By agreement with the publisher, this book is accessible by the search feature, but cannot be browsed.
NCBI Bookshelf. A service of the National Library of Medicine, National Institutes of Health.
Alberts B, Johnson A, Lewis J, et al. Molecular Biology of the Cell. 4th edition. New York: Garland Science; 2002.
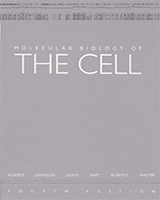
Molecular Biology of the Cell. 4th edition.
Show detailsThree types of cytoskeletal filaments are common to many eucaryotic cells and are fundamental to the spatial organization of these cells. Intermediate filaments provide mechanical strength and resistance to shear stress. Microtubules determine the positions of membrane-enclosed organelles and direct intracellular transport. Actin filaments determine the shape of the cell's surface and are necessary for whole-cell locomotion (Panel 16-1). But these cytoskeletal filaments would be ineffective on their own. Their usefulness to the cell depends on a large number of accessory proteins that link the filaments to other cell components, as well as to each other. This set of accessory proteins is essential for the controlled assembly of the cytoskeletal filaments in particular locations, and it includes the motor proteins that either move organelles along the filaments or move the filaments themselves.
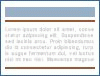
Panel 16-1
The Three Major Types of Protein that Form the Cytoskeleton.
Cytoskeletal systems are dynamic and adaptable, organized more like ant trails than interstate highways. A single trail of ants may persist for many hours, extending from the ant nest to a delectable picnic site, but the individual ants within the trail are anything but static. If the ant scouts find a new and better source of food, or if the picnickers clean up and leave, the dynamic structure rearranges itself with astonishing rapidity, to deal with the new situation. In a similar way, large-scale cytoskeletal structures can change or persist, according to need, lasting for lengths of time ranging from less than a minute up to the lifetime of the cell. The individual macromolecular components that make up these structures are in a constant state of flux. Thus, like the alteration of an ant trail, a structural rearrangement in a cell requires little extra energy when conditions change. In this section, we discuss the remarkable mechanisms that cause cytoskeletal filaments to be dynamic, and thereby able to respond rapidly to any eventuality.
Regulation of the dynamic behavior and assembly of the cytoskeletal filaments allows eucaryotic cells to build an enormous range of structures from the three basic filament systems. Micrographs that reveal some of these structures are shown in Panel 16-1. Microtubules, which are frequently found in a star-like cytoplasmic array emanating from the center of an interphase cell, can quickly rearrange themselves to form a bipolar mitotic spindle during cell division. They can also form motile whips called cilia and flagella on the surface of the cell, or tightly aligned bundles that serve as tracks for the transport of materials down long neuronal axons. Actin filaments form many types of cell-surface projections. Some of these are dynamic structures, such as the lamellipodia and filopodia that cells use to explore territory and pull themselves around. Others are stable structures such as the regular bundles of stereocilia on the surface of hair cells in the inner ear, which tilt as rigid rods in response to sound. Inside cells, actin filaments can also form either transient or stable structures: the contractile ring, for example, assembles transiently to divide cells in two during cytokinesis; more stable arrays allow cells to brace themselves against an underlying substratum and enable muscle to contract. Intermediate filaments line the inner face of the nuclear envelope, forming a protective cage for the cell's DNA; in the cytosol, they are twisted into strong cables that can hold epithelial cell sheets together, help neuronal cells to extend long and robust axons, or allow us to form tough appendages such as hair and fingernails.
Each Type of Cytoskeletal Filament Is Constructed from Smaller Protein Subunits
Cytoskeletal structures frequently reach all the way from one end of the cell to the other, spanning tens or even hundreds of micrometers. Yet the individual protein molecules of the cytoskeleton are generally only a few nanometers in size. The cell is able to build the large structures by the repetitive assembly of large numbers of the small subunits, like building a skyscraper out of bricks. Because these subunits are small, they can diffuse rapidly within cytoplasm, whereas the assembled filaments cannot. In this way, cells can undergo rapid structural reorganizations, disassembling filaments at one site and reassembling them at another site far away (Figure 16-2).

Figure 16-2
The cytoskeleton and changes in cell shape. The formation of protein filaments from much smaller protein subunits allows regulated filament assembly and disassembly to reshape the cytoskeleton. (A) Filament formation from a small protein. (B) Rapid reorganization (more...)
Intermediate filaments are made up of smaller subunits that are themselves elongated and fibrous, whereas actin filaments and microtubules are made of subunits that are compact and globular—actin subunits for actin filaments, tubulin subunits for microtubules. All three types of cytoskeletal filaments form as helical assemblies of subunits (see Figure 3-27), which self-associate, using a combination of end-to-end and side-to-side protein contacts. Differences in the structures of the subunits and the strengths of the attractive forces between them produce critical differences in the stability and mechanical properties of each type of filament.
Many biological polymers—including DNA, RNA, and proteins—are held together by covalent linkages between their subunits. In contrast, the three types of cytoskeletal “polymers” are held together by weak noncovalent interactions, which means that their assembly and disassembly can occur rapidly, without covalent bonds being formed or broken.
Within the cell, hundreds of different cytoskeleton-associated accessory proteins regulate the spatial distribution and the dynamic behavior of the filaments. These accessory proteins bind to the filaments or their subunits to determine the sites of assembly of new filaments, to regulate the partitioning of polymer proteins between filament and subunit forms, to change the kinetics of filament assembly and disassembly, to harness energy to generate force, and to link filaments to one another or to other cellular structures such as organelles and the plasma membrane. In these processes, the accessory proteins bring cytoskeletal structure under the control of extracellular and intracellular signals, including those that trigger the dramatic transformations of the cytoskeleton that occur during the cell cycle. Acting together, the accessory proteins enable a eucaryotic cell to maintain a highly organized but flexible internal structure and, in many cases, to move.
Filaments Formed from Multiple Protofilaments Have Advantageous Properties
In general, the linking of protein subunits together to form a filament can be thought of as a simple association reaction. A free subunit binds to the end of a filament that contains n subunits to generate a filament of length n + 1. The addition of each subunit to the end of the polymer creates a new end to which yet another subunit can bind. However, the robust cytoskeletal filaments in living cells are not built by simply stringing subunits together in this way in a single straight file. A thousand tubulin monomers, for example, lined up end to end, would be enough to span the diameter of a small eucaryotic cell, but a filament formed in this way would not have enough strength to avoid breakage by ambient thermal energy, unless each subunit were bound very tightly to its neighbor. Such tight binding would limit the rate at which the filaments could disassemble, making the cytoskeleton a static and less useful structure.
Cytoskeletal polymers combine strength with adaptability because they are built out of multiple protofilaments—long linear strings of subunits joined end to end—that associate with one another laterally. Typically, the protofilaments twist around one another in a helical lattice. The addition or loss of a subunit at the end of one protofilament makes or breaks one set of longitudinal bonds and either one or two sets of lateral bonds. In contrast, breakage of the composite filament in the middle requires breaking sets of longitudinal bonds in several protofilaments all at the same time (Figure 16-3). The large energy difference between these two processes allows most cytoskeletal filaments to resist thermal breakage, while leaving the filament ends as dynamic structures in which addition and loss of subunits occur rapidly.

Figure 16-3
The thermal stability of cytoskeletal filaments with dynamic ends. Formation of a cytoskeletal filament from more than one protofilament allows the ends to be dynamic, while the filaments themselves are resistant to thermal breakage. In this hypothetical (more...)
As with other specific protein-protein interactions, the subunits in a cytoskeletal filament are held together by a large number of hydrophobic interactions and weak noncovalent bonds (see Figure 3-5). The locations and types of subunit-subunit contacts are different for the different cytoskeletal filaments. Intermediate filaments, for example, assemble by forming strong lateral contacts between α-helical coiled coils, which extend over most of the length of each elongated fibrous subunit. Because the individual subunits are staggered in the filament, intermediate filaments tolerate stretching and bending, forming strong rope-like structures (Figure 16-4). Microtubules, by contrast, are built from globular subunits held together primarily by longitudinal bonds, and the lateral bonds holding the 13 protofilaments together are comparatively weak. For this reason, microtubules break much more easily than do intermediate filaments.

Figure 16-4
A strong filament formed from elongated fibrous subunits with strong lateral contacts. Intermediate filaments are formed in this way and are consequently especially resistant to bending or stretching forces.
Nucleation Is the Rate-limiting Step in the Formation of a Cytoskeletal Polymer
There is an important additional consequence of the multiple-protofilament organization of cytoskeletal polymers. Short oligomers composed of a few subunits can assemble spontaneously, but they are unstable and disassemble readily because each monomer is bonded only to a few other monomers. For a new large filament to form, subunits must assemble into an initial aggregate, or nucleus, that is stabilized by many subunit-subunit contacts and can then elongate rapidly by addition of more subunits. The initial process of nucleus assembly is called filament nucleation, and it can take quite a long time, depending on how many subunits must come together to form the nucleus. The instability of smaller aggregates creates a kinetic barrier to nucleation, which is easily observed in a solution of pure actin or tubulin—the subunits of actin filaments and microtubules, respectively. When polymerization is initiated in a test tube containing a solution of pure individual subunits (by raising the temperature or raising the salt concentration), there is an initial lag phase, during which no filaments are observed. During this lag phase, however, nuclei are assembling slowly, so that the lag phase is followed by a phase of rapid filament elongation, during which subunits add quickly onto the ends of the nucleated filaments (Figure 16-5A). Finally, the system approaches a steady state at which the rate of addition of new subunits to the filament ends is exactly balanced by the rate of subunit dissociation from the ends. The concentration of free subunits left in solution at this point is called the critical concentration, Cc. As explained in Panel 16-2, the value of the critical concentration is equal to the rate constant for subunit loss divided by the rate constant for subunit addition—that is, Cc = koff/kon.
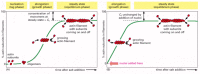
Figure 16-5
The time course of actin polymerization in a test tube. (A) Polymerization is begun by raising the salt concentration in a solution of pure actin subunits. (B) Polymerization is begun in the same way, but with preformed fragments of actin filaments present (more...)
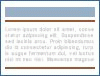
Panel 16-2
The Polymerization of Actin and Tubulin.
The lag phase in filament growth is eliminated if preexisting nuclei (such as filament fragments that have been chemically cross-linked) are added to the solution at the beginning of the polymerization reaction (Figure 16-5B). The cell takes great advantage of this nucleation requirement: it uses special proteins to catalyze filament nucleation at specific sites, thereby determining the location where new cytoskeletal filaments are assembled. Indeed, this type of regulation of filament nucleation is a primary way that cells control their shape and movement.
The Tubulin and Actin Subunits Assemble Head-to-Tail, Creating Filaments that Are Polar
Microtubules are formed from protein subunits of tubulin. The tubulin subunit is itself a heterodimer formed from two closely related globular proteins called α-tubulin and β-tubulin, tightly bound together by noncovalent bonds (Figure 16-6). These two tubulin proteins are found only in this complex. Each α or β monomer has a binding site for one molecule of GTP. The GTP that is bound to the α-tubulin monomer is physically trapped at the dimer interface and is never hydrolyzed or exchanged; it can therefore be considered to be an integral part of the tubulin heterodimer structure. The nucleotide on the β-tubulin, in contrast, may be in either the GTP or the GDP form, and it is exchangeable. As we see, the hydrolysis of GTP at this site to produce GDP has an important effect on microtubule dynamics.
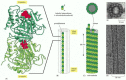
Figure 16-6
The structure of a microtubule and its subunit. (A) The subunit of each protofilament is a tubulin heterodimer, formed from a very tightly linked pair of α- and β-tubulin monomers. The GTP molecule in the α-tubulin monomer is so (more...)
A microtubule is a stiff, hollow cylindrical structure built from 13 parallel protofilaments, each composed of alternating α-tubulin and β-tubulin molecules. When the tubulin heterodimers assemble to form the hollow cylindrical microtubule, they generate two new types of protein-protein contacts. Along the longitudinal axis of the microtubule, the “top” of one β-tubulin molecule forms an interface with the “bottom” of the α-tubulin molecule in the adjacent dimer subunit. This interface is very similar to the interface holding the α and β monomers together in the dimer subunit, and the binding energy is strong. Perpendicular to these interactions, lateral contacts are formed between neighboring protofilaments. In this dimension, the main lateral contacts are between monomers of the same type (α-α and β-β). Together, the longitudinal and lateral contacts are repeated in the regular helical lattice of the microtubule. Because most of the subunits in a microtubule are held in place by multiple contacts within the lattice, the addition and loss of subunits occurs almost exclusively at the microtubule ends (see Figure 16-3).
Each protofilament in a microtubule is assembled from subunits that all point in the same direction, and the protofilaments themselves are aligned in parallel (in Figure 16-6, for example, the α-tubulin is down and the β-tubulin up in each heterodimer). Therefore, the microtubule itself has a distinct structural polarity, with α-tubulins exposed at one end and β-tubulins exposed at the other end.
The actin subunit is a single globular polypeptide chain and is thus a monomer rather than a dimer. Like tubulin, each actin subunit has a binding site for a nucleotide, but for actin the nucleotide is ATP (or ADP) rather than GTP (or GDP) (Figure 16-7). As for tubulin, the actin subunits assemble head-to-tail to generate filaments with a distinct structural polarity. The actin filament can be considered to consist of two parallel protofilaments that twist around each other in a right-handed helix. Actin filaments are relatively flexible compared with the hollow cylindrical microtubules. But in a living cell, actin filaments are cross-linked and bundled together by a variety of accessory proteins (see below), making these large-scale actin structures much stronger than an individual actin filament.

Figure 16-7
The structures of an actin monomer and actin filament. (A) The actin monomer has a nucleotide (either ATP or ADP) bound in a deep cleft in the center of the molecule. (B) Arrangement of monomers in a filament. Although the filament is often described (more...)
The Two Ends of a Microtubule and of an Actin Filament Are Distinct and Grow at Different Rates
The structural polarity of actin filaments and microtubules is created by the regular, parallel orientation of all of their subunits. This orientation makes the two ends of each polymer different in ways that have a profound effect on filament growth rates. Addition of a subunit to either end of a filament of n subunits results in a filament of n + 1 subunits. In the absence of ATP or GTP hydrolysis, the free energy difference, and therefore the equilibrium constant (and the critical concentration), must be the same for addition of subunits at either end of the polymer. In this case, the ratio of the forward and backward rate constants, kon/koff, must be identical at the two ends, even though the absolute values of these rate constants may be very different at each end.
In a structurally polar filament, the kinetic rate constants for association and dissociation—kon and koff, respectively—are often much greater at one end than at the other. Thus, if an excess of purified subunits is allowed to assemble onto marked fragments of preformed filaments, one end of each fragment elongates much faster than the other (Figure 16-8). If filaments are rapidly diluted so that the free subunit concentration drops below the critical concentration, the fast-growing end also depolymerizes fastest. The more dynamic of the two ends of a filament, where both growth and shrinkage are fast, is called the plus end, and the other end is called the minus end.

Figure 16-8
The preferential growth of microtubules at the plus end. Both microtubules and actin filaments grow faster at one end than at the other. In this case, a stable bundle of microtubules obtained from the core of a cilium (discussed later) was incubated with (more...)
On microtubules, α subunits are exposed at the minus end, and β subunits are exposed at the plus end. On actin filaments, the ATP-binding cleft on the monomer points toward the minus end. (For historical reasons, the plus ends of actin filaments are usually referred to as “barbed” ends, and minus ends as “pointed” ends, because of the arrowhead appearance of myosin heads when bound along the filament.)
Filament elongation proceeds spontaneously when the free energy change (ΔG) for addition of the soluble subunit is less than zero. This is the case when the concentration of subunits in solution exceeds the critical concentration. Likewise, filament depolymerization proceeds spontaneously when this free energy change is greater than zero. A cell can couple an energetically unfavorable process to these spontaneous processes; thus, the free energy released during spontaneous filament polymerization or depolymerization can be used to do mechanical work—in particular, to push or pull an attached load. For example, elongating microtubules can help push out membranes, and shrinking microtubules can help pull mitotic chromosomes away from their sisters during anaphase. Similarly, elongating actin filaments help protrude the leading edge of motile cells, as we discuss later.
Filament Treadmilling and Dynamic Instability Are Consequences of Nucleotide Hydrolysis by Tubulin and Actin
Thus far, our discussion of filament dynamics has ignored a critical fact that applies to both actin filaments and microtubules. In addition to their ability to form noncovalent polymers, the actin and tubulin subunits are both enzymes that can catalyze the hydrolysis of a nucleoside triphosphate, ATP or GTP, respectively. For the free subunits, this hydrolysis proceeds very slowly; however, it is accelerated when the subunits are incorporated into filaments. Shortly after incorporation of an actin or tubulin subunit into a filament, nucleotide hydrolysis occurs; the free phosphate group is released from each subunit, but the nucleoside diphosphate remains trapped in the filament structure. (On tubulin, the nucleotide-binding site lies at the interface between two neighboring subunits—see Figure 16-6, whereas in actin, the nucleotide is deep in a cleft near the center of the subunit—see Figure 16-7.) Thus, two different types of filament structures can exist, one with the “T form” of the nucleotide bound (ATP for actin, GTP for tubulin), and one with the “D form” bound (ADP for actin, GDP for tubulin).
When the nucleotide is hydrolyzed, much of the free energy released by cleavage of the high-energy phosphate-phosphate bond is stored in the polymer lattice, making the free energy change upon dissociation of the D-form polymer higher than the free energy change upon dissociation of the T-form polymer. Consequently, the equilibrium constant for dissociation KD = koff/kon for the D-form polymer, which is numerically equal to its critical concentration [Cc(D)], is larger than the corresponding equilibrium constant for the T-form polymer. Thus, Cc(D) is greater than Cc(T). For certain concentrations of free subunits, D-form polymers will therefore shrink while T-form polymers grow.
In living cells, most of the free subunits are in the T form, as the free concentration of both ATP and GTP is much higher than that of ADP and GDP. The longer the time that subunits have been in the polymer lattice, the more likely they are to have hydrolyzed their bound nucleotide. Whether the subunit at the very end of a filament is in the T or the D form will depend on the relative rates of hydrolysis and subunit addition. If the rate of subunit addition is high, that is if the filament is growing rapidly, then it is likely that a new subunit will add on to the polymer before the nucleotide in the previously added subunit has been hydrolyzed, so that the tip of the polymer remains in the T form, forming an ATP cap or GTP cap. However, if the rate of subunit addition is low, hydrolysis may occur before the next subunit is added, and the tip of the filament will then be in the D form.
The rate of subunit addition at the end of a filament is the product of the free subunit concentration and the rate constant kon. The kon is much faster for the plus end of a filament than for the minus end because of a structural difference between the two ends (see Panel 16-2, pp. 912–913). At an intermediate concentration of free subunits, it is therefore possible for the rate of subunit addition to be faster than nucleotide hydrolysis at the plus end, but slower than nucleotide hydrolysis at the minus end. In this case, the plus end of the filament remains in the T conformation, while the minus end adopts the D conformation. As just explained, the D form has a higher critical concentration than the T form; in other words, the D form leans more readily toward disassembly, while the T form leans more readily toward assembly. If the concentration of free subunits in solution is in an intermediate range—higher than the critical concentration of the T form (that is, the plus end), but lower than the critical concentration of the D form (that is, the minus end)—the filament adds subunits at the plus end, and simultaneously loses subunits from the minus end. This leads to the remarkable property of filament treadmilling (Figure 16-9 and Panel 16-2).

Figure 16-9
The treadmilling of an actin filament or microtubule, made possible by the nucleoside triphosphate hydrolysis that follows subunit addition. (A) Explanation for the different critical concentrations (Cc) at the plus and minus ends. Subunits with bound (more...)
During treadmilling, subunits are recruited at the plus end of the polymer in the T form and shed from the minus end in the D form. The ATP or GTP hydroly-sis that occurs along the way gives rise to the difference in the free energy of the association/dissociation reactions at the plus and minus ends of the actin filament or microtubule and thereby makes treadmilling possible. At a particular intermediate subunit concentration, the filament growth at the plus end is exactly balanced by the filament shrinkage at the minus end. Now, the subunits cycle rapidly between the free and filamentous states, while the total length of the filament remains unchanged. This “steady-state treadmilling” requires a constant consumption of energy in the form of nucleoside triphosphate hydrolysis. While the extent of treadmilling inside the cell is uncertain, the treadmilling of single filaments has been observed in vitro for actin, and a phenomenon that looks like treadmilling can be observed in live cells for individual microtubules (Figure 16-10).
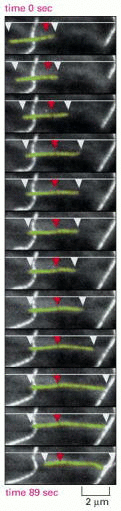
Figure 16-10
Treadmilling behavior of a microtubule, as observed in a living cell. A cell was injected with tubulin that had been covalently linked to the fluorescent dye rhodamine, so that approximately 1 tubulin subunit in 20 was fluorescent. The fluorescence of (more...)
The kinetic differences between the behavior of the T form and the D form have another important consequence for the behaviors of filaments. If the rate of subunit addition at one end is similar in magnitude to the rate of hydrolysis, there is a finite probability that this end will start out in a T form, but that hydrolysis will eventually “catch up” with the addition and transform the end to a D form. This transformation is sudden and random, with a certain probability per unit time. Thus, in a population of microtubules, at any instant some of the ends are in the T form, and some are in the D form, with the ratio depending on the hydrolysis rate and the free subunit concentration.
Suppose that the concentration of free subunits is intermediate between the critical concentration for a T-form end and the critical concentration for a D-form end (that is, in the same range of concentrations where treadmilling is observed). Now, any end that happens to be in the T form will grow, whereas any end that happens to be in the D form will shrink. On a single filament, an end might grow for a certain length of time in a T form, but then suddenly change to the D form and begin to shrink rapidly, even while the free subunit concentration is held constant. At some later time, it might then regain a T-form and begin to grow again. This rapid interconversion between a growing and shrinking state, at a uniform free subunit concentration, is called dynamic instability (Figure 16-11). The change to rapid shrinkage is called a catastrophe, while the change to growth is called a rescue. In cells, dynamic instability is thought to predominate in microtubules, whereas treadmilling may predominate in actin filaments.

Figure 16-11
Dynamic instability due to the structural differences between a growing and a shrinking microtubule end. (A) If the free tubulin concentration in solution is between the critical values indicated in Figure 16-9, a single microtubule end may undergo transitions (more...)
For microtubules, the structural difference between a T-form end and a D-form end is dramatic. Tubulin subunits with GTP bound to the β-monomer produce straight protofilaments that make strong and regular lateral contacts with one another. But the hydrolysis of GTP to GDP is associated with a subtle conformational change in the protein, which makes the protofilaments curved. On a rapidly growing microtubule, the GTP cap constrains the curvature of the protofilaments, and the ends appear straight. But when the terminal subunits have hydrolyzed their nucleotides, this constraint is released, and the curved protofilaments spring apart. This cooperative release of the energy of hydrolysis stored in the microtubule lattice results in the curled protofilaments peeling off rapidly, and rings and curved oligomers of GDP-containing tubulin are seen near the ends of depolymerizing microtubules (Figure 16-11C).
Treadmilling and Dynamic Instability Require Energy but Are Useful
Both dynamic instability and treadmilling allow a cell to maintain the same overall filament content, while individual subunits constantly recycle between the filaments and the cytosol. How dynamic are the microtubules and actin filaments inside a living cell? Typically, a microtubule, with major structural differences between its growing and shrinking ends, switches between growth and shrinkage a few times per minute. The ends of individual microtubules can therefore be seen in real time to exhibit such dynamic instability (Figure 16-12). For actin filaments, by contrast, the difference between the two types of ends is not so extreme, and the dynamic instability of an individual filament cannot be observed directly with the light microscope. With appropriate techniques based on fluorescence microscopy, however, one can show that actin filament turnover is typically rapid, with individual filaments persisting for only a few minutes.

Figure 16-12
Direct observation of the dynamic instability of microtubules in a living cell. Microtubules in a newt lung epithelial cell were observed after the cell was injected with a small amount of rhodamine labeled tubulin, as in Figure 16-10. The dynamic instability (more...)
At first glance, this dynamic behavior of filaments seems like a waste of energy. To maintain a constant concentration of actin filaments and microtubules, most of which are undergoing a process of either treadmilling or dynamic instability, the cell must hydrolyze large amounts of nucleoside triphosphate. As we explained with our ant-trail analogy at the beginning of the chapter, the advantage to the cell seems to lie in the spatial and temporal flexibility that is inherent in a structural system with constant turnover. Individual subunits are small and can diffuse very rapidly; an actin or tubulin subunit can diffuse across the diameter of a typical eucaryotic cell in a second or two. As noted above, the rate-limiting step in the formation of a new filament is nucleation, so these rapidly diffusing subunits tend to assemble either on the ends of preexisting filaments or at special sites where the nucleation step is catalyzed by special proteins. The new filaments in either case are highly dynamic, and unless specifically stabilized, they have only a fleeting existence. By controlling where filaments are nucleated and selectively stabilized, a cell can control the location of its filament systems, and hence its structure. It seems that the cell is continually testing a wide variety of internal structures and only preserving those that are useful. When external conditions change, or when internal signals arise (as during the transitions in the cell cycle), the cell is poised to change its structure rapidly (Figure 16-13).
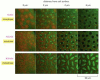
Figure 16-13
The rapid changes in cytoskeletal organization observed during the development of a Drosophila early embryo. In this giant multinuclear cell, the early nuclear divisions occur every 10 minutes or so in a common cytoplasm. The rapid rearrangements of the (more...)
In certain specialized structures, particularly in various specialized cells in a multicellular organism, parts of the cytoskeleton become less dynamic. In a terminally differentiated cell such as a neuron, for example, it is desirable to maintain a consistent structure over time, and many of the actin filaments and microtubules are stabilized by association with other proteins. However, when new connections are made in the brain, as when the information you are reading here is transferred into long-term memory, even a cell as stable as a neuron can grow new processes to make new synapses. To do this, a neuron requires the inherently dynamic and exploratory activities of its cytoskeletal filaments.
Other Polymeric Proteins Also Use Nucleotide Hydrolysis to Couple a Conformational Change to Cell Movements
It is remarkable that actin and tubulin have both evolved nucleoside triphosphate hydrolysis for the same basic reason—to enable them to depolymerize readily after they have polymerized. Actin and tubulin are completely unrelated in amino acid sequence: actin is distantly related in structure to the glycolytic enzyme hexokinase, whereas tubulin is distantly related to a large family of GTPases that includes the heterotrimeric G proteins and monomeric GTPases such as Ras, structures that are discussed in Chapter 3.
Several other types of protein polymers bind and hydrolyze nucleoside triphosphates. For example, dynamin assembles in helical coils around the base of membrane invaginations, helping to pinch off the invaginations to form vesicles (see Figure 13-9). Hydrolysis of GTP by dynamin generates a cooperative conformational change in the dynamin coil and provides the energy for the constriction. Bacteria and archaea have a tubulin homolog called FtsZ that is essential for cell division. A band of FtsZ protein forms at the site of septation, where the new cell wall is to form. Constriction and disassembly of the FtsZ band then help to pinch the two daughter cells apart. In vitro, FtsZ forms protofilaments and rings reminiscent of tubulin, and it also binds and hydrolyzes GTP (Figure 16-14). The increase in ring curvature and disassembly of the FtsZ filaments is similar to the disassembly of curved GDP-tubulin protofilaments described earlier (see Figure 16-11), and it may occur by a similar mechanism.

Figure 16-14
The bacterial FtsZ protein, a tubulin homolog in procaryotes. (A) A band of FtsZ protein forms a ring in a dividing bacterial cell. This ring has been labeled by fusing the FtsZ protein to the green fluorescent protein (GFP) and observing the living (more...)
These examples point to a second general way in which protein polymers can use the energy of nucleotide hydrolysis to affect cell structure and dynamics. In a linear protein polymer, a modest hydrolysis-linked conformational change by a few tenths of a nanometer in each subunit can be amplified by the thousands of subunits acting in parallel, so as to drive movements over tens or hundreds of nanometers. For dynamin and FtsZ, apparently, the effect of the GTP-dependent conformational changes is to bend lipid bilayers.
Tubulin and Actin Have Been Highly Conserved During Eucaryotic Evolution
Tubulin is found in all eucaryotic cells, and FtsZ has been found in all bacteria and archaea examined. Rod-shaped and spiral-shaped bacteria also contain an actin homolog, MreB, that forms filaments and regulates bacterial length. Thus, the principle of organizing cell structure by a self-association of nucleotide-binding proteins seems to be used in all cells.
Tubulin molecules themselves come in multiple isoforms. In mammals, there are at least six forms of α-tubulin and a similar number of forms of β-tubulin, each encoded by a different gene. The different forms of tubulin are very similar, and they generally copolymerize into mixed microtubules in the test tube, although they can have distinct locations in the cell and perform subtly different functions. As a particularly striking example, the microtubules in six specialized touch-sensitive neurons in the nematode Caenorhabditis elegans contain a specific form of β-tubulin, and mutations in the gene for this protein result in the loss of touch-sensitivity with no apparent defect in other cell functions.
Yeast and human tubulins are 75% identical in amino acid sequence. Most of the variation among different isoforms of tubulin is found in the amino acids near the C-terminus, which form a ridge on the surface of the microtubule. Thus, variations among isoforms are expected to affect primarily the association of accessory proteins with the surface of the microtubule, rather than microtubule polymerization per se.
Like tubulin, actin is found in all eucaryotic cells. Most organisms have multiple genes encoding actin; humans have six. Actin is extraordinarily well conserved among eucaryotes. The amino acid sequences of actins from different species are usually about 90% identical. But, again like tubulin, small variations in actin amino acid sequence can create significant functional differences. In vertebrates, there are three subtly different isoforms of actin, termed α, β, and γ, that differ slightly in their amino acid sequences. The α-actin is expressed only in muscle cells, while β and γ are found together in almost all nonmuscle cells. Yeast actin and Drosophila muscle actin are 89% identical, yet the expression of yeast actin in Drosophila results in a fly that looks normal but is unable to fly.
What is the explanation for the unusually strict conservation of actin and tubulin in eucaryotic evolution? Most other cytoskeletal proteins, including intermediate filament proteins and the large families of accessory proteins that bind to actin or tubulin, are not particularly well conserved at the level of amino acid sequence. The likely explanation is that the structure of the entire surface of an actin filament or microtubule is constrained because so many other proteins must be able to interact with these two ubiquitous and abundant cell components. A mutation in actin that could result in a desirable change in its interaction with one other protein might cause undesirable changes in its interactions with a number of other proteins that bind at or near the same site. Genetic and biochemical studies in the yeast Saccharomyces cerevisiae have demonstrated that actin interacts directly with dozens of other proteins, and indirectly with even more (Figure 16-15). Over time, evolving organisms have found it more profitable to leave actin and tubulin alone, and alter their binding partners instead.

Figure 16-15
Actin at the crossroads. Actin binds to a very large variety of accessory proteins in all eucaryotic cells. This diagram shows most of the interactions that have been demonstrated, using either genetic or biochemical techniques, in the yeast Saccharomyces (more...)
Intermediate Filament Structure Depends on The Lateral Bundling and Twisting of Coiled Coils
All eucaryotic cells contain actin and tubulin. But the third major type of cytoskeletal filament, the intermediate filament, is found in only some metazoans, including vertebrates, nematodes, and molluscs. Even in these organisms, intermediate filaments are not required in every cell type. The specialized glial cells (called oligodendrocytes) that make myelin in the vertebrate central nervous system, for example, do not contain intermediate filaments. Cytoplasmic intermediate filaments are closely related to their ancestors, the much more widely used nuclear lamins. The nuclear lamins are filamentous proteins that form a meshwork lining the inner membrane of the eucaryotic nuclear envelope, where they provide anchorage sites for chromosomes and nuclear pores (their dynamic behavior during cell division is discussed in Chapter 12). Several times during metazoan evolution, lamin genes have apparently duplicated, and the duplicates have evolved to produce rope-like, cytoplasmic intermediate filaments. These filaments have mechanical properties that are especially useful to soft-bodied animals such as nematodes and vertebrates that do not have an exoskeleton. Intermediate filaments are particularly prominent in the cytoplasm of cells that are subject to mechanical stress, and their major function seems to be to impart physical strength to cells and tissues.
The individual polypeptides of intermediate filaments are elongated molecules with an extended central α-helical domain that forms a parallel coiled coil with another monomer. A pair of parallel dimers then associates in an antiparallel fashion to form a staggered tetramer. This tetramer represents the soluble subunit that is analogous to the αβ-tubulin dimer, or the actin monomer (Figure 16-16).

Figure 16-16
A model of intermediate filament construction. The monomer shown in (A) pairs with an identical monomer to form a dimer (B), in which the conserved central rod domains are aligned in parallel and wound together into a coiled coil. (C) Two dimers then (more...)
Since the tetrameric subunit is made up of two dimers pointing in opposite directions, its two ends are the same. The assembled intermediate filament therefore lacks the overall structural polarity that is critical for actin filaments and microtubules. The tetramers pack together laterally to form the filament, which includes eight parallel protofilaments made up of tetramers. Each individual intermediate filament therefore has a cross section of 32 individual α-helical coils. This large number of polypeptides all lined up together, with the strong lateral hydrophobic interactions typical of coiled-coil proteins, gives intermediate filaments a rope-like character. They can be easily bent but are extremely difficult to break (Figure 16-17).

Figure 16-17
Mechanical properties of actin, tubulin, and intermediate filament polymers. Networks composed of microtubules, actin filaments, or a type of intermediate filament called vimentin, all at equal concentration, were exposed to a shear force in a viscometer, (more...)
Less is understood about the mechanism of assembly and disassembly of intermediate filaments than of actin filaments and microtubules, but they are clearly highly dynamic structures in most cell types. Under normal conditions, protein phosphorylation probably regulates their disassembly, in much the same way that phosphorylation regulates the disassembly of nuclear lamins in mitosis (see Figure 12-20). As evidence for a rapid turnover, labeled subunits microinjected into tissue culture cells rapidly add themselves onto the existing intermediate filaments within a few minutes, while an injection of peptides derived from a conserved helical region of the subunit induces the rapid disassembly of the intermediate filament network. Interestingly, the latter injection can induce the disassembly of the microtubule and actin filament networks in some cells that contain all three networks revealing that there is a fundamental mechanical integration among the different cytoskeletal systems in these cells.
Intermediate Filaments Impart Mechanical Stability to Animal Cells
Intermediate filaments come in a wide variety of types, with substantially more sequence variation in the subunit isoforms than occurs in the isoforms of actin or tubulin. A central α-helical domain has 40 or so heptad repeat motifs that form an extended coiled-coil (see Figure 3-11). This domain is similar in the different isoforms, but the N- and C-terminal globular domains can vary a great deal.
Different families of intermediate filaments are expressed in different cell types (Table 16-1). The most diverse intermediate filament family is that of the keratins: there are about 20 found in different types of human epithelial cells, and about 10 more that are specific to hair and nails. A single epithelial cell may produce multiple types of keratins, and these copolymerize into a single network (Figure 16-18). Every keratin filament is made up of an equal mixture of type I (acidic) and type II (neutral/basic) keratin chains; these form heterodimers, two of which then join to form the fundamental tetrameric subunit (see Figure 16-16). Cross-linked keratin networks held together by disulfide bonds may survive even the death of their cells, forming tough coverings for animals, as in the outer layer of skin and in hair, nails, claws, and scales. The diversity in keratins is clinically useful in the diagnosis of epithelial cancers (carcinomas), as the particular set of keratins expressed gives an indication of the epithelial tissue in which the cancer originated and thus can help to guide the choice of treatment.
Table 16-1
Major Types of Intermediate Filament Proteins in Vertebrate Cells.

Figure 16-18
Keratin filaments in epithelial cells. Immunofluorescence micrograph of the network of keratin filaments (green) in a sheet of epithelial cells in culture. The filaments in each cell are indirectly connected to those of its neighbors by desmosomes (discussed (more...)
Mutations in keratin genes cause several human genetic diseases. For example, when defective keratins are expressed in the basal cell layer of the epidermis, they produce a disorder called epidermolysis bullosa simplex, in which the skin blisters in response to even very slight mechanical stress, which ruptures the basal cells (Figure 16-19). Other types of blistering diseases, including disorders of the mouth, esophageal lining, and the cornea of the eye, are caused by mutations in the different keratins whose expression is specific to those tissues. All of these maladies are typified by cell rupture as a consequence of mechanical trauma and a disorganization or clumping of the keratin filament cytoskeleton. Many of the specific mutations that cause these diseases alter the ends of the central rod domain, underlining the importance of this particular part of the protein for correct filament assembly.

Figure 16-19
Blistering of the skin caused by a mutant keratin gene. A mutant gene encoding a truncated keratin protein (lacking both the N- and C-terminal domains) was expressed in a transgenic mouse. The defective protein assembles with the normal keratins and thereby (more...)
A second family of intermediate filaments, called neurofilaments, is found in high concentrations along the axons of vertebrate neurons (Figure 16-20). Three types of neurofilament proteins (NF-L, NF-M, NF-H) coassemble in vivo, forming heteropolymers that contain NF-L plus one of the others. The NF-H and NF-M proteins have lengthy C-terminal tail domains that bind to neighboring filaments, generating aligned arrays with a uniform interfilament spacing. During axonal growth, new neurofilament subunits are incorporated all along the axon in a dynamic process that involves the addition of subunits along the filament length, as well as the addition of subunits at the filament ends. After an axon has grown and connected with its target cell, the diameter of the axon may increase as much as fivefold. The level of neurofilament gene expression seems to directly control axonal diameter, which in turn controls how fast electrical signals travel down the axon.

Figure 16-20
Two types of intermediate filaments in cells of the nervous system. (A) Freeze-etch electron microscopic image of neurofilaments in a nerve cell axon, showing the extensive cross-linking through protein cross-bridges—an arrangement believed to (more...)
The neurodegenerative disease amyotrophic lateral sclerosis (ALS, or Lou Gehrig's Disease) is associated with an accumulation and abnormal assembly of neurofilaments in motor neuron cell bodies and in the axon, which may interfere with normal axonal transport. The degeneration of the axons leads to muscle weakness and atrophy, which is usually fatal. The overexpression of human NF-L or NF-H in mice results in mice that have an ALS-like disease.
The vimentin-like filaments are a third family of intermediate filaments. Desmin, a member of this family, is expressed in skeletal, cardiac, and smooth muscle. Mice lacking desmin show normal initial muscle development, but adults have a variety of muscle cell abnormalities, including misaligned muscle fibers.
Thus, intermediate filaments in general seem to serve as the ligaments of the cell, whereas microtubules and actin filaments seem to serve, respectively, as the bones and muscles of the cell. Just as we require our ligaments, bones, and muscles to work together, so all three cytoskeletal filament systems must normally function collectively to give a cell its strength, its shape, and its ability to move.
Filament Polymerization Can Be Altered by Drugs
Because the survival of eucaryotic cells depends on a balanced assembly and disassembly of the highly conserved cytoskeletal filaments formed from actin and tubulin, the two types of filaments are frequent targets for natural toxins. These toxins are produced in self-defense by plants, fungi, or sponges that do not wish to be eaten but cannot run away from predators, and they generally perturb the filament polymerization reaction. The toxin binds tightly to either the filament form or the free subunit form of a polymer, driving the assembly reaction in the direction that favors the form to which the toxin binds. For example, the drug latrunculin, extracted from the sea sponge Latrunculia magnifica, binds to and stabilizes actin monomers; it thereby causes a net depolymerization of actin filaments. In contrast, phalloidin, from the fungus Amanita phalloides (death cap), binds to and stabilizes actin filaments, causing a net increase in actin polymerization. (One remedy for Amanita mushroom poisoning is to eat a large quantity of raw meat: the high concentration of actin filaments in the muscle tissue binds the phalloidin and thereby reduces phalloidin's toxicity.) Either change in actin filaments is very toxic for cells. Similarly, colchicine, from the meadow saffron (or autumn crocus), binds to and stabilizes free tubulin, causing microtubule depolymerization. In contrast, taxol, extracted from the bark of a rare species of yew tree, binds to and stabilizes microtubules, causing a net increase in tubulin polymerization. These and some other natural products that are commonly used by cell biologists to manipulate the cytoskeleton are listed in Table 16-2.
Table 16-2
Drugs That Affect Actin Filaments and Microtubules.
Drugs like these have a rapid and profound effect on the organization of the cytoskeleton in living cells (Figure 16-21). They provided early evidence that the cytoskeleton is a dynamic structure, maintained by a rapid and continual exchange of subunits between the soluble and filamentous forms and that this subunit flux is necessary for normal cytoskeletal function.

Figure 16-21
Effect of the drug taxol on microtubule organization. (A) Molecular structure of taxol. Recently, organic chemists have succeeded in synthesizing this complex molecule, which is widely used for cancer treatment. (B) Immunofluorescence micrograph showing (more...)
The drugs listed in Table 16-2 have been useful to cell biologists trying to probe the roles of actin and microtubules in various cell processes. Some of them are also used in the treatment of cancer. Both microtubule-depolymerizing drugs such as vinblastine and microtubule-polymerizing drugs such as taxol preferentially kill dividing cells, since both microtubule assembly and disassembly are crucial for correct function of the mitotic spindle (discussed in Chapter 18). These drugs efficiently kill certain types of tumor cells in a human patient, although not without toxicity to rapidly dividing normal cells, including those in the bone marrow, intestine, and hair follicles. Taxol in particular has been widely used to treat some specific cancers that are resistant to other chemotherapeutic agents.
The common laboratory reagent acrylamide, used as the precursor in making polyacrylamide gels for size separation of proteins and nucleic acids (see Figure 8-14), is also a cytoskeletal toxin. By an unknown mechanism, it causes the disassembly or rearrangement of intermediate filament networks. Acryl-amide can be absorbed through the skin and acts as a potent neurotoxin by dismantling the neurofilament bundles in peripheral nerve axons. This is why it causes an unpleasant tingling sensation when spilled on bare skin and why it should always be handled cautiously.
Summary
The cytoplasm of eucaryotic cells is spatially organized by a network of protein filaments known as the cytoskeleton. This network contains three principal types of filaments: microtubules, actin filaments, and intermediate filaments. All three types of filaments form as helical assemblies of subunits that self-associate using a combination of end-to-end and side-to-side protein contacts. Differences in the structure of the subunits and the manner of their self-assembly give the filaments different mechanical properties. Intermediate filaments are rope-like and easy to bend but hard to break. Microtubules are strong, rigid hollow tubes. Actin filaments are the thinnest of the three and are hard to stretch but easy to break.
In living cells, all three types of cytoskeletal filaments undergo constant remodeling through the assembly and disassembly of their subunits. Microtubules and actin filaments add and lose subunits only at their ends, with one end (the plus end) growing faster than the other. Tubulin and actin (the subunits of microtubules and actin filaments, respectively) bind and hydrolyze nucleoside triphosphates (tubulin binds GTP and actin binds ATP). Nucleotide hydrolysis underlies the characteristic dynamic behavior of these two filaments. Actin filaments in cells seem to predominantly undergo treadmilling, where a filament assembles at one end while simultaneously disassembling at the other end. Microtubules in cells predominantly display dynamic instability, where a microtubule end undergoes alternating bouts of growth and shrinkage.
Whereas tubulin and actin have been strongly conserved in eucaryotic evolution, the family of intermediate filaments is very diverse. There are a variety of tissue-specific forms, including keratin filaments in epithelial cells, neurofilaments in nerve cells, and desmin filaments in muscle cells. In all these cells, the primary job of intermediate filaments is to provide mechanical strength.
- Each Type of Cytoskeletal Filament Is Constructed from Smaller Protein Subunits
- Filaments Formed from Multiple Protofilaments Have Advantageous Properties
- Nucleation Is the Rate-limiting Step in the Formation of a Cytoskeletal Polymer
- The Tubulin and Actin Subunits Assemble Head-to-Tail, Creating Filaments that Are Polar
- The Two Ends of a Microtubule and of an Actin Filament Are Distinct and Grow at Different Rates
- Filament Treadmilling and Dynamic Instability Are Consequences of Nucleotide Hydrolysis by Tubulin and Actin
- Treadmilling and Dynamic Instability Require Energy but Are Useful
- Other Polymeric Proteins Also Use Nucleotide Hydrolysis to Couple a Conformational Change to Cell Movements
- Tubulin and Actin Have Been Highly Conserved During Eucaryotic Evolution
- Intermediate Filament Structure Depends on The Lateral Bundling and Twisting of Coiled Coils
- Intermediate Filaments Impart Mechanical Stability to Animal Cells
- Filament Polymerization Can Be Altered by Drugs
- Summary
- The Self-Assembly and Dynamic Structure of Cytoskeletal Filaments - Molecular Bi...The Self-Assembly and Dynamic Structure of Cytoskeletal Filaments - Molecular Biology of the Cell
Your browsing activity is empty.
Activity recording is turned off.
See more...