By agreement with the publisher, this book is accessible by the search feature, but cannot be browsed.
NCBI Bookshelf. A service of the National Library of Medicine, National Institutes of Health.
Purves D, Augustine GJ, Fitzpatrick D, et al., editors. Neuroscience. 2nd edition. Sunderland (MA): Sinauer Associates; 2001.
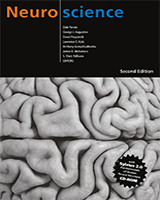
Neuroscience. 2nd edition.
Show detailsUnderstanding the physical structure of ion channels is obviously the key to sorting out how they actually work. For instance, much insight into the detailed operation of ion channels has come from recent X-ray crystallographic studies of a bacterial K+ channel (Figure 4.6). This particular molecule was chosen for analysis because the large quantity of channel protein needed for crystallography could be obtained by growing vast numbers of bacteria. The channel is formed by subunits that each cross the plasma membrane twice; between these two membrane-spanning structures is a loop that inserts into the plasma membrane (Figure 4.6A). Four of these subunits are assembled together to form a channel (Figure 4.6B). In the center of the assembled channel is a narrow opening through the protein that allows K+ to flow across the membrane. The pore, as this tunnel is usually called, is formed by the protein loop, as well as by the membrane-spanning domains. The structure of the pore is well suited for conducting K+ ions (Figure 4.6C). The narrowest part is near the outside mouth of the channel and is so narrow that only a nonhydrated K+ can fit through this bottleneck. Larger cations, such as Cs+, cannot traverse this region of the pore and smaller cations such as Na+ cannot enter the pore because the “walls” of the pore are too far apart to stabilize a dehydrated Na+ ion. This part of the channel complex is thus responsible for the selective permeability to K+ and is therefore called the selectivity filter. Deeper within the channel is a water-filled cavity that connects to the interior of the cell via the pore . This cavity evidently collects K+ from the interior of the cell and, utilizing negative charges from the protein, allows K+ ions to become dehydrated. These “naked” ions are then able to move through the selectivity filter (recall that the normal concentration gradient drives K+ out of cells). In sum, the physical structure of this particular K+ channel provides a detailed picture of how ions are conducted from one side of the plasma membrane to the other, and how a channel can be selectively permeable to K+. It is likely that other K+ channels, as well as other types of ion channels, use similar structures to achieve ion selectivity.

Figure 4.6
Structure of a simple bacterial K+ channel determined by crystallography. (A) Structure of one subunit of the channel, which consists of two membrane-spanning domains and a pore loop that inserts into the membrane. (B) Three-dimensional arrangement of (more...)
Although this bacterial K+ channel nicely illustrates the structural principles responsible for conduction and selectivity in ion channels, the channel does not open or close in response to changes in the membrane potential. Its structure cannot, therefore, indicate much about the mechanisms involved in the voltage gating of ion channels. Understanding this aspect of ion channel function has come from less direct studies of other channel proteins. Although such channels have not yet proven amenable to crystallographic study, much about their ionic selectivity and gating properties has been learned by exploring the functions of particular amino acids within the proteins using mutagenesis and the expression of such channels in Xenopus oocytes. The general transmembrane architecture of all the major ion channel families is consistant with that of the bacterial K+ channel (Figure 4.7). Thus, these molecules are all integral membrane proteins that span the plasma membrane repeatedly. K+ channel subunits typically span the membrane six times (Figure 4.7C,E), though there are some K+ channels that, like the bacterial channel, span the membrane only twice (Figure 4.7D), and others that span the membrane four times (Figure 4.7F) or seven times (Figure 4.7E). Four of these K+ channel subunits aggregate together to form a single functional ion channel. Na+ (and Ca2+) channel subunits cross the membrane 24 times (Figure 4.7A,B), and the channel is evidently formed by just one of these Na+ or Ca2+ channel subunits. Like the bacterial K+ channel, the membrane-spanning domains of all ion channels appear to form a central pore through which ions can diffuse (Figure 4.8). This pore typically arises from the same sort of protein loop that lines the pore of the bacterial channel and has a selectivity filter that determines the permeant ions. As expected, the amino acid composition of the pore loop differs among channels, presumably allowing each channel type to conduct a specific ion. These distinct structural features of channel subunits also provide unique binding sites for drugs and also for various neurotoxins known to block specific subclasses of ion channels (Box C).

Figure 4.7
Topology of the principal subunits of voltage-gated Na+, Ca2+, K+, and Cl- channels. Repeating motifs of Na+ (A) and Ca2+ (B) channels are labeled I, II, III, and IV; (C-F) K+ channels are more diverse. In all cases, four subunits combine to form a functional (more...)

Figure 4.8
A charged voltage sensor permits voltage-dependent gating of ion channels. The process of voltage activation involves the rotation of a positively charged transmembrane domain. This movement causes a change in the conformation of the pore loop, enabling (more...)
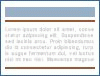
Box C
Toxins That Poison Ion Channels.
A special feature of voltage-activated channels is a sensor that detects the electrical potential across the membrane. This sensor is a transmembrane domain that contains positive charges (see Figure 4.8). The domain is a helical structure with charged amino acids along one face of the helix. Evidently, membrane depolarization influences the charged amino acids such that the helix rotates, thereby allowing the channel pore to open. Similarly, channels that inactivate as a function of membrane voltage also have sequences of amino acids that plug the channel pore during prolonged depolarization.
In short, ion channels are integral membrane proteins with characteristic features that allow them to assemble into multimolecular aggregates. Collectively, these structures allow channels to conduct ions, sense the transmembrane potential, inactivate, and bind to various neurotoxins. Mutations in ion channel genes can have profound effects on channel structure and function, thereby leading to a variety of neurological disorders (Box D).
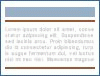
Box D
Diseases Caused by Altered Ion Channels.
- The Molecular Structure of Ion Channels - NeuroscienceThe Molecular Structure of Ion Channels - Neuroscience
Your browsing activity is empty.
Activity recording is turned off.
See more...