By agreement with the publisher, this book is accessible by the search feature, but cannot be browsed.
NCBI Bookshelf. A service of the National Library of Medicine, National Institutes of Health.
Janeway CA Jr, Travers P, Walport M, et al. Immunobiology: The Immune System in Health and Disease. 5th edition. New York: Garland Science; 2001.
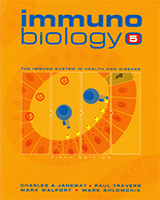
Immunobiology: The Immune System in Health and Disease. 5th edition.
Show detailsIn contrast to the immunoglobulins, which interact with pathogens and their toxic products in the extracellular spaces of the body, T cells only recognize foreign antigens that are displayed on the surfaces of the body's own cells. These antigens can derive from pathogens such as viruses or intracellular bacteria, which replicate within cells, or from pathogens or their products that cells have internalized by endocytosis from the extracellular fluid.
T cells can detect the presence of an intracellular pathogen because infected cells display on their surface peptide fragments derived from the pathogen's proteins. These foreign peptides are delivered to the cell surface by specialized host-cell glycoproteins. These are encoded in a large cluster of genes that were first identified by their powerful effects on the immune response to transplanted tissues. For that reason, the gene complex was called the major histocompatibility complex (MHC), and the peptide-binding glycoproteins are still known as MHC molecules. The recognition of antigen as a small peptide fragment bound to an MHC molecule and displayed at the cell surface is one of the most distinctive features of T cells, and will be the focus of this part of the chapter. How peptide fragments of antigen become complexed with MHC molecules will be considered in Chapter 5.
In this part of the chapter we will describe the structure and properties of the T-cell antigen receptor, T-cell receptor, or TCR for short. As might be expected from their function as highly variable antigen-recognition structures, T-cell receptors are closely related to antibody molecules in the structure of their genes. There are, however, important differences between T-cell receptors and immunoglobulins that reflect the special features of antigen recognition by the T-cell receptor, and its lack of effector functions.
3-10. The antigen receptor on T cells is very similar to a Fab fragment of immunoglobulin
T-cell receptors were first identified using monoclonal antibodies that bound only one cloned T-cell line but not others and that could specifically inhibit antigen recognition by that clone of T cells, or specifically activate them (see Appendix I, Section A-19). These clonotypic antibodies were then used to show that each T cell bears about 30,000 antigen-receptor molecules on its surface, each receptor consisting of two different polypeptide chains, termed the T-cell receptor α (TCRα) and β (TCRβ) chains, linked by a disulfide bond. These α:β heterodimers are very similar in structure to the Fab fragment of an immunoglobulin molecule (Fig. 3.11), and they account for antigen recognition by most T cells. A minority of T cells bear an alternative, but structurally similar, receptor made up of a different pair of polypeptide chains designated γ and δ. γ:δ T-cell receptors appear to have different antigen-recognition properties from the α:β T-cell receptors, and the function of γ:δ T cells in immune responses is not yet entirely clear. In the rest of this chapter, we shall use the term T-cell receptor to mean the α:β receptor, except where specified otherwise. Both types of T-cell receptor differ from the membrane-bound immunoglobulin that serves as the B-cell receptor: a T-cell receptor has only one antigen-binding site, whereas a B-cell receptor has two, and T-cell receptors are never secreted, whereas immunoglobulin can be secreted as antibody.

Figure 3.11
The T-cell receptor resembles a membrane-bound Fab fragment. The Fab fragment of antibody molecules is a disulfide-linked heterodimer, each chain of which contains one immunoglobulin C domain and one V domain; the juxtaposition of the V domains forms the (more...)
Our initial insights into the structure and function of the α:β T-cell receptor came from studies of cloned cDNA encoding the receptor chains. The amino acid sequences predicted from T-cell receptor cDNAs show clearly that both chains of the T-cell receptor have an amino-terminal variable (V) region with homology to an immunoglobulin V domain, a constant (C) region with homology to an immunoglobulin C domain, and a short hinge region containing a cysteine residue that forms the interchain disulfide bond (Fig. 3.12). Each chain spans the lipid bilayer by a hydrophobic transmembrane domain, and ends in a short cytoplasmic tail. These close similarities of T-cell receptor chains to the heavy and light immunoglobulin chains first enabled prediction of the structural resemblance of the T-cell receptor heterodimer to a Fab fragment of immunoglobulin.

Figure 3.12
Structure of the T-cell receptor. The T-cell receptor heterodimer is composed of two trans-membrane glycoprotein chains, α and β. The extracellular portion of each chain consists of two domains, resembling immunoglobulin V and C domains, (more...)
Recently, the three-dimensional structure of the T-cell receptor has been determined. The structure is indeed similar to that of an antibody Fab fragment, as was suspected from earlier studies on the genes that encoded it. The T-cell receptor chains fold in much the same way as those of a Fab fragment (Fig. 3.13a), although the final structure appears a little shorter and wider. There are, however, some distinct differences between T-cell receptors and Fab fragments. The most striking difference is in the Cα domain, where the fold is unlike that of any other immunoglobulin-like domain. The half of the domain that is juxtaposed with the Cβ domain forms a β sheet similar to that found in other immunoglobulin-like domains, but the other half of the domain is formed of loosely packed strands and a short segment of α helix (Fig. 3.13b). The intramolecular disulfide bond, which in immunoglobulin-like domains normally joins two β strands, in a Cα domain joins a β strand to this segment of α helix.

Figure 3.13
The crystal structure of an α:β T-cell receptor resolved at 2.5 Å. In panels a and b the α chain is shown in pink and the β chain in blue. Disulfide bonds are shown in green. In panel a, the T-cell receptor is viewed (more...)
There are also differences in the way in which the domains interact. The interface between the V and C domains of both T-cell receptor chains is more extensive than in antibodies, which may make the hinge joint between the domains less flexible. And the interaction between the Cα and Cβ domains is distinctive in being assisted by carbohydrate, with a sugar group from the Cα domain making a number of hydrogen bonds to the Cβ domain (see Fig. 3.13b). Finally, a comparison of the variable binding sites shows that, although the complementarity-determining region (CDR) loops align fairly closely with those of antibody molecules, there is some displacement relative to those of the antibody molecule (Fig. 3.13c). This displacement is particularly marked in the Vα CDR2 loop, which is oriented at roughly right angles to the equivalent loop in antibody V domains, as a result of a shift in the β strand that anchors one end of the loop from one face of the domain to the other. A strand displacement also causes a change in the orientation of the Vβ CDR2 loop in two of the seven Vβ domains whose structures are known. As yet, the crystallographic structures of only seven T-cell receptors have been solved to this level of resolution, so it remains to be seen to what degree all T-cell receptors share these features, and whether there is more variability to be discovered.
3-11. A T-cell receptor recognizes antigen in the form of a complex of a foreign peptide bound to an MHC molecule
Antigen recognition by T-cell receptors clearly differs from recognition by B-cell receptors and antibodies. Antigen recognition by B cells involves direct binding of immunoglobulin to the intact antigen and, as discussed in Section 3-8, antibodies typically bind to the surface of protein antigens, contacting amino acids that are discontinuous in the primary structure but are brought together in the folded protein. T cells, on the other hand, were found to respond to short contiguous amino acid sequences in proteins. These sequences were often buried within the native structure of the protein and thus could not be recognized directly by T-cell receptors unless some unfolding of the protein antigen and its ‘processing’ into peptide fragments had occurred (Fig. 3.14).

Figure 3.14
Differences in the recognition of hen egg-white lysozyme by immunoglobulins and T-cell receptors. Antibodies can be shown by X-ray crystallography to bind epitopes on the surface of proteins, as shown in panel a, where the epitopes for three antibodies (more...)
The nature of the antigen recognized by T cells became clear with the realization that the peptides that stimulate T cells are recognized only when bound to an MHC molecule. These cell-surface glycoproteins are encoded by genes within the major histocompatibility complex (MHC). The ligand recognized by the T cell is thus a complex of peptide and MHC molecule. The evidence for involvement of the MHC in T-cell recognition of antigen was at first indirect, but it has recently been proved conclusively by stimulating T cells with purified peptide:MHC complexes. The T-cell receptor interacts with this ligand by making contacts with both the MHC molecule and the antigen peptide.
3-12. T cells with different functions are distinguished by CD4 and CD8 cell-surface proteins and recognize peptides bound to different classes of MHC molecule
T cells fall into two major classes that have different effector functions. The two classes are distinguished by the expression of the cell-surface proteins CD4 and CD8. These two types of T cell differ in the class of MHC molecule they recognize. There are two classes of MHC molecule—MHC class I and MHC class II—which differ in their structure and expression pattern on tissues of the body (see Section 3-13). CD4 and CD8 were known as markers for different functional sets of T cells for some time before it became clear that they play an important part in the direct recognition of MHC class II and MHC class I molecules, respectively. CD4 binds to the MHC class II molecule and CD8 to the MHC class I molecule. During antigen recognition, depending on the type of T-cell, CD4 or CD8 molecules associate on the T-cell surface with the T-cell receptor and bind to invariant sites on the MHC portion of the composite MHC:peptide ligand. This binding is required for the T cell to make an effective response, and so CD4 and CD8 are called co-receptors.
CD4 is a single-chain molecule composed of four immunoglobulin-like domains (Fig. 3.15). The first two domains (D1 and D2) of the CD4 molecule are packed tightly together to form a rigid rod some 60 Å long, which is joined by a flexible hinge to a similar rod formed by the third and fourth domains (D3 and D4). CD4 binds MHC class II molecules through a region that is located mainly on a lateral face of the first domain, D1. Because CD4 binds to a site on the β2 domain of the MHC class II molecule that is well away from the site where the T-cell receptor binds (Fig. 3.16a), the CD4 molecule and the T-cell receptor can bind the same peptide:MHC class II complex. CD4 interacts strongly with a cytoplasmic tyrosine kinase called Lck, and can deliver this tyrosine kinase into close proximity with the signaling components of the T-cell receptor complex. This results in enhancement of the signal that is generated when the T-cell receptor binds its peptide:MHC class II ligand, as we will discuss further in Chapter 6. When CD4 and the T-cell receptor can simultaneously bind to the same MHC class II:peptide complex, the sensitivity of a T cell to antigen presented by MHC class II molecules is markedly increased; the T-cell in this case requires 100-fold less antigen for activation.

Figure 3.15
The outline structures of the CD4 and CD8 co-receptor molecules. . The CD4 molecule contains four immunoglobulin-like domains, as shown in diagrammatic form in panel a, and as a ribbon diagram of the structure in panel b. The amino-terminal domain, D (more...)

Figure 3.16
The binding sites for CD4 and CD8 on MHC class II and class I molecules lie in the immunoglobulin-like domains. The binding sites for CD4 and CD8 on the MHC class II and class I molecules, respectively, lie in the immunoglobulin-like domains nearest to (more...)
CD4 binding to an MHC class II molecule on its own is weak, and it is not clear whether such binding would be able to transmit a signal to the interior of the T cell. As shown in Fig. 3.17, CD4 can form homodimers through a site in the D4 domain, which leaves the MHC-binding site free to interact with an MHC class II molecule. Thus, the CD4 dimer could cross-link two MHC class II molecules and thus the two T-cell receptors bound to them. Whether the dimerization of CD4 is important in its co-receptor function is not known at present.

Figure 3.17
CD4 is capable of forming dimers. The structure of the extra-cellular domains of the CD4 molecule has been determined by X-ray crystallography. Two molecules of CD4 can interact with each other through their D4 domains, forming homodimers. The site that (more...)
Although CD4 and CD8 both function as co-receptors, their structures are quite distinct. The CD8 molecule is a disulfide-linked heterodimer consisting of an α and a β chain, each containing a single immunoglobulin-like domain linked to the membrane by a segment of extended polypeptide chain (see Fig. 3.15). This segment is extensively glycosylated, which is thought to be important in maintaining this polypeptide in an extended conformation and protecting it from cleavage by proteases. CD8α chains can also form homo-dimers, although these are not found when the CD8β chains are present.
CD8 binds weakly to an invariant site in the α3 domain of an MHC class I molecule (Fig. 3.16b), which is equivalent to the site in MHC class II molecules to which CD4 binds. Although only the interaction of the CD8α homodimer with MHC class I is so far known in detail, it is clear from this that the MHC class I binding site of the CD8 α:β heterodimer will be formed by the interaction of the CD8α and β chains. In addition, CD8 (most probably through the α chain) interacts with residues in the base of the α2 domain of the MHC class I molecule. Binding in this way, CD8 leaves the upper surface of the MHC class I molecule exposed and free to interact simultaneously with a T-cell receptor, as shown in Fig. 3.18. Like CD4, CD8 also binds Lck through the cytoplasmic tail of the α chain and brings it into close proximity with the T-cell receptor. And as with CD4, the presence of CD8 increases the sensitivity of T cells to antigen presented by MHC class I molecules by about 100-fold. Thus, CD4 and CD8 have similar functions and bind to the same approximate location in MHC class I and MHC class II molecules even though the structures of the two co-receptor proteins are only distantly related.

Figure 3.18
CD8 binds to a site on MHC class I molecules distant from that to which the T-cell receptor binds. The relative positions of the T-cell receptor and CD8 molecules bound to the same MHC class I molecule can be seen in this hypothetical reconstruction of (more...)
3-13. The two classes of MHC molecule are expressed differentially on cells
MHC class I and MHC class II molecules have a distinct distribution among cells that reflects the different effector functions of the T cells that recognize them (Fig. 3.19). MHC class I molecules present peptides from pathogens, commonly viruses, to CD8 cytotoxic T cells, which are specialized to kill any cell that they specifically recognize. As viruses can infect any nucleated cell, almost all such cells express MHC class I molecules, although the level of constitutive expression varies from one cell type to the next. For example, cells of the immune system express abundant MHC class I on their surface, whereas liver cells (hepatocytes) express relatively low levels (see Fig. 3.19). Nonnucleated cells, such as mammalian red blood cells, express little or no MHC class I, and thus the interior of red blood cells is a site in which an infection can go undetected by cytotoxic T cells. As red blood cells cannot support viral replication, this is of no great consequence for viral infection, but it may be the absence of MHC class I that allows the Plasmodium species that cause malaria to live in this privileged site.

Figure 3.19
The expression of MHC molecules differs between tissues. MHC class I molecules are expressed on all nucleated cells, although they are most highly expressed in hematopoietic cells. MHC class II molecules are normally expressed only by a subset of hematopoietic cells (more...)
In contrast, the main function of the CD4 T cells that recognize MHC class II molecules is to activate other effector cells of the immune system. Thus MHC class II molecules are normally found on B lymphocytes, dendritic cells, and macrophages—cells that participate in immune responses—but not on other tissue cells (see Fig. 3.19). When CD4 T cells recognize peptides bound to MHC class II molecules on B cells, they stimulate the B cells to produce antibody Likewise, CD4 T cells recognizing peptides bound to MHC class II molecules on macrophages activate these cells to destroy the pathogens in their vesicles We shall see in Chapter 8 that MHC class II molecules are also expressed on specialized antigen-presenting cells in lymphoid tissues where naive T cells encounter antigen and are first activated. The expression of both MHC class I and MHC class II molecules is regulated by cytokines, in particular interferons, released in the course of immune responses. Interferon-γ (IFN-γ), for example, increases the expression of MHC class I and MHC class II molecules, and can induce the expression of MHC class II molecules on certain cell types that do not normally express them. Interferons also enhance the antigenpresenting function of MHC class I molecules by inducing the expression of key components of the intracellular machinery that enables peptides to be loaded onto the MHC molecules.
3-14. The two classes of MHC molecule have distinct subunit structures but similar three-dimensional structures
The two classes of MHC molecule differ from each other in their structure and also have different distributions on the cells of the body. Their different structures enable the two classes of MHC molecules to serve distinct functions in antigen presentation, binding peptides from different intracellular sites and activating different subsets of T cells, as we will see in Chapter 5. Despite their differences in subunit structure, however, MHC class I and class II molecules are closely related in overall structure. In both classes, the two paired protein domains nearest the membrane resemble immunoglobulin domains, whereas the two domains distal to the membrane fold together to create a long cleft, or groove, which is the site at which a peptide binds. Purified peptide:MHC class I and peptide:MHC class II complexes have been characterized structurally, allowing us to describe in detail both the MHC molecules themselves and the way in which they bind peptides.
MHC class I structure is outlined in Fig. 3.20. MHC class I molecules consist of two polypeptide chains, a larger α chain encoded in the MHC genetic locus, and a smaller noncovalently associated chain, β2-microglobulin, which is not polymorphic and is not encoded in the MHC locus. Only the class I α chain spans the membrane. The complete molecule has four domains, three formed from the MHC-encoded α chain, and one contributed by β2-microglobulin. The α3 domain and β2-microglobulin have a folded structure that closely resembles that of an immunoglobulin domain. The most remarkable feature of MHC class I molecules is the structure of the folded α1 and α2 domains. These two domains form the walls of a cleft on the surface of the molecule; this is the site of peptide binding. They also are sites of polymorphisms that determine T-cell antigen recognition (see Chapter 5).

Figure 3.20
The structure of an MHC class I molecule determined by X-ray crystallography. Panel a shows a computer graphic representation of a human MHC class I molecule, HLA-A2, which has been cleaved from the cell surface by the enzyme papain. The surface of the (more...)
An MHC class II molecule consists of a noncovalent complex of two chains, α and β, both of which span the membrane (Fig. 3.21). The MHC class II α and β chains are both encoded within the MHC. The crystallographic structure of the MHC class II molecule shows that it is folded very much like the MHC class I molecule. The major differences lie at the ends of the peptide-binding cleft, which are more open in MHC class II molecules compared with MHC class I molecules. The main consequence of this is that the ends of a peptide bound to an MHC class I molecule are substantially buried within the molecule, whereas the ends of peptides bound to MHC class II molecules are not. Again, the sites of major polymorphism are located in the peptide-binding cleft, which in the case of an MHC class II molecule are formed by the α1, and β1 domains (see Chapter 5).

Figure 3.21
MHC class II molecules resemble MHC class I molecules in overall structure. The MHC class II molecule is composed of two trans-membrane glycoprotein chains, α (34 kDa) and β (29 kDa), as shown schematically in panel d. Each chain has two (more...)
In both MHC class I and class II molecules, bound peptides are sandwiched between the two α-helical segments of the MHC molecule (Fig. 3.22). The T-cell receptor interacts with this compound ligand, making contacts with both the MHC molecule and with the peptide fragment of antigen.

Figure 3.22
MHC molecules bind peptides tightly within the cleft. When MHC molecules are crystallized with a single synthetic peptide antigen, the details of peptide binding are revealed. In MHC class I molecules (panels a and c) the peptide is bound in an elongated conformation (more...)
3-15. Peptides are stably bound to MHC molecules, and also serve to stabilize the MHC molecule on the cell surface
An individual can be infected by a wide variety of different pathogens the proteins of which will not generally have peptide sequences in common. If T cells are to be alerted to all possible infections, then the MHC molecules on each cell (both class I and class II) must be able to bind stably to many different peptides. This behavior is quite distinct from that of other peptide-binding receptors, such as those for peptide hormones, which usually bind only a single type of peptide. The crystal structures of peptide:MHC complexes have helped to show how a single binding site can bind peptides with high affinity while retaining the ability to bind a wide variety of different peptides.
An important feature of the binding of peptides to MHC molecules is that the peptide is bound as an integral part of the MHC molecule's structure, and MHC molecules are unstable when peptides are not bound. The stability of peptide binding is important, because otherwise, peptide exchanges occurring at the cell surface would prevent peptide:MHC complexes from being reliable indicators of infection or of uptake of specific antigen. As a result of this stability, when MHC molecules are purified from cells, their bound peptides co-purify with them, and this has enabled the peptides bound by specific MHC molecules to be analyzed. The peptides are released from the MHC molecules by denaturing the complex in acid, and can then be purified and sequenced. Pure synthetic peptides can also be incorporated into previously empty MHC molecules and the structure of the complex determined, revealing details of the contacts between the MHC molecule and the peptide. From the sequences of peptides bound to specific MHC molecules, combined with structural analysis of the peptide:MHC complex, a detailed picture of the binding interactions has been built up. We will first discuss the peptide-binding properties of MHC class I molecules.
3-16. MHC class I molecules bind short peptides of 8–10 amino acids by both ends
The binding of a peptide in the peptide-binding cleft of an MHC class I molecule is stabilized at both ends by contacts between atoms in the free amino and carboxy termini of the peptide and invariant sites that are found at each end of the cleft of all MHC class I molecules (Fig. 3.23). These contacts are thought to be the main stabilizing contacts for peptide:MHC class I complexes because synthetic peptide analogues lacking terminal amino and carboxyl groups fail to bind stably to MHC class I molecules. Other residues in the peptide serve as additional anchors. Peptides that bind to MHC class I molecules are usually 8–10 amino acids long. The peptide lies in an elongated conformation along the groove; variations in peptide length appear to be accommodated, in most cases, by a kinking in the peptide backbone. However, two examples of MHC class I molecules where the peptide is able to extend out of the groove at the carboxy terminus suggest that some length variation may also be accommodated in this way.

Figure 3.23
Peptides are bound to MHC class I molecules by their ends. MHC class I molecules interact with the back-bone of a bound peptide (shown in yellow) through a series of hydrogen bonds and ionic interactions (shown as dotted blue lines) at each end of the peptide. (more...)
These interactions give all MHC class I molecules their broad peptide-binding specificity. In addition, MHC molecules are highly polymorphic. There are hundreds of different versions, or alleles, of the MHC class I genes in the human population as a whole, and each individual carries only a small selection of them. The main differences between the allelic MHC variants are found at certain sites in the peptide-binding cleft, resulting in different amino acids in key peptide interaction sites in the different MHC variants. The consequence of this is that the different MHC variants preferentially bind different peptides. The peptides that can bind to a given MHC variant have the same or very similar amino acid residues at two or three particular positions along the peptide sequence. The amino acid side chains at these positions insert into pockets in the MHC molecule that are lined by the polymorphic amino acids. Because the binding of these side chains anchors the peptide to the MHC molecule, the peptide residues involved have been called anchor residues. Both the position and identity of these anchor residues can vary, depending on the particular MHC class I variant that is binding the peptide. However, most peptides that bind to MHC class I molecules have a hydrophobic (or sometimes basic) anchor residue at the carboxy terminus (Fig. 3.24). Changing an anchor residue can prevent the peptide from binding and, conversely, most synthetic peptides of suitable length that contain these anchor residues will bind the appropriate MHC class I molecule, in most cases irrespective of the amino acids at other positions in the peptide. These features of peptide binding enable an individual MHC class I molecule to bind a wide variety of different peptides, yet allow different MHC class I allelic variants to bind different sets of peptides.

Figure 3.24
Peptides bind to MHC molecules through structurally related anchor residues. Peptides eluted from two different MHC class I molecules are shown. The anchor residues (green) differ for peptides that bind different alleles of MHC class I molecules but are (more...)
3-17. The length of the peptides bound by MHC class II molecules is not constrained
Peptide binding to MHC class II molecules has also been analyzed by elution of bound peptides and by X-ray crystallography, and differs in several ways from peptide binding to MHC class I molecules. Peptides that bind to MHC class II molecules are at least 13 amino acids long and can be much longer. The clusters of conserved residues that bind the two ends of a peptide in MHC class I molecules are not found in MHC class II molecules, and the ends of the peptide are not bound. Instead, the peptide lies in an extended conformation along the MHC class II peptide-binding groove. It is held in this groove both by peptide side chains that protrude into shallow and deep pockets lined by polymorphic residues, and by interactions between the peptide backbone and side chains of conserved amino acids that line the peptide-binding cleft in all MHC class II molecules (Fig. 3.25). Although there are fewer crystal structures of MHC class II-bound peptides than of MHC class I, the available data show that amino acid side chains at residues 1, 4, 6, and 9 of an MHC class II-bound peptide can be held in these binding pockets.

Figure 3.25
Peptides bind to MHC class II molecules by interactions along the length of the binding groove. A peptide (yellow; shown as the peptide backbone only, with the amino terminus to the left and the carboxy terminus to the right), is bound by an MHC class (more...)
The binding pockets of MHC class II molecules are more permissive in their accommodation of different amino acid side chains than are those of the MHC class I molecule, making it more difficult to define anchor residues and predict which peptides will be able to bind particular MHC class II molecules (Fig. 3.26). Nevertheless, by comparing the sequences of known binding peptides, it is usually possible to detect a pattern of permissive amino acids for each of the different alleles of MHC class II molecules, and to model how the amino acids of this peptide sequence motif will interact with the amino acids that make up the peptide-binding cleft in the MHC class II molecule. Because the peptide is bound by its backbone and allowed to emerge from both ends of the binding groove there is, in principle, no upper limit to the length of peptides that could bind to MHC class II molecules. However, it appears that longer peptides bound to MHC class II molecules are trimmed by peptidases to a length of 13–17 amino acids in most cases. Like MHC class I molecules, MHC class II molecules that lack bound peptide are unstable, but the critical stabilizing interactions that the peptide makes with the MHC class II molecule are not yet known.

Figure 3.26
Peptides that bind MHC class II molecules are variable in length and their anchor residues lie at various distances from the ends of the peptide. The sequences of a set of peptides that bind to the mouse MHC class II Ak allele are shown in the upper panel. (more...)
3-18. The crystal structures of several MHC:peptide:T-cell receptor complexes all show the same T-cell receptor orientation over the MHC:peptide complex
At the time that the first X-ray crystallographic structure of a T-cell receptor was published, a structure of the same T-cell receptor bound to a peptide:MHC class I ligand was also produced. This structure (Fig. 3.27), which had been forecast by site-directed mutagenesis of the MHC class I molecule, showed the T-cell receptor aligned diagonally over the peptide and the peptide-binding groove, with the T-cell receptor α chain lying over the α2 domain and the amino-terminal end of the bound peptide, the T-cell receptor β chain lying over the α1 domain and the carboxy-terminal end of the peptide, with the CDR3 loops of both T-cell receptor α and T-cell receptor β meeting over the central amino acids of the peptide. The T-cell receptor is threaded through a valley between the two high points on the two surrounding α helices that form the walls of the peptide-binding cleft.

Figure 3.27
The T-cell receptor binds to the MHC:peptide complex. Panel a: the T-cell receptor binds to the top of the MHC:peptide complex, straddling, in the case of the class I molecule shown here, both the α1 and α2 domain helices. The CDRs of the (more...)
Analysis of other MHC class I:peptide:T-cell receptor complexes and of the single example so far of an MHC class II:peptide:T-cell receptor complex (Fig. 3.28) shows that all of them have a very similar orientation, particularly for the Vα domain, although some variability does occur in the location and orientation of the Vβ domain. In this orientation, the Vα domain makes contact primarily with the amino terminus of the bound peptide, whereas the Vβ domain contacts primarily the carboxy terminus of the bound peptide. Both chains also interact with the α helices of the MHC class I molecule (see Fig. 3.27). The T-cell receptor contacts are not symmetrically distributed over the MHC molecule, so whereas the Vα CDR1 and CDR2 loops are in close contact with the helices of the MHC:peptide complex around the amino terminus of the bound peptide, the β-chain CDR1 and CDR2 loops, which interact with the complex at the carboxy terminus of the bound peptide, have variable contributions to the binding. This suggests that the Vα contacts are responsible for the conserved orientation of the T-cell receptor on the MHC:peptide complex.

Figure 3.28
The T-cell receptor interacts with MHC class I and MHC class II molecules in a similar fashion. The structure of a T-cell receptor binding to an MHC class II molecule has been determined, and shows the T-cell receptor binding to an equivalent site, and (more...)
Comparison of the three-dimensional structure of the T-cell receptor to that of the same T-cell receptor complexed to its MHC-peptide ligand could address the question of whether the T-cell receptor, like some other receptors, undergoes a conformational change, or ‘induced fit,’ in its three-dimensional structure when it binds its specific ligand. To date, there is no certain answer, owing to the limitations of the available structures. For one thing, all the T-cell receptors analyzed to date have either been bound to ligands that do not produce activation, or are bound to ligands that can activate them, but the comparable unliganded receptor structures are not available. Also, the crystals of the T-cell receptor are all formed at 0 °C or below, which locks the receptor into a single conformation. However, subtly different peptides can have strikingly different effects when the same T cell recognizes either of the two peptides complexed with MHC. This could be due to differences in how T-cell receptor conformation is altered by binding the two related yet different ligands. Recent evidence also suggests that the temperature at which the T-cell receptor binds to a particular peptide:MHC complex makes a large difference in the extent of T-cell receptor aggregation; protein conformation is affected by temperature, and so these differences may well result from a conformational change.
From an examination of these structures it is hard to predict whether the main binding energy is contributed by T-cell receptor contacts with the bound peptide, or by T-cell receptor contacts with the MHC molecule. It is known that alterations as simple as changing a leucine to isoleucine in the peptide are sufficient to alter the T-cell response from strong killing to absolutely no response at all. Studies show that mutations of single residues in the presenting MHC molecules can have the same effect. Thus, the specificity of T-cell recognition involves both the peptide and its presenting MHC molecule. This dual specificity underlies the MHC restriction of T-cell responses, a phenomenom that was observed long before the peptidebinding properties of MHC molecules were known. We will recount the story of how MHC restriction was discovered when we return to the issue of how MHC polymorphism affects antigen recognition by T cells in Chapter 5. Another consequence of this dual specificity is a need for T-cell receptors to be able to interact appropriately with the antigen-presenting surface of MHC molecules. It appears that there is some inherent specificity for MHC molecules encoded in the T-cell receptor genes, as well as selection during T-cell development for a repertoire of receptors able to interact appropriately with the particular MHC molecules present in that individual. We will be discussing the evidence for this in Chapter 7.
3-19. A distinct subset of T cells bears an alternative receptor made up of γ and δ chains
During the search for the gene for the T-cell receptor α chain, another T-cell receptorlike gene was unexpectedly discovered. This gene was named T-cell receptor γ, and its discovery led to a search for further T-cell receptor genes. Another receptor chain was identified using antibody to the predicted sequence of the γ chain and was called the δ chain. It was soon discovered that a minority population of T cells bore a distinct type of T-cell receptor made up of γ:δ heterodimers rather than α:β heterodimers. The development of these cells is described in Sections 7-13 and 7-14.
To date, there is no crystallographic structure of a γ:δ T-cell receptor, although it is expected to be similar in shape to α:β T-cell receptors. γ:δ T-cell receptors may be specialized to bind certain kinds of ligands, including heat-shock proteins and nonpeptide ligands such as mycobacterial lipid antigens. It seems likely that γ:δ T-cell receptors are not restricted by the ‘classical’ MHC class I and class II molecules. They may bind the free antigen, much as immunoglobulins do, and/or they may bind to peptides or other antigens presented by nonclassical MHC-like molecules. These are proteins that resemble MHC class I molecules but are relatively nonpolymorphic. We still know little about how γ:δ T-cell receptors bind antigen and thus how these cells function, and what their role is in immune responses. The structure and rearrangement of the genes for γ:δ T-cell receptors is covered in Sections 4-13 and 7-13 and the functions of γ:δ T cells are considered in Chapter 8.
Summary
The receptor for antigen on most T cells, the α:β T-cell receptor, is composed of two protein chains, T-cell receptor α and T-cell receptor β, and resembles in many respects a single Fab fragment of immunoglobulin. T-cell receptors are always membrane-bound. α:β T-cell receptors do not recognize antigen in its native state, as do the immunoglobulin receptors of B cells, but recognize a composite ligand of a peptide antigen bound to an MHC molecule. MHC molecules are highly polymorphic glycoproteins encoded by genes in the major histocompatibility complex (MHC). Each MHC molecule binds a wide variety of different peptides, but the different variants each preferentially recognize sets of peptides with particular sequence and physical features. The peptide antigen is generated intracellularly, and bound stably in a peptide-binding cleft on the surface of the MHC molecule. There are two classes of MHC molecules and these are bound in their nonpolymorphic domains by CD8 and CD4 molecules that distinguish two different functional classes of α:β T cells. CD8 binds MHC class I molecules and can bind simultaneously to the same class I MHC:peptide complex being recognized by a T-cell receptor, thus acting as a co-receptor and enhancing the T-cell response; CD4 binds MHC class II molecules and acts as a co-receptor for T-cell receptors that recognise class II MHC:peptide ligands. T-cell receptors interact directly with both the antigenic peptide and polymorphic features of the MHC molecule that displays it, and this dual specificity underlies the MHC restriction of T-cell responses. A second type of T-cell receptor, composed of a γ and a δ chain, is structurally similar to the α:β T-cell receptor but appears to bind different ligands, including nonpeptide ligands. It is not thought to be MHC restricted. It is found on a minority population of T cells, the γ:δ T cells, whose biological function is still not clear.
- The antigen receptor on T cells is very similar to a Fab fragment of immunoglobulin
- A T-cell receptor recognizes antigen in the form of a complex of a foreign peptide bound to an MHC molecule
- T cells with different functions are distinguished by CD4 and CD8 cell-surface proteins and recognize peptides bound to different classes of MHC molecule
- The two classes of MHC molecule are expressed differentially on cells
- The two classes of MHC molecule have distinct subunit structures but similar three-dimensional structures
- Peptides are stably bound to MHC molecules, and also serve to stabilize the MHC molecule on the cell surface
- MHC class I molecules bind short peptides of 8–10 amino acids by both ends
- The length of the peptides bound by MHC class II molecules is not constrained
- The crystal structures of several MHC:peptide:T-cell receptor complexes all show the same T-cell receptor orientation over the MHC:peptide complex
- A distinct subset of T cells bears an alternative receptor made up of γ and δ chains
- Summary
- Antigen recognition by T cells - ImmunobiologyAntigen recognition by T cells - Immunobiology
- LOC114403114 [Glycine soja]LOC114403114 [Glycine soja]Gene ID:114403114Gene
Your browsing activity is empty.
Activity recording is turned off.
See more...