NCBI Bookshelf. A service of the National Library of Medicine, National Institutes of Health.
Breman JG, Alilio MS, White NJ, editors. Defining and Defeating the Intolerable Burden of Malaria III: Progress and Perspectives: Supplement to Volume 77(6) of American Journal of Tropical Medicine and Hygiene. Northbrook (IL): American Society of Tropical Medicine and Hygiene; 2007 Dec.
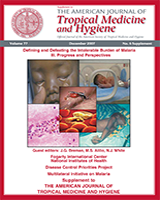
Defining and Defeating the Intolerable Burden of Malaria III: Progress and Perspectives: Supplement to Volume 77(6) of American Journal of Tropical Medicine and Hygiene.
Show detailsMalaria has been the greatest scourge of humankind for many millennia, and as a consequence has had more impact than any other pathogen in shaping the human genome. The sequencing of the human genome provides a new opportunity to determine the genetic traits that confer resistance to infection or disease. The identification of these traits can reveal immune responses, or host–parasite interactions, which may be useful for designing vaccines or new drugs. Similarly, the parasite genome sequence is being exploited to accelerate the development of new antimalarial interventions, for example by identifying parasite metabolic pathways that may be targeted by drugs. The malaria parasites are well known for their ability to undergo antigenic variation, and in parallel to cause a diverse array of disease syndromes, including the severe syndromes that commonly cause death. Genome-based technologies are being harnessed to relate gene and protein expression levels, or genetic variation, to the parasite forms that are targets of protective immunity. Well-conducted clinical studies are required to relate host or parasite diversity with disease. However, genomics studies of human populations raise important ethical issues, such as the disposition of data related to disease susceptibility or paternity, and the ability of communities to understand the nature of the research.
The Many Manifestations of Malaria
Although human malaria results from infection with any of four Plasmodium species, research has primarily concerned itself with P. falciparum malaria, which is the deadliest kind. P. falciparum malaria takes a devastating toll on human life, with estimates of 1 to 3 million deaths annually. The deadly syndromes of malaria can take diverse forms, including severe anemia, respiratory distress, and cerebral malaria. The sequence of events that leads to severe disease and death is incompletely understood.
Understanding the mechanisms of disease will offer important opportunities for intervention, and to a large extent the story of these diseases must have been written into the genomes of the parasite and the host. In some cases, parasite factors underlie the different syndromes, such as the well-known property of parasites causing pregnancy malaria to bind the placental receptor chondroitin sulfate A (CSA).1 Scientists have used the parasite genome to assist with identifying the parasite protein(s) that mediate parasite binding to CSA.2
Looked at from another perspective, the vast majority of malaria infections—500 million cases annually by some counts—do not result in death. By that measure, malaria does not seem especially dangerous because more than 99% of cases do not cause death. Therein lies another tale—one of parasite and host co-adaptation. In part, this story is written into the human genome, which has been selectively shaped by the malaria parasite to a greater extent than any other pathogen.3 Otherwise deleterious genetic traits have been maintained at high frequencies in malaria-exposed populations because of the selective advantage that they confer against the disease.4,5 Understanding how the human genome has been shaped by this experience can offer important insights into host strategies that prevent severe disease and death and whether these are conferred by innate resistance or acquired immunity.
The Genome Promise and Some Questions
The mechanisms of survival for both the parasite and its human host offer insights that can guide the development of malaria vaccines and antimalarial drugs. P. falciparum exhibits great diversity in both its phenotypic and genotypic characteristics on a world-wide scale.6 Parasite diversity has been interpreted as a way for the parasite to escape the immune responses of the infecting human host.7–9 Some properties of parasite-infected erythrocytes (IE) such as rosetting (binding to uninfected erythrocytes) or adhesion to different endothelial receptors have been associated with severe malaria, and are variably expressed by the parasite.
Conversely, the ability to resist P. falciparum malaria is an important adaptation of human populations living in endemic areas.10 The sickle cell trait is the best studied innate mechanism of resistance to malaria. During the past 20 years, polymorphisms in multiple other human genes have been described that affect susceptibility to P. falciparum infections, including additional hemoglobin mutations,11,12 HLA Bw53,13 and Toll-like receptor polymorphisms.14
Unraveling the strands of these two tales—the tale of a lethal parasite and the tale of human survival—may now be possible with the completed sequences of human and Plasmodium falciparum genomes.15,16 These major achievements have opened the door to rapid advancements in the research areas of comparative genomics, transcriptomics, and proteomics. Four years after the publication of the parasite genome, though, are we able to list new drug targets, new malaria vaccine candidates, and new diagnostic tools derived from this field of research?
Many post-genomics studies have been completed, and substantial data have been generated. What are the strategies for turning genome sequences or genomic technologies into malaria intervention or public health tools? What information can be derived from the parasite genome or the human genome, and which genome may contain the information that is necessary for a solution? Finally, how will these new technologies be integrated into clinical studies in tropical countries, and how can scientists in these countries access the tools and best design their research in a post-genomic world?
Mining the Parasite Genome
Barring eradication of either malaria parasites or the mosquitoes that transmit them, the solution to the malaria problem requires effective drugs and vaccines. In recent times, we have been losing the race to stay ahead of the parasite. Deaths have mounted as chloroquine-resistant parasites spread around the world, and resistance to sulfadoxine-pyrimethamine developed soon after it was introduced to replace chloroquine in many areas. Meantime, the promise of a highly effective vaccine has remained elusive, despite some key advances including the partially effective RTS, S vaccine.17
The parasite genome may offer the means to stay ahead in the race against the parasite. Traditional approaches to drug development for malaria have yielded a limited pharmacopeia. Our best drugs have been derived from traditional herbal treatments, for example quinine and artemisinin, or from cumbersome small molecule library and synthetic analogue screens, such as mefloquine. As the few remaining drugs lose their efficacy—and there is worrying evidence that resistance to artemisinins may be emerging in some areas where it has been introduced18—we must find alternative strategies to identify new targets.
The malaria genome, of course, contains many potential drug targets. Genes that are essential for parasite survival are the natural starting point, and those encoding proteins involved in metabolic pathways are the traditional candidates. The genome sequence has greatly extended our understanding of the parasite’s metabolic pathways, and thus revealed numerous targets for drug development. For example, the identification of genes involved in isoprenoid biosynthesis in the falciparum genome led to the identification of fosmidomycin and its derivatives as potential antimalarial drugs.19
Transfection technologies allow scientists to selectively “knockout” individual genes and to determine whether the parasite can survive without them. Notably, some genes are essential to parasite survival during blood stage development, whereas other genes are essential during the liver stage20 or in the sexual stage (the stage that transmits to mosquitoes). Any of these may be a useful drug target. A blood stage target is ideal for treatment of sick persons, whereas a liver stage target may be useful for developing prophylactic agents.
The Parasite Genome and Vaccine Discovery
The completion of the malaria genome sequence also allows a more deliberate approach to vaccine development. Antigens may be selected according to their role in protective immunity. Protective immunity can prevent infection or can prevent disease. Immunity that prevents infection can be achieved by inoculating humans with parasites attenuated by irradiation.21–23 Immunity that prevents disease develops naturally when people are exposed to malaria over an extended period of time. For example, adult residents of areas with stable malaria transmission are able to limit parasitemia and do not often become sick when they are infected with P. falciparum. Passive transfer studies in humans have demonstrated that the IgG fraction of serum contains this protective activity.24,25
New technologies have been developed that capitalize on the available genome sequences. These include DNA microarrays to study gene transcription, proteomics tools to measure protein expression, and bioinformatics approaches that predict protein function and localization based on gene or protein sequences. These tools are high throughput approaches, and in some cases have overcome technical problems that previously stymied laboratory studies of malaria parasites. For these reasons, the genome-based technologies will be extremely useful to identify the malaria antigens that may be involved in models of protective immunity.
Surface proteins are a logical target for vaccines, because they often carry out key functions such as adhesion or invasion, and they are accessible to antibodies. Proteomics tools survey the full repertoire of surface proteins expressed by a pathogen, from which the optimal antigens for a vaccine can be rationally selected. For example, proteomics studies identified novel surface proteins of Streptococcus agalactica and Helicobacter pylori.26,27
In the case of P. falciparum, mass spectrometry (MS) has been used to characterize proteins expressed on the surface of the infected erythrocytes (IE) in fresh samples collected from Tanzanian donors.28 Parasites form electron-dense structures called “knobs” on the IE surface that mediate adhesion to the vascular endothelium. In the study from Tanzania, knob proteins were enriched by differential detergent extraction followed by various fractionation methods prior to liquid chromatography (LC)-MS/MS. This approach identified peptides from hundreds of proteins. Among these, 75% contained a predicted transmembrane (TM) domain, and 70% had no known function. This approach identified variant surface antigens as well as several conserved proteins with predicted TM domains or signal peptides, which were associated with the CSA-binding or placental parasites.
DNA microarrays that measure gene transcription are a useful adjunct to proteomics studies. Although gene transcription levels do not necessarily correspond to protein levels, microarrays (which measure thousands of genes) have greater sensitivity than MS technologies (which measure hundreds of proteins). DNA microarrays have been applied to laboratory isolates to define the entire transcriptome of P. falciparum during intraerythrocytic and mosquito stages.27,29 One approach to malaria antigen discovery entails identifying the genes that are upregulated in virulent or disease-causing parasite forms. For example, upregulated genes identified by microarray analysis of Neisseria meningitidis serogroup B were shown to encode proteins that protect against meningococcus in a mouse model.30,31
For malaria, vaccine developers will be interested in genes that are upregulated in the parasite stages or forms that are the target of protective immunity. The genes expressed by LS parasites encode proteins that are targets of protective immunity and could be used as subunit vaccines. Alternatively, these genes may be essential to liver stage development and therefore could be knocked out to produce genetically attenuated parasites that induce protective immunity but cannot cause blood stage infection and disease.20
Among blood-stage parasites, IEs that bind to the placental receptor CSA have been shown to be commonly involved in malaria of pregnant women. For a vaccine against pregnancy malaria, scientists are using microarrays to identify the subset of genes that are preferentially expressed by CSA-binding or placental parasites.32 The parasite forms that cause the severe malaria syndromes of children remain unknown. However, there is intense interest to identify these parasite forms because the antigens that these parasite forms express may be useful to develop vaccines that prevent disease and death due to malaria in young African children.
Human Genomic Analysis of Malaria Resistance
Given the importance of environment and the likely importance of parasite strain variation in determining the risk of malaria, it may seem at first unlikely that human genetic variation would play a major role. However, the strongest known determinant of severe malaria due to P. falciparum is a human genetic factor, sickle cell trait, which reduces risk by approximately 10-fold.13,33 Host genetic factors are estimated to account for 25% of variation in risk of hospital admission for malaria among Kenyan children, whereas household accounts for only 14% of the variation.34 Risk of malaria infection appears to have a similar contribution from host genetics (24%) and household (29%). Importantly, sickle cell trait accounted for only 2% of the total variation, implying that many other host genetic determinants remain to be discovered.
Although human genetics is now being used as an investigative tool in many diseases, malaria may prove to be the most productive application of this approach because it is the strongest known force for evolutionary selection in the recent history of the human genome. In addition to the HbS allele, which encodes sickle hemoglobin, a remarkable range of genetic variants of erythrocyte proteins have risen to high frequency in human populations due to their protective effect against P. falciparum malaria. They include variants of globin structure (HbS, HbC, HbE), globin regulation (thalassemias), band III protein (ovalocytosis), and glucose-6-phosphate dehydrogenase (reviewed in ref 3). Malaria resistance alleles have served as an important paradigm in the development of statistical methods to uncover signatures of recent positive selection in the human genome.35–38
An elegant demonstration of how genetic discoveries can assist vaccine development is provided by Duffy blood group antigen. Some 30 years ago it was discovered that the reason for the near absence of P. vivax from sub-Saharan Africa is due to the fact that most of the population have a genetic variant that prevents the Duffy blood group antigen being expressed on erythrocytes.39 This genetic discovery led to the molecular discovery of the protein that the parasite uses to bind to Duffy antigen,40 which is essential for erythrocyte invasion by P. vivax and represents an obvious target for a P. vivax vaccine.41
Knowledge of the human genome sequence has revolutionized the investigation of genetic diversity within human populations. Of the 3 billion base pairs in the human genome, in the order of 10 million (i.e., approximately 1 in 300) base pairs are estimated to have polymorphisms present at more than 1% frequency in human populations.38 Armed with this knowledge, the medical research community is investing huge efforts in large-scale epidemiologic studies to uncover the genetic basis of common human diseases. The long-term goal is not just to make genetic discoveries, but also to use these discoveries to dissect the complex web of molecular pathways that underlie the evolution of disease, as well as the molecular mechanisms that protect against disease, and thereby to accelerate the process of vaccine and drug discovery.
How can we most efficiently and reliably discover novel malaria resistance alleles? The most popular approach is candidate gene analysis, in which the investigators begin by selecting genes or gene regions of particular interest, and then type polymorphisms in those genes for association with severe malaria. The advent of PCR-based genotyping methods has led to dozens of candidate gene studies of malaria over the past 15 years or so, many of which have identified putative disease associations (reviewed in ref 3).
However, there are some problems in interpreting this mass of candidate gene data. One problem is that in very few studies have the candidate genes been tested for more than a handful of known polymorphisms, so a negative result may simply mean that important polymorphisms were omitted from the analysis. This problem will eventually be solved as more polymorphisms are discovered, and as new technologies make it possible for investigators to type many polymorphisms at affordable cost. A more serious problem is that many candidate gene studies are underpowered and have not been replicated in independent studies, leading to a high rate of both false negative and false positive results. Population stratification is an important cause of false positives in case-control studies. It arises when the cases and controls are sampled from sub-populations of different ancestry, so that the apparent disease association simply reflects the different genetic makeup of the sub-populations rather than genetic effects on the disease itself. It is thus imperative to match cases and controls by ethnic group, but even with careful matching it may be difficult to avoid subtle stratification effects in ethnically heterogeneous populations.42 Family-based association studies, in which the genotypes of an affected child are compared with those of its parents, provide a way of excluding these population artifacts and represent an important complementary approach to standard case-control analysis.33
The cutting edge of genomic epidemiology goes beyond candidate genes to the whole human genome, which harbors thousands of genes whose function is unknown, some of which may play a critical role in host–parasite interactions. Discovery of novel genes that influence the evolution of disease and of protective immunity could provide vital clues for vaccine development, as illustrated by the example of Duffy antigen given previously. Screening the whole human genome for malaria resistance alleles by genetic association analysis would not have been feasible a few years ago, but 3 recent advances now make this possible. First, the International HapMap Project has surveyed almost 4 million single nucleotide polymorphisms (SNPs) across the genome, and by describing the common combinations of alleles found in individuals of African, Asian, and European origin, provides a rational basis for the identification of SNPs that are informative about common forms of human variation in different populations.38 Second, new high-throughput genotyping technologies allow an individual to be genotyped for over half a million SNPs in a single assay. Finally, the establishment of large, multicenter collaborations such as the Malaria Genomic Epidemiology Network (www.malariagen.net) makes it feasible to achieve the sample sizes required to carry out genome-wide association studies that are adequately powered to correct for the vast number of multiple observations.43 According to current thinking this needs at least 2,000 cases and 2,000 controls, and the formation of large-scale partnerships allows this to be increased by at least 4-fold to detect genetic effects of modest magnitude.
Genome Science in the Field
The broad intention of human and malaria parasite genetic studies is to explain observed differences in malaria disease incidence, prevalence and severity, and to apply accrued knowledge to disease control. These studies involve human subjects by collecting blood specimens and clinical information, and therefore they should meet all Good Clinical Practice principles. Some genetic studies are designed to be nested in ongoing trials or observational studies, which have separately received ethical approval.
However, many of these genomic studies are commonly introduced into the research plan at later stages of the main study. In general, genome-based studies must be performed in well-equipped laboratories, meaning that these are mostly conducted outside the endemic countries in Africa or elsewhere. In these cases, the collection and management of specimens and clinical information raise several ethical issues. How does the study team obtain valid written informed consent for samples meant for future studies? What measures are in place to ensure that samples transferred to another laboratory or country are strictly used for intended and stated investigations?
Practical issues also must be considered. The team must be prepared to manage the cross-border transportation of genetic materials. Thought must be given as well to the nature of the genetic information being collected, and the manner in which this information may be returned to the study participants. Study teams should be particularly aware of unearthing sensitive human genetic findings, such as information on genetic traits associated with disease susceptibility, or family information that may determine paternity of children. Although these ethical issues have no clear cut answers, they should be adequately addressed by the study team in collaboration with local Institutional Review Boards (IRBs) and Ethics Review Committees (ERCs).
Apart from scientists, lay populations are also aware that disease severity varies within communities exposed to similar intensity of transmission and presumably malaria parasites of similar virulence. The awareness of the lay population is a good thing because this will facilitate their understanding of the basis for genomics studies as well as obtaining informed consent for the studies.
Inter-individual variations in disease manifestations under similar epidemiologic settings may be explained in part by variations in the parasite and in part by genetic determinants in the host. Although current knowledge indicates that some polymorphisms within the human genome such as hemoglobinopathies and certain thalassemias confer protection to malaria, the true mechanisms of protection remains unclear. Understanding these mechanisms is wholly dependent on good clinical studies, partnered with strong basic research in genomics, pathogenesis, and immunology.
Conclusions
The genome sequences of the malaria parasite P. falciparum and its human host have created new opportunities to solve the malaria problem. Although the availability of genome sequences and genome-based technologies have already greatly expanded our understanding of both the parasite and the host response to it, the new genomic era has yet to deliver on its promise of effective new drugs and vaccines. The solution to the malaria problem lies in an understanding of both parasite and human genomes, and the insights these offer into the myriad interactions between the parasite and the host. In many cases, the full realization of the genomic promise will require the application of these technologies to populations affected by malaria, meaning that studies designed to meet the highest clinical and ethical standards must be conducted in resource-poor communities. Laboratory and clinical researchers around the globe are ready to embrace the opportunities created by the genome sequences. Most people expect that a wealth of information emanating from these studies will profoundly enhance our understanding of the malaria problem. What remains less clear is where in the parasite or human genomes we may find solutions to the malaria problem, hidden right in front of us. Finding those solutions is the very reason for research.
Acknowledgments: DPK and MD thank their colleagues in the Malaria Genomic Epidemiology Network for collaborations. PED thanks M. Fried for ideas and discussions
Financial support: FN is a member of the Malaria Immunology and Pathogenesis Network (MIMPAC). DPK receives support from the Medical Research Council, Wellcome Trust, Bill and Melinda Gates Foundation and Grand Challenges in Global Health. MD receives support from the International Atomic Energy Authority and the Bill & Melinda Gates Foundation. PED receives support from Bill & Melinda Gates Foundation (Grant 29202), Grand Challenges in Global Health/ Foundation for NIH (Grant 1364), US Department of Defense (Award W81XWH-05-2-0014), the US National Institutes of Health (R01AI52059 and U19AI065664), and the Fogarty International Center/NIH (D43 TW05509-04).
References
- 1.
- Fried M, Duffy PE. Adherence of Plasmodium falciparum to chondroitin sulfate A in the human placenta. Science. 1996;272:1502–1504. [PubMed: 8633247]
- 2.
- Salanti A, Staalsoe T, Lavstsen T, Jensen AT, Sowa MP, Arnot DE, Hviid L, Theander TG. Selective upregulation of a single distinctly structured var gene in chondroitin sulphate A-adhering Plasmodium falciparum involved in pregnancy-associated malaria. Mol Microbiol. 2003;49:179–191. [PubMed: 12823820]
- 3.
- Kwiatkowski DP. How malaria has affected the human genome and what human genetics can teach us about malaria. Am J Hum Genet. 2005;77:171–192. [PMC free article: PMC1224522] [PubMed: 16001361]
- 4.
- Haldane J. The rate of mutation of human genes. Hereditas. 1948;35:267–273.
- 5.
- Nagel RL, Roth EF Jr. Malaria and red cell genetic defects. Blood. 1989;74:1213–1221. [PubMed: 2669996]
- 6.
- Kemp DJ, Cowman AF, Walliker D. Genetic diversity in Plasmodium falciparum. Adv Parasitol. 1990;29:75–149. [PubMed: 2181830]
- 7.
- Bull PC, Pain A, Ndungu FM, Kinyanjui SM, Roberts DJ, Newbold CI, Marsh K. Plasmodium falciparum antigenic variation: relationships between in vivo selection, acquired antibody response, and disease severity. J Infect Dis. 2005;192:1119–1126. [PubMed: 16107968]
- 8.
- Ntoumi F, Flori L, Mayengue PI, Matondo Maya DW, Issifou S, Deloron P, Lell B, Kremsner PG, Rihet P. Influence of carriage of hemoglobin AS and the Fc gamma receptor IIa-R131 allele on levels of immunoglobulin G2 antibodies to Plasmodium falciparum merozoite antigens in Gabonese children. J Infect Dis. 2005;192:1975–1980. [PubMed: 16267770]
- 9.
- Takala SL, Escalante AA, Branch OH, Kariuki S, Biswas S, Chaiyaroj SC, Lal AA. Genetic diversity in the Block 2 region of the merozoite surface protein 1 (MSP-1) of Plasmodium falciparum: additional complexity and selection and convergence in fragment size polymorphism. Infect Genet Evol. 2006;6:417–424. [PMC free article: PMC1853307] [PubMed: 16517218]
- 10.
- Miller LH, Good MF, Milon G. Malaria pathogenesis. Science. 1994;264:1878–1883. [PubMed: 8009217]
- 11.
- Yuthavong Y, Wilairat P. Protection against malaria by thalassaemia and haemoglobin variants. Parasitol Today. 1993;9:241–245. [PubMed: 15463767]
- 12.
- Weatherall DJ, Miller LH, Baruch DI, Marsh K, Doumbo OK, Casals-Pascual C, Roberts DJ. Malaria and the red cell. Hematology (Am Soc Hematol Educ Program). 2002:35–57. [PubMed: 12446418]
- 13.
- Hill AV, Allsopp CE, Kwiatkowski D, Anstey NM, Twumasi P, Rowe PA, Bennett S, Brewster D, McMichael AJ, Greenwood BM. Common west African HLA antigens are associated with protection from severe malaria. Nature. 1991;352:595–600. [PubMed: 1865923]
- 14.
- Mockenhaupt FP, Cramer JP, Hamann L, Stegemann MS, Eckert J, Oh NR, Otchwemah RN, Dietz E, Ehrhardt S, Schroder NW, Bienzle U, Schumann RR. Toll-like receptor (TLR) polymorphisms in African children: common TLR-4 variants predispose to severe malaria. Proc Natl Acad Sci USA. 2006;103:177–182. [PMC free article: PMC1324982] [PubMed: 16371473]
- 15.
- Gardner MJ, Hall N, Fung E, White O, Berriman M, Hyman RW, Carlton JM, Pain A, Nelson KE, Bowman S, Paulsen IT, James K, Eisen JA, Rutherford K, Salzberg SL, Craig A, Kyes S, Chan MS, Nene V, Shallom SJ, Suh B, Peterson J, Angiuoli S, Pertea M, Allen J, Selengut J, Haft D, Mather MW, Vaidya AB, Martin DM, Fairlamb AH, Fraunholz MJ, Roos DS, Ralph SA, McFadden GI, Cummings LM, Subramanian GM, Mungall C, Venter JC, Carucci DJ, Hoffman SL, Newbold C, Davis RW, Fraser CM, Barrell B. Genome sequence of the human malaria parasite Plasmodium falciparum. Nature. 2002;419:498–511. [PMC free article: PMC3836256] [PubMed: 12368864]
- 16.
- Lasonder E, Ishihama Y, Andersen JS, Vermunt AM, Pain A, Sauerwein RW, Eling WM, Hall N, Waters AP, Stunnenberg HG, Mann M. Analysis of the Plasmodium falciparum proteome by high-accuracy mass spectrometry. Nature. 2002;419:537–542. [PubMed: 12368870]
- 17.
- Heppner DG Jr, Kester KE, Ockenhouse CF, Tornieporth N, Ofori O, Lyon JA, Stewart VA, Dubois P, Lanar DE, Krzych U, Moris P, Angov E, Cummings JF, Leach A, Hall BT, Dutta S, Schwenk R, Hillier C, Barbosa A, Ware LA, Nair L, Darko CA, Withers MR, Ogutu B, Polhemus ME, Fukuda M, Pichyangkul S, Gettyacamin M, Diggs C, Soisson L, Milman J, Dubois MC, Garcon N, Tucker K, Wittes J, Plowe CV, Thera MA, Duombo OK, Pau MG, Goudsmit J, Ballou WR, Cohen J. Towards an RTS,S-based, multi-stage, multi-antigen vaccine against falciparum malaria: progress at the Walter Reed Army Institute of Research. Vaccine. 2005;23:2243–2250. [PubMed: 15755604]
- 18.
- Jambou R, Legrand E, Niang M, Khim N, Lim P, Volney B, Ekala MT, Bouchier C, Esterre P, Fandeur T, Mercereau-Puijalon O. Resistance of Plasmodium falciparum field isolates to in-vitro artemether and point mutations of the SERCA-type PfATPase6. Lancet. 2005;366:1960–1963. [PubMed: 16325698]
- 19.
- Jomaa H, Wiesner J, Sanderbrand S, Altincicek B, Weidemeyer C, Hintz M, Turbachova I, Eberl M, Zeidler J, Lichtenthaler HK, Soldati D, Beck E. Inhibitors of the nonmevalonate pathway of isoprenoid biosynthesis as antimalarial drugs. Science. 1999;285:1573–1576. [PubMed: 10477522]
- 20.
- Mueller AK, Labaied M, Kappe SH, Matuschewski K. Genetically modified Plasmodium parasites as a protective experimental malaria vaccine. Nature. 2005;433:164–167. [PubMed: 15580261]
- 21.
- Clyde DF, Most H, McCarthy VC, Vanderberg JP. Immunization of man against sporozite-induced falciparum malaria. Am J Med Sci. 1973;266:169–177. [PubMed: 4583408]
- 22.
- Herrington D, Davis J, Nardin E, Beier M, Cortese J, Eddy H, Losonsky G, Hollingdale M, Sztein M, Levine M, Nussenzweig RS, Clyde D, Edelman R. Successful immunization of humans with irradiated malaria sporozoites: humoral and cellular responses of the protected individuals. Am J Trop Med Hyg. 1991;45:539–547. [PubMed: 1951863]
- 23.
- Nussenzweig RS, Vanderberg J, Most H, Orton C. Protective immunity produced by the injection of x-irradiated sporozoites of plasmodium berghei. Nature. 1967;216:160–162. [PubMed: 6057225]
- 24.
- Cohen S, McGregor IA, Carrington S. Gamma-globulin and acquired immunity to human malaria. Nature. 1961;192:733–737. [PubMed: 13880318]
- 25.
- McGregor IA, Carrington SP. Treatment of East African P. falciparum malaria with west African human γ-globulin. Trans R Soc Trop Med Hyg. 1963;57:170–175.
- 26.
- Hughes MJ, Moore JC, Lane JD, Wilson R, Pribul PK, Younes ZN, Dobson RJ, Everest P, Reason AJ, Redfern JM, Greer FM, Paxton T, Panico M, Morris HR, Feldman RG, Santangelo JD. Identification of major outer surface proteins of Streptococcus agalactiae. Infect Immun. 2002;70:1254–1259. [PMC free article: PMC127763] [PubMed: 11854208]
- 27.
- Larsson T, Bergstrom J, Nilsson C, Karlsson KA. Use of an affinity proteomics approach for the identification of low-abundant bacterial adhesins as applied on the Lewis(b)-binding adhesin of Helicobacter pylori. FEBS Lett. 2000;469:155–158. [PubMed: 10713262]
- 28.
- Fried M, Wendler JP, Mutabingwa TK, Duffy PE. Mass spectrometric analysis of Plasmodium falciparum erythrocyte membrane protein-1 variants expressed by placental malaria parasites. Proteomics. 2004;4:1086–1093. [PubMed: 15048989]
- 29.
- Le Roch KG, Zhou Y, Blair PL, Grainger M, Moch JK, Haynes JD, De La Vega P, Holder AA, Batalov S, Carucci DJ, Winzeler EA. Discovery of gene function by expression profiling of the malaria parasite life cycle. Science. 2003;301:1503–1508. [PubMed: 12893887]
- 30.
- Grifantini R, Bartolini E, Muzzi A, Draghi M, Frigimelica E, Berger J, Ratti G, Petracca R, Galli G, Agnusdei M, Giuliani MM, Santini L, Brunelli B, Tettelin H, Rappuoli R, Randazzo F, Grandi G. Previously unrecognized vaccine candidates against group B meningococcus identified by DNA microarrays. Nat Biotechnol. 2002;20:914–921. [PubMed: 12172557]
- 31.
- Grifantini R, Bartolini E, Muzzi A, Draghi M, Frigimelica E, Berger J, Randazzo F, Grandi G. Gene expression profile in Neisseria meningitidis and Neisseria lactamica upon host-cell contact: from basic research to vaccine development. Ann NY Acad Sci. 2002;975:202–216. [PubMed: 12538166]
- 32.
- Francis SE, Malkov VA, Oleinikor AV, Rossnagle E, Wendler JP, Mutabingwa TK, Fried M, Duffy PE. Six genes are preferentially transcribed by the circulating and sequested forms of Plasmodium falciparum parasites that infect pregnant women. Infect Immun. 2007;75:4838–4850. [PMC free article: PMC2044550] [PubMed: 17698567]
- 33.
- Ackerman H, Usen S, Jallow M, Sisay-Joof F, Pinder M, Kwiatkowski DP. A comparison of case-control and family-based association methods: the example of sickle-cell and malaria. Ann Hum Genet. 2005;69:559–565. [PubMed: 16138914]
- 34.
- Mackinnon MJ, Mwangi TW, Snow RW, Marsh K, Williams TN. Heritability of malaria in Africa. PLoS Med. 2005;2:e340. [PMC free article: PMC1277928] [PubMed: 16259530]
- 35.
- Tishkoff SA, Varkonyi R, Cahinhinan N, Abbes S, Argyropoulos G, Destro-Bisol G, Drousiotou A, Dangerfield B, Lefranc G, Loiselet J, Piro A, Stoneking M, Tagarelli A, Tagarelli G, Touma EH, Williams SM, Clark AG. Haplotype diversity and linkage disequilibrium at human G6PD: recent origin of alleles that confer malarial resistance. Science. 2001;293:455–462. [PubMed: 11423617]
- 36.
- Sabeti PC, Reich DE, Higgins JM, Levine HZ, Richter DJ, Schaffner SF, Gabriel SB, Platko JV, Patterson NJ, McDonald GJ, Ackerman HC, Campbell SJ, Altshuler D, Cooper R, Kwiatkowski D, Ward R, Lander ES. Detecting recent positive selection in the human genome from haplotype structure. Nature. 2002;419:832–837. [PubMed: 12397357]
- 37.
- Ohashi J, Naka I, Patarapotikul J, Hananantachai H, Brittenham G, Looareesuwan S, Clark AG, Tokunaga K. Extended linkage disequilibrium surrounding the hemoglobin E variant due to malarial selection. Am J Hum Genet. 2004;74:1198–1208. [PMC free article: PMC1182083] [PubMed: 15114532]
- 38.
- Altshuler D, Brooks LD, Chakravarti A, Collins FS, Daly MJ, Donnelly P. A haplotype map of the human genome. Nature. 2005;437:1299–1320. [PMC free article: PMC1880871] [PubMed: 16255080]
- 39.
- Miller LH, Mason SJ, Clyde DF, McGinniss MH. The resistance factor to Plasmodium vivax in blacks. The Duffy-blood-group genotype, FyFy. N Engl J Med. 1976;295:302–304. [PubMed: 778616]
- 40.
- Chitnis CE, Miller LH. Identification of the erythrocyte binding domains of Plasmodium vivax and Plasmodium knowlesi proteins involved in erythrocyte invasion. J Exp Med. 1994;180:497–506. [PMC free article: PMC2191600] [PubMed: 8046329]
- 41.
- Yazdani SS, Shakri AR, Pattnaik P, Rizvi MM, Chitnis CE. Improvement in yield and purity of a recombinant malaria vaccine candidate based on the receptor-binding domain of Plasmodium vivax Duffy binding protein by codon optimization. Biotechnol Lett. 2006;28:1109–1114. [PubMed: 16794771]
- 42.
- Marchini J, Cardon LR, Phillips MS, Donnelly P. The effects of human population structure on large genetic association studies. Nat Genet. 2004;36:512–517. [PubMed: 15052271]
- 43.
- Chokshi DA, Parker M, Kwiatkowski DP. Data sharing and intellectual property in a genomic epidemiology network: policies for large-scale research collaboration. Bull World Health Organ. 2006;84:382–387. [PMC free article: PMC2627357] [PubMed: 16710548]
Authors’ addresses: Francine Ntoumi, Hopital Albert Schweitzer, Research Unit, Lambarene B P. Box 118, Gabon, E-mail: gro.ptcde@imuotn. Dominic Kwiatkowski and Mahamadou Diakité, Wellcome Trust Centre for Human Genetics Roosevelt Drive, Oxford OX3 7BN, and Wellcome Trust Sanger Institute, Hinxton, United Kingdom, E-mail: dominic.kwiatkowski@paediatrics .ox.ac.uk. Theonest K. Mutabingwa, MOMS Project, PO Box 476, Morogoro Regional Hospital, Morogoro, Tanzania, E-mail: gro.irbs@awgnibatumt. Patrick E. Duffy, Seattle Biomedical Research Institute,307 Westlake Avenue North, Suite 500, Seattle WA 98109, E-mail: gro.irbs@yffud.kcirtap.
- New Interventions for Malaria: Mining the Human and Parasite Genomes - Defining ...New Interventions for Malaria: Mining the Human and Parasite Genomes - Defining and Defeating the Intolerable Burden of Malaria III: Progress and Perspectives
Your browsing activity is empty.
Activity recording is turned off.
See more...