249-257
81. Gurevich VV, Gurevich EV 2008 How and why do GPCRs dimerize? Trends Pharmacol Sci 29:234-240
82. Urizar E, Montanelli L, Loy T, Bonomi M, Swillens S, Gales C, Bouvier M, Smits G, Vassart G, Costagliola S 2005 Glycoprotein hormone receptors: link between receptor homodimerization and negative cooperativity. Embo J 24:1954-1964
83. Costagliola S, Urizar E, Mendive F, Vassart G 2005 Specificity and promiscuity of gonadotropin receptors. Reproduction 130:275-281
84. Daelemans C, Smits G, de Maertelaer V, Costagliola S, Englert Y, Vassart G, Delbaere A 2004 Prediction of severity of symptoms in iatrogenic ovarian hyperstimulation syndrome by follicle-stimulating hormone receptor Ser680Asn polymorphism. J Clin Endocrinol Metab 89:6310-6315
85. Gonzalez-Robayna IJ, Falender AE, Ochsner S, Firestone GL, Richards JS 2000 Follicle-Stimulating hormone (FSH) stimulates phosphorylation and activation of protein kinase B (PKB/Akt) and serum and glucocorticoid-lnduced kinase (Sgk): evidence for A kinase-independent signaling by FSH in granulosa cells. Mol Endocrinol 14:1283-1300
86. Wayne CM, Fan HY, Cheng X, Richards JS 2007 Follicle-stimulating hormone induces multiple signaling cascades: evidence that activation of Rous sarcoma oncogene, RAS, and the epidermal growth factor receptor are critical for granulosa cell differentiation. Mol Endocrinol 21:1940-1957
87. Hunzicker-Dunn M, Maizels ET 2006 FSH signaling pathways in immature granulosa cells that regulate target gene expression: branching out from protein kinase A. Cell Signal 18:1351-1359
88. Gougeon A, Testart J 1990 Influence of human menopausal gonadotropin on the recruitment of human ovarian follicles. Fertil Steril 54:848-852
89. Hillier SG 2001 Gonadotropic control of ovarian follicular growth and development. Mol Cell Endocrinol 179:39-46
90. Knight PG, Glister C 2006 TGF-beta superfamily members and ovarian follicle development. Reproduction 132:191-206
91. Simpson ER 2004 Aromatase: biologic relevance of tissue-specific expression. Semin Reprod Med 22:11-23
92. Inkster SE, Brodie AM 1991 Expression of aromatase cytochrome P-450 in premenopausal and postmenopausal human ovaries: an immunocytochemical study. J Clin Endocrinol Metab 73:717-726
93. Suzuki T, Sasano H, Tamura M, Aoki H, Fukaya T, Yajima A, Nagura H, Mason JI 1993 Temporal and spatial localization of steroidogenic enzymes in premenopausal human ovaries: in situ hybridization and immunohistochemical study. Mol Cell Endocrinol 97:135-143
94. Doody KJ, Lorence MC, Mason JI, Simpson ER 1990 Expression of messenger ribonucleic acid species encoding steroidogenic enzymes in human follicles and corpora lutea throughout the menstrual cycle. J Clin Endocrinol Metab 70:1041-1045
95. Ghersevich SA, Poutanen MH, Martikainen HK, Vihko RK 1994 Expression of 17 beta-hydroxysteroid dehydrogenase in human granulosa cells: correlation with follicular size, cytochrome P450 aromatase activity and oestradiol production. J Endocrinol 143:139-150
96. Sawetawan C, Milewich L, Word RA, Carr BR, Rainey WE 1994 Compartmentalization of type I 17 beta-hydroxysteroid oxidoreductase in the human ovary. Mol Cell Endocrinol 99:161-168
97. Zhang Y, Word RA, Fesmire S, Carr BR, Rainey WE 1996 Human ovarian expression of 17 beta-hydroxysteroid dehydrogenase types 1, 2, and 3. J Clin Endocrinol Metab 81:3594-3598
98. Shimasaki S, Zachow RJ, Li D, Kim H, Iemura S, Ueno N, Sampath K, Chang RJ, Erickson GF 1999 A functional bone morphogenetic protein system in the ovary. Proc Natl Acad Sci U S A 96:7282-7287
99. Ascoli M, Fanelli F, Segaloff DL 2002 The lutropin/choriogonadotropin receptor, a 2002 perspective. Endocr Rev 23:141-174
100. McNatty KP, Baird DT, Bolton A, Chambers P, Corker CS, McLean H 1976 Concentration of oestrogens and androgens in human ovarian venous plasma and follicular fluid throughout the menstrual cycle. J Endocrinol 71:77-85
101. Dooley CA, Attia GR, Rainey WE, Moore DR, Carr BR 2000 Bone morphogenetic protein inhibits ovarian androgen production. J Clin Endocrinol Metab 85:3331-3337
102. Fainstat T 1968 Organ culture of postnatal rat ovaries in chemically defined medium. Fertil Steril 19:317-338
103. Hillier SG, Yong EL, Illingworth PJ, Baird DT, Schwall RH, Mason AJ 1991 Effect of recombinant activin on androgen synthesis in cultured human thecal cells. J Clin Endocrinol Metab 72:1206-1211
104. Hillier SG, Yong EL, Illingworth PJ, Baird DT, Schwall RH, Mason AJ 1991 Effect of recombinant inhibin on androgen synthesis in cultured human thecal cells. Mol Cell Endocrinol 75:R1-6
105. Magoffin DA, Erickson GF 1988 An improved method for primary culture of ovarian androgen-producing cells in serum-free medium: effect of lipoproteins, insulin, and insulinlike growth factor-I. In Vitro Cell Dev Biol 24:862-870
106. Nahum R, Thong KJ, Hillier SG 1995 Metabolic regulation of androgen production by human thecal cells in vitro. Hum Reprod 10:75-81
107. Yamamoto N, Christenson LK, McAllister JM, Strauss JF, 3rd 2002 Growth differentiation factor-9 inhibits 3'5'-adenosine monophosphate-stimulated steroidogenesis in human granulosa and theca cells. J Clin Endocrinol Metab 87:2849-2856
108. Russell DL, Robker RL 2007 Molecular mechanisms of ovulation: co-ordination through the cumulus complex. Hum Reprod Update 13:289-312
109. Kim J, Sato M, Li Q, Lydon JP, Demayo FJ, Bagchi IC, Bagchi MK 2008 Peroxisome proliferator-activated receptor gamma is a target of progesterone regulation in the preovulatory follicles and controls ovulation in mice. Mol Cell Biol 28:1770-1782
110. Unluturk U, Harmanci A, Kocaefe C, Yildiz BO 2007 The Genetic Basis of the Polycystic Ovary Syndrome: A Literature Review Including Discussion of PPAR-gamma. PPAR Res 2007:49109
111. Russell DL, Doyle KM, Ochsner SA, Sandy JD, Richards JS 2003 Processing and localization of ADAMTS-1 and proteolytic cleavage of versican during cumulus matrix expansion and ovulation. J Biol Chem 278:42330-42339
112. Conti M, Hsieh M, Park JY, Su YQ 2006 Role of the epidermal growth factor network in ovarian follicles. Mol Endocrinol 20:715-723
113. Ben-Ami I, Freimann S, Armon L, Dantes A, Strassburger D, Friedler S, Raziel A, Seger R, Ron-El R, Amsterdam A 2006 PGE2 up-regulates EGF-like growth factor biosynthesis in human granulosa cells: new insights into the coordination between PGE2 and LH in ovulation. Mol Hum Reprod 12:593-599
114. Athanasiou S, Bourne TH, Khalid A, Okokon EV, Crayford TJ, Hagstrom HG, Campbell S, Collins WP 1996 Effects of indomethacin on follicular structure, vascularity, and function over the periovulatory period in women. Fertil Steril 65:556-560
115. Chung NP, Mruk D, Mo MY, Lee WM, Cheng CY 2001 A 22-amino acid synthetic peptide corresponding to the second extracellular loop of rat occludin perturbs the blood-testis barrier and disrupts spermatogenesis reversibly in vivo. Biol Reprod 65:1340-1351
116. Varani S, Elvin JA, Yan C, DeMayo J, DeMayo FJ, Horton HF, Byrne MC, Matzuk MM 2002 Knockout of pentraxin 3, a downstream target of growth differentiation factor-9, causes female subfertility. Mol Endocrinol 16:1154-1167
117. Mehlmann LM, Jones TL, Jaffe LA 2002 Meiotic arrest in the mouse follicle maintained by a Gs protein in the oocyte. Science 297:1343-1345
118. Norris RP, Freudzon M, Mehlmann LM, Cowan AE, Simon AM, Paul DL, Lampe PD, Jaffe LA 2008 Luteinizing hormone causes MAP kinase-dependent phosphorylation and closure of connexin 43 gap junctions in mouse ovarian follicles: one of two paths to meiotic resumption. Development 135:3229-3238
119. Conti M, Hsieh M, Musa Zamah A, Oh JS 2011 Novel signaling mechanisms in the ovary during oocyte maturation and ovulation. Mol Cell Endocrinol
120. Chappell PE, Lydon JP, Conneely OM, O'Malley BW, Levine JE 1997 Endocrine defects in mice carrying a null mutation for the progesterone receptor gene. Endocrinology 138:4147-4152
121. Lydon JP, DeMayo FJ, Funk CR, Mani SK, Hughes AR, Montgomery CA, Jr., Shyamala G, Conneely OM, O'Malley BW 1995 Mice lacking progesterone receptor exhibit pleiotropic reproductive abnormalities. Genes Dev 9:2266-2278
122. Lim H, Paria BC, Das SK, Dinchuk JE, Langenbach R, Trzaskos JM, Dey SK 1997 Multiple female reproductive failures in cyclooxygenase 2-deficient mice. Cell 91:197-208
123. Dissen GA, Hill DF, Costa ME, Les Dees CW, Lara HE, Ojeda SR 1996 A role for trkA nerve growth factor receptors in mammalian ovulation. Endocrinology 137:198-209
124. Levin ER, Rosen GF, Cassidenti DL, Yee B, Meldrum D, Wisot A, Pedram A 1998 Role of vascular endothelial cell growth factor in Ovarian Hyperstimulation Syndrome. J Clin Invest 102:1978-1985
125. Xu F, Stouffer RL 2005 Local delivery of angiopoietin-2 into the preovulatory follicle terminates the menstrual cycle in rhesus monkeys. Biol Reprod 72:1352-1358
126. Crisp TM, Dessouky DA, Denys FR 1970 The fine structure of the human corpus luteum of early pregnancy and during the progestational phase of the mestrual cycle. Am J Anat 127:37-69
127. Carr BR, MacDonald PC, Simpson ER 1982 The role of lipoproteins in the regulation of progesterone secretion by the human corpus luteum. Fertil Steril 38:303-311
Publication Details
Author Information and Affiliations
Publication History
Last Update: January 30, 2012.
Copyright
This electronic version has been made freely available under a Creative Commons (CC-BY-NC-ND) license. A copy of the license can be viewed at http://creativecommons.org/licenses/by-nc-nd/2.0/.
Publisher
MDText.com, Inc., South Dartmouth (MA)
NLM Citation
Williams CJ, Erickson GF. Morphology and Physiology of the Ovary. [Updated 2012 Jan 30]. In: Feingold KR, Anawalt B, Blackman MR, et al., editors. Endotext [Internet]. South Dartmouth (MA): MDText.com, Inc.; 2000-.