By agreement with the publisher, this book is accessible by the search feature, but cannot be browsed.
NCBI Bookshelf. A service of the National Library of Medicine, National Institutes of Health.
Alberts B, Johnson A, Lewis J, et al. Molecular Biology of the Cell. 4th edition. New York: Garland Science; 2002.
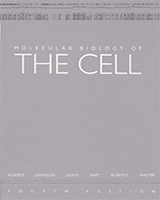
Molecular Biology of the Cell. 4th edition.
Show detailsA central challenge in all areas of cell biology is to understand how the functions of many individual molecular components combine to produce complex cell behaviors. Thus far in this chapter, we have seen how various basic cytoskeletal mechanisms, such as muscle contraction, can be understood in terms of the activities of just a few types of molecules. In this final section, we examine more complex cell behaviors and consider how they are built up from these basic cytoskeletal mechanisms.
The cell behaviors that we describe all rely on coordinated deployment of the same components and processes that we have explored in the first three sections of the chapter: the dynamic assembly and disassembly of cytoskeletal polymers, the regulation and modification of their structure by polymer-associated proteins, and the actions of motor proteins moving along the polymers. How are all these activities coordinated to define a cell's shape, to enable it to crawl, or to divide it neatly into two at mitosis? These problems of cytoskeletal coordination will challenge scientists for many years to come. We shall focus on the control of cell shape and locomotion here, leaving the role of the cytoskeleton in cell division for discussion in Chapter 18.
Mechanisms of Cell Polarization Can Be Readily Analyzed in Yeast Cells
Compared with most animal cells, the budding yeast Saccharomyces cerevisiae has a very simple structure. The most notable feature is the marked asymmetry of the yeast cell, evident in the asymmetrical way it divides by budding to create a small daughter cell and a large mother cell. This asymmetry derives from the polar orientation of its actin cytoskeleton. Because genetic analysis in yeast is relatively easy, it has been possible to use genetic screens to identify many of the molecules involved in generating and maintaining this cell polarity. As in other fields where budding yeast has proved its utility as a model organism for cell biologists, most of these molecules have turned out to have homologs with similar functions in more complex cells.
Budding yeast cells are unusual among eucaryotes in having very few cytoplasmic microtubules, so that most aspects of its polarity depend on actin. There are two types of actin filament assemblies in these cells: actin cables (long bundles of actin filaments) and actin patches (small assemblies of filaments associated with the cell cortex, probably marking sites of endocytosis and exocytosis). Proliferating budding yeast cells must be highly polarized to allow the mother cell to grow a bud from a single site on the cell surface. In this process, the actin patches are highly concentrated at the growing tip of the bud, with the actin cables aligned and pointing toward them. This actin organization presumably directs the secretion of new cell wall material to the site of budding (Figure 16-81).

Figure 16-81
Polarity of actin patches and cables throughout the yeast cell cycle. Filamentous actin structures in the yeast cell, labeled here with fluorescent phalloidin, include actin patches (round bright spots) and actin cables (extended lines). (A) In a mother (more...)
On starvation, yeasts, like many other unicellular organisms, sporulate. But sporulation can occur only in diploid budding yeast cells, whereas budding yeasts mainly proliferate as haploid cells. A starving haploid individual must therefore locate a partner of the opposite mating type, woo it, and mate with it before sporulating. Yeast cells are unable to swim and, instead, reach their mates by polarized growth. The haploid form of budding yeast comes in two mating types, a and α, which secrete mating factors known as a-factor and α-factor, respectively. These secreted signal molecules act by binding to cell-surface receptors that belong to the G-protein-linked receptor superfamily discussed in Chapter 15. One consequence of the binding of α-factor to its receptor is to cause the recipient cell to become polarized, adopting a shape known as a “shmoo” (Figure 16-82). In the presence of an α-factor gradient, the a-cell shmoo tip is directed toward the highest concentration of the signal molecule, which under normal circumstances would direct it toward an amorous α cell located nearby.

Figure 16-82
Morphological polarization of yeast cells in response to mating factor. (A) Cells of Saccharomyces cerevisiae are usually spherical. (B) They become polarized when treated with mating factor from cells of the opposite mating type. The polarized cells (more...)
This polarized cell growth requires alignment of the actin cytoskeleton in response to the mating factor signal. When the signal binds to its receptor, the receptor activates Cdc42, the Rho-family GTPase that is also responsible for some types of actin rearrangements in animal cells (see Figure 16-50D). The signals that the actin cytoskeleton receives from the outside world are then relayed to the microtubule cytoskeleton, causing polarized growth. In addition, the microtubule-organizing center (which in yeast is embedded in the nuclear membrane and is called the spindle pole body) rotates to the side of the nucleus that is closest to the shmoo tip, so that the nucleus becomes oriented along an axis that will guide it to meet and fuse with the nucleus of its mating partner. Extensive genetic studies have identified most of the genes involved in receiving and transducing this spatial signal. As might be expected, many of the proteins that they encode are involved in regulating actin filament and microtubule rearrangements (Figure 16-83).

Figure 16-83
The signaling pathway in the yeast mating factor response. The extracellular mating factor binds to a G-protein-linked receptor in the plasma membrane. Activation of the receptor triggers dissociation of the GTP-bound Gα subunit from a heterotrimeric (more...)
Haploid budding yeast cells use this same polarization machinery during vegetative growth. To form the bud that will grow out to become a daughter cell, the yeast must direct new plasma membrane and cell wall material primarily to a single site. As with shmoo formation, this requires an initial cytoskeletal polarity, with most actin patches in the growing bud and actin cables oriented along the bud axis. In haploid cells, a new bud site is always constructed immediately adjacent to the previous bud site. In this case, the spatial cues that set up cytoskeletal polarity are intrinsic to the cell, left behind from previous rounds of cell division. Cdc42 is once again involved in transducing the signal from the destined bud site to the cytoskeleton, and most of the proteins involved in the upstream and downstream pathways have been identified through genetic experiments. Subsequent to their identification in yeast, many of these proteins have been found to have homologs in other organisms, where they are often likewise involved in the establishment of cell polarity.
Specific RNA Molecules Are Localized by the Cytoskeleton
The cytoskeleton is used to position RNA molecules as well as proteins in cells. The yeast mother and daughter cells retain distinct identities, as revealed by major differences in their subsequent ability to undergo mating-type switching (discussed in Chapter 7) and in the choice of their next bud site. Many of these differences are caused by a gene regulatory protein called Ash1. Both ash1 mRNA and protein are localized exclusively to the growing bud and therefore end up only in the daughter cell. One of the two type V myosins found in yeast, MyoV, is required for this asymmetric distribution of ash1 mRNA. A genetic screen for other mutations that disrupt the mother/daughter difference has revealed that at least six other gene products that are associated with the cytoskeleton are required for normal polarity; these include tropomyosin, profilin, and actin itself, as well as a complex of two proteins that form a direct link between a specific sequence in the ash1 mRNA and the myosin V protein (Figure 16-84).

Figure 16-84
Polarized mRNA localization in the yeast bud tip. (A) The molecular mechanism of ash1 mRNA localization, as determined by genetics and biochemistry. (B) Fluorescent in situ hybridization (FISH) was used to localize the ash1 mRNA (red) in this dividing (more...)
This type of selective positioning of RNA represents yet another way in which the cytoskeleton is involved in cell polarity. For example, in the developing Drosophila embryo (discussed in Chapter 21), a group of mRNAs that encode genes necessary for proper development of the posterior region of the embryo are localized posteriorly by their association with actin filaments, but only after they are transported there by motor proteins moving along oriented microtubules. Identifying the molecules and organizational principles that determine cell polarity in these more complex systems is an active area of research.
Many Cells Can Crawl Across A Solid Substratum
Many cells move by crawling over surfaces rather than by using cilia or flagella to swim. Predatory amoebae crawl continuously in search of food, and they can easily be observed to attack and devour smaller ciliates and flagellates in a drop of pond water. In animals, almost all cell locomotion occurs by crawling, with the notable exception of swimming sperm. During embryogenesis, the structure of an animal is created by the migrations of individual cells to specific target locations and by the coordinated movements of whole epithelial sheets. In vertebrates, neural crest cells are remarkable for their long-distance migrations from their site of origin in the neural tube to a variety of sites throughout the embryo. These cells have diverse fates, becoming skin pigment cells, sensory and sympathetic neurons and glia, and various structures of the face. Long-distance crawling is fundamental to the construction of the entire nervous system: it is in this way that the actin-rich growth cones at the advancing tips of developing axons travel to their eventual synaptic targets, guided by combinations of soluble signals and signals bound to cell surfaces and extracellular matrix along the way.
The adult animal is also seething with crawling cells. Macrophages and neutrophils crawl to sites of infection and engulf foreign invaders as a critical part of the innate immune response. Osteoclasts tunnel into bone, forming channels that are filled in by the osteoblasts that follow after them, in a continuous process of bone remodeling and renewal. Similarly, fibroblasts can migrate through connective tissues, remodeling them where necessary and helping to rebuild damaged structures at sites of injury. In an ordered procession, the cells in the epithelial lining of the intestine travel up the sides of the intestinal villi, replacing absorptive cells lost at the tip of the villus. Cell crawling also has a role in many cancers, when cells in a primary tumor invade neighboring tissues and crawl into blood vessels or lymph vessels and are thereby carried to other sites in the body to form metastases.
Cell crawling is a highly complex integrated process, dependent on the actin-rich cortex beneath the plasma membrane. Three distinct activities are involved: protrusion, in which actin-rich structures are pushed out at the front of the cell; attachment, in which the actin cytoskeleton connects across the plasma membrane to the substratum; and traction, in which the bulk of the trailing cytoplasm is drawn forward (Figure 16-85). In some crawling cells, such as keratocytes from the fish epidermis, these activities are closely coordinated, and the cells seem to glide forward smoothly without changing shape. In other cells, such as fibroblasts, these activities are more independent, and the locomotion is jerky and irregular.

Figure 16-85
A model of how forces generated in the actin-rich cortex move a cell forward. The actin-polymerization-dependent protrusion and firm attachment of a lamellipodium at the leading edge of the cell moves the edge forward (green arrows at front) and stretches (more...)
Plasma Membrane Protrusion Is Driven by Actin Polymerization
The first step in locomotion, protrusion of a leading edge, seems to rely primarily on forces generated by actin polymerization pushing the plasma membrane outward. Different cell types generate different types of protrusive structures, including filopodia (also known as microspikes), lamellipodia, and pseudopodia. All are filled with a dense core of filamentous actin, which excludes membrane-enclosed organelles. The three structures differ primarily in the way in which the actin is organized—in one, two, or three dimensions, respectively—and we have already discussed how this results from the presence of different actin-associated proteins.
Filopodia, formed by migrating growth cones and some types of fibroblasts, are essentially one-dimensional. They contain a core of long, bundled actin filaments, which are reminiscent of those in microvilli but longer and thinner, as well as more dynamic. Lamellipodia, formed by epithelial cells and fibroblasts, as well as by some neurons, are two-dimensional, sheet-like structures. They contain an orthogonally cross-linked mesh of actin filaments, most of which lie in a plane parallel to the solid substratum. Pseudopodia, formed by amoebae and neutrophils, are stubby three-dimensional projections filled with an actin-filament gel. Perhaps because their two-dimensional geometry is most convenient for examination with the light microscope, we know more about the dynamic organization and protrusion mechanism of lamellipodia than we do for either filopodia or pseudopodia.
Lamellipodia contain all of the machinery that is required for cell motility. They have been especially well studied in the epithelial cells of the epidermis of fish and frogs, which are known as “keratocytes” because of their abundant keratin filaments. These cells normally cover the animal by forming an epithelial sheet, and they are specialized to close wounds very rapidly, moving at rates up to 30 μm/min. When cultured as individual cells, keratocytes assume a distinctive shape with a very large lamellipodium and a small, trailing cell body that is not attached to the substratum (Figure 16-86). Fragments of this lamellipodium can be sliced off with a micropipette. Although the fragments generally lack microtubules and membrane-enclosed organelles, they continue to crawl normally, looking like tiny keratocytes (Figure 16-87).

Figure 16-86
Migratory keratocytes from a fish epidermis. (A) Light micrographs of a keratocyte in culture, taken about 15 sec apart. This cell is moving at about 15 μm/sec. (B) Keratocyte seen by scanning electron microscopy, showing its broad, flat lamellipodium (more...)

Figure 16-87
Behavior of lamellipodial fragments. Fragments of the large lamellipodium of a keratocyte can be separated from the main cell, either by surgery with a micropipette or by treating the cell with certain drugs. (A) Many of these fragments continue to move (more...)
The dynamic behavior of actin filaments can be studied in keratocyte lamellipodia by marking a small patch of actin and examining its fate. This reveals that, while the lamellipodia crawl forward, the actin filaments remain stationary with respect to the substrate. The actin filaments in the meshwork are mostly oriented with their plus ends facing forward. The minus ends are frequently attached to the sides of other actin filaments by ARP complexes (see Figure 16-28), helping to form the two-dimensional web (Figure 16-88). The web as a whole seems to be undergoing treadmilling, assembling at the front and dis-assembling at the back, reminiscent of the treadmilling that occurs in individual actin filaments and microtubules discussed previously (see Figure 16-9).

Figure 16-88
Actin filament nucleation and web formation by the ARP complex in lamellipodia. (A) A keratocyte with actin filaments labeled in red by fluorescent phalloidin, and the ARP complex labeled in green with an antibody raised against one of its component proteins. (more...)
Maintenance of unidirectional motion by lamellipodia is thought to require the cooperation and mechanical integration of several factors. Filament nucleation is localized at the leading edge, with new actin filament growth occurring primarily in that location to push the plasma membrane forward. Most filament depolymerization occurs at sites located well behind the leading edge. Because cofilin (see Figure 16-34) binds cooperatively and preferentially to actin filaments containing ADP-actin (the D form), the new T-form filaments generated at the leading edge should be resistant to depolymerization by cofilin (Figure 16-89). As the filaments age and ATP hydrolysis proceeds, cofilin can efficiently disassemble the older filaments. Thus, the delayed ATP hydrolysis by filamentous actin is thought to provide the basis for a mechanism that maintains an efficient, unidirectional treadmilling process in the lamellipodium (Figure 16-90). Finally, bipolar myosin II filaments seem to associate with the actin filaments in the web and pull them into a new orientation—from nearly perpendicular to the leading edge to an orientation almost parallel to the leading edge. This contraction prevents protrusion and it pinches in the sides of the locomoting lamellipodium, helping to gather in the sides of the cell as it moves forward.

Figure 16-89
Cofilin in lamellipodia. (A) A keratocyte with actin filaments labeled in red by fluorescent phalloidin and cofilin labeled in green with a fluorescent antibody. The regions where the two overlap appear yellow. Although the dense actin meshwork reaches (more...)

Figure 16-90
A model for protrusion of the actin meshwork at the leading edge. Two time points during advance of the lamellipodium are illustrated, with newly assembled structures at the later time point shown in a lighter color. Nucleation is mediated by the ARP (more...)
Cell Adhesion and Traction Allow Cells to Pull Themselves Forward
Current evidence suggests that lamellipodia of all cells share this basic, simple type of dynamic organization, but the interactions between the cell and its physical environment usually make the situation considerably more complex than for fish keratocytes crawling on a culture dish. Particularly important in locomotion is the intimate crosstalk between the cytoskeleton and cell adhesion. Although some degree of adhesion to the substratum is necessary for any form of cell crawling, adhesion and locomotion rate seem generally to be inversely related, with highly adhesive cells moving more slowly than weakly adhesive ones. Keratocytes are so weakly adhesive to the substratum that the force of actin polymerization can push the leading edge forward very rapidly. In contrast, when neurons from the sea slug Aplysia are cultured on a sticky substratum, they form large lamellipodia that become stuck too tightly to move forward. In these lamellipodia, the same cycle of localized nucleation of new actin filaments, depolymerization of old filaments, and myosin-dependent contraction continues to operate. But because the leading edge is prevented physically from moving forward, the entire actin mesh moves backward toward the cell body instead (Figure 16-91). The adhesion of most cells lies somewhere between these two extremes, and most lamellipodia exhibit some combination of forward actin filament protrusion (like keratocytes) and rearward actin flux (like the Aplysia neurons).

Figure 16-91
Rearward movement of the actin network in a growth-cone lamellipodium. (A) A growth cone from a neuron of the sea slug Aplysia is cultured on a highly adhesive substratum and viewed by differential-interference-contrast microscopy. Microtubules and membrane-enclosed (more...)
As a lamellipodium, filopodium, or pseudopodium extends forward over a substratum, it can form new attachment sites. These sites can be seen by interference reflection microscopy, as sites where the cell plasma membrane is extremely close to the substratum. In crawling cells, the individual attachment sites, which contain much of the same molecular machinery found in focal contacts (see Figure 16-49), generally form at the cell front and remain stationary as the cell moves forward over them, persisting until the rear of the cell catches up with them. When an individual lamellipodium fails to adhere to the substratum, it is usually lifted up onto the dorsal surface of the cell and rapidly carried backward as a “ruffle” (Figure 16-92).

Figure 16-92
Lamellipodia and ruffles at the leading edge of a human fibroblast migrating in culture. The arrow in this scanning electron micrograph shows the direction of cell movement. As the cell moves forward, lamellipodia that fail to attach to the substratum (more...)
The attachment sites established at the leading edge serve as anchorage points, which allow the cell to generate traction on the substratum and pull its body forward. Traction forces seem to be generated by myosin motor proteins, especially myosin II. In many locomoting cells, myosin II is highly concentrated at the posterior of the cell (Figure 16-93). Dictyostelium amoebae that are deficient in myosin II are able to protrude pseudopodia at normal speeds, but the translocation of their cell body is much slower than that of wild-type amoebae, indicating the importance of myosin II contraction in this part of the cell locomotion cycle. It is not clear how the activity of myosin II pulls the cell body forward. One might imagine that contractile bundles of actin and myosin linking the back of the cell to the front would be responsible; but the observed distribution of myosin II, in some cells at least, suggests a somewhat different strategy, which is depicted in Figure 16-85. Contraction of the actin-rich cortex at the rear of the cell may also selectively weaken the older adhesive interactions that tend to hold the cell back. In addition, myosin II may transport cell body components forward over a polarized array of actin filaments.

Figure 16-93
The localization of myosin I and myosin II in a normal crawling Dictyostelium amoeba. This cell was crawling toward the upper right at the time that it was fixed and labeled with antibodies specific for two myosin isoforms. Myosin I (green) is mainly (more...)
The traction forces generated by locomoting cells exert a significant pull on the substratum (Figure 16-94). In a living animal, most crawling cells move across a semiflexible substratum made of extracellular matrix, which can be deformed and rearranged by these cell forces. In culture, movement of fibro-blasts through a gel of collagen fibrils aligns the collagen, generating an organized extracellular matrix that in turn affects the shape and direction of locomotion of the fibroblasts within it (Figure 16-95). This two-way mechanical interaction between cells and their physical environment is thought to be a major way that vertebrate tissues organize themselves.

Figure 16-94
Adhesive cells exert traction forces on the substratum. These fibroblasts have been cultured on a very thin sheet of silicon rubber. Attachment of the cells, followed by contraction of their cytoskeleton, has caused the rubber substratum to wrinkle. (From (more...)

Figure 16-95
Shaping of the extracellular matrix by cell pulling. This micrograph shows a region between two pieces of embryonic chick heart (tissue explants rich in fibroblasts and heart muscle cells) that were grown in culture on a collagen gel for 4 days. A dense (more...)
External Signals Can Dictate the Direction of Cell Migration
Cell locomotion requires an initial polarization of the cell to set it off in a particular direction. Carefully controlled cell polarization processes are also required for oriented cell divisions in tissues and for formation of a coherent, organized multicellular structure. The molecular mechanisms that generate cell polarity are only beginning to be understood for vertebrates. Most of what we know so far about the topic has been learned through genetic studies in yeasts, flies, and worms. In all known cases, however, the cytoskeleton has a central role, and many of the molecular components have been evolutionarily conserved.
Polarized cell movement is of little use unless it is responsive to cues in the environment, and these can be both chemical and physical. Chemotaxis is defined as cell movement in a direction controlled by a gradient of a diffusible chemical. One well-studied example is the chemotactic movement of a class of white blood cells, called neutrophils, toward a source of bacterial infection. Receptor proteins on the surface of neutrophils enable them to detect the very low concentrations of the N-formylated peptides that are derived from bacterial proteins (only procaryotes begin protein synthesis with N-formylmethionine). Using these receptors, neutrophils are guided to bacterial targets by their ability to detect a difference of only 1% in the concentration of these diffusible peptides on one side of the cell versus the other (Figure 16-96). Both in this case and in the similar chemotaxis of Dictyostelium amoebae toward a source of cyclic AMP, a local polymerization of actin near the receptors is stimulated when the receptors bind their ligands. This actin polymerization response depends on the monomeric Rho-family GTPases discussed earlier. As in the shmooing yeast (see Figure 16-83), the responding cell extends a protrusion toward the signal. The preferential localization of protrusive activity toward one side of the cell indirectly causes reorientation of the traction-generating machinery, and the cell body then follows its “nose” and moves toward the attractive signal.

Figure 16-96
Neutrophil polarization and chemotaxis. The pipette tip at the right is leaking a small amount of the peptide formyl-Met-Leu-Phe. Only bacterial proteins have formylated methionine residues, so the human neutrophil recognizes this peptide as the product (more...)
The direction of cell migration can also be influenced by nondiffusible chemical cues attached to the extracellular matrix or to the surface of cells. Receptor activation by these signals can cause increased cell adhesion, in addition to directed actin polymerization. Most long-distance cell migrations in animals, including neural-crest-cell migration and the travels of neuronal growth cones, depend on a combination of diffusible and non-diffusible signals to steer the locomoting cells or growth cones to their proper destinations (see Figure 21-98).
To help organize persistent movement in a particular direction cells use their microtubules. In many locomoting cells, the position of the centrosome is influenced by the location of protrusive actin polymerization, being found on the forward side of the nucleus. The mechanism of centrosome reorientation is not clear. It is thought that the activation of receptors on one edge of a cell might not only stimulate actin polymerization there (and therefore local protrusion) but also locally activate dynein-like motor proteins that move the centrosome by pulling on its microtubules. The centrosome nucleates a large number of dynamic microtubules, and its repositioning means that many of these have their plus ends extending from the centrosome into the protrusive region of the cell. The dynamic microtubule plus ends may indirectly modulate local adhesion and also activate the Rac GTPase to further increase actin polymerization in the protrusive region. The increased concentration of microtubules would thereby encourage further protrusion, creating a positive feedback loop that enables protrusive motility to persist in the same direction for a prolonged period. Regardless of the exact mechanism, the orientation of the centrosome seems to reinforce the polarity information that the actin cytoskeleton receives from the outside world, allowing a sensitive response to weak signals.
A similar cooperative feedback loop seems to operate in other instances of cell polarization. A particularly interesting example is the killing of specific target cells by cytotoxic T lymphocytes. These cells kill other cells that carry foreign antigens on their surface and are a critical component of the vertebrate's adaptive immune response to infection. When the receptors on the surface of the T cell recognize antigen on the surface of the target cell, Rho-family GTPases are activated and cause actin polymerization under the zone of contact between the two cells, creating a specialized region of the cortex. This specialized site causes the centrosome to reorient, moving with its microtubules to the zone of T-cell-target contact (Figure 16-97). The microtubules, in turn, position the Golgi apparatus right under the contact zone, focusing the killing machinery onto the target cell. The mechanism of killing is discussed in Chapter 24.

Figure 16-97
The polarization of a cytotoxic T cell after target-cell recognition. (A) Changes in the cytoskeleton of a cytotoxic T cell after it has made contact with a target cell. The initial recognition event results in signals that cause actin polymerization (more...)
The Complex Morphological Specialization of Neurons Depends on The Cytoskeleton
Many of the most striking differences between eucaryotic and prokaryotic cells are consequences of the eucaryotic cytoskeleton. The regulated, directed transport of intracellular components by motor proteins enables the eucaryotic cell to enlarge beyond the limits set by the speed of diffusion, which restrict procaryotic cell growth. At the same time, the cytoskeleton provides the framework for the elaborate architectures so characteristic of eucaryotic cells (Figure 1-27; Panel 16-1). No cell exemplifies these two features of size and architectural complexity better than a neuron.
Neurons begin life in the embryo as unremarkable cells, which use actin-based motility to migrate to specific locations. Once there, however, they send out a series of long specialized processes that will either receive electrical signals (dendrites) or transmit these electrical signals to their target cells (axons). Both types of processes (collectively called neurites) are filled with bundles of microtubules, which are critical to both their structure and their function.
In axons, all the microtubules are oriented in the same direction, with their minus end pointing back toward the cell body and their plus end pointing forward toward the axon terminals (Figure 16-98). The microtubules do not reach from the cell body all the way to the axon terminals; each is typically only a few micrometers in length, but large numbers are staggered in an overlapping array. This set of perfectly aligned microtubule tracks acts as a highway to transport many specific proteins and protein-containing vesicles. These are needed at the axon terminals, where synapses must be constructed and maintained, but they are made only in the cell body and dendrites where the cell keeps all its ribosomes. The longest axon in the human body reaches from the base of the spinal cord to the tip of the big toe, being up to a meter in length. Mitochondria, large numbers of specific proteins in transport vesicles, and synaptic vesicle precursors make the long journey in the forward (anterograde) direction. They are carried there by plus-end-directed kinesin-family motor proteins that can move them a meter in about a day, which is a great improvement over diffusion, which would take approximately 8 years to move a mitochondrion this distance. Many members of the kinesin superfamily contribute to this anterograde axonal transport, most carrying specific subsets of membrane-enclosed organelles along the microtubules. The great diversity of the kinesin family motor proteins used in axonal transport suggests that they are involved in specific targeting, as well as in movement. At the same time, old components from the axon terminals are carried back to the cell body for degradation and recycling; this retrograde axonal transport occurs along the same set of oriented microtubules, and it relies on cytoplasmic dynein, which is a minus-end-directed motor protein.

Figure 16-98
Microtubule organization in fibroblasts and neurons. (A) In a fibroblast, microtubules emanate outward from the centrosome in the middle of the cell. Vesicles with plus-end-directed kinesin attached move outward, and vesicles with minus-end-directed dynein (more...)
Axonal structure depends on these microtubules, as well as on the contributions of the other two major cytoskeletal systems—actin filaments and intermediate filaments. Actin filaments line the cortex of the axon, just beneath the plasma membrane, and actin-based motor proteins such as myosin V are also abundant in the axon, perhaps to help move materials, although their exact function is still unclear. Neurofilaments, the specialized intermediate filaments of nerve cells, provide the most important structural support in the axon. A disruption in neurofilament structure, or in the cross-linking proteins that attach the neurofilaments to the microtubules and actin filaments distributed along the axon, can result in axonal disorganization and eventually axonal degeneration.
The construction of the elaborate branching architecture of the neuron during development depends on actin-based motility. As mentioned earlier, the tips of growing axons and dendrites extend by means of a growth cone, a specialized motile structure rich in actin (Figure 16-99). Most neuronal growth cones produce filopodia, and some make lamellipodia as well. The protrusion and stabilization of growth-cone filopodia are exquisitely sensitive to environmental cues. Some cells secrete soluble proteins such as netrin to attract or repel growth cones. In addition, there are fixed guidance markers along the way, attached to the extracellular matrix or to the surfaces of cells. When a filopodium encounters such a “guidepost” in its exploration, it quickly forms adhesive contacts. It is thought that a myosin-dependent collapse of the actin meshwork in the unstabilized part of the growth cone then causes the developing axon to turn toward the guidepost. Other extracellular directional signals act as repellents and cause growth cone collapse at the site of signal action. A complex combination of positive and negative signals, both soluble and insoluble, accurately guide the growth cone to its final destination (discussed in Chapter 21).

Figure 16-99
Neuronal growth cones. (A) Scanning electron micrograph of two growth cones at the end of a neurite, put out by a chick sympathetic neuron in culture. Here, a previously single growth cone has recently split into two. Note the many filopodia and the large (more...)
Microtubules reinforce the directional decisions made by the actin-rich protrusive structures at the leading edge of the growth cone. Microtubules from the axonal parallel array just behind the growth cone are constantly growing into the growth cone and shrinking back by dynamic instability. Adhesive guidance signals are somehow relayed to the dynamic microtubule ends, so that microtubules growing in the correct direction are stabilized against disassembly. In this way, a microtubule-rich axon is left behind, marking the path that the growth cone has traveled.
Dendrites are generally much shorter projections than axons, and they function to receive signals rather than to send them. The microtubules in dendrites all lie parallel to one another but their polarities are mixed, with some pointing their plus ends toward the dendrite tip, while others point back toward the cell body. Nevertheless, dendrites also form as the result of growth-cone activity (see Figure 21-97). Travelling along their complex paths, the growth cones at the tips of both axons and dendrites create the intricate and highly individual morphology of each mature neuronal cell (Figure 16-100). In this way, the cytoskeleton provides the engine for construction of the entire nervous system, as well as the supporting structures that strengthen, stabilize, and maintain its parts.

Figure 16-100
The complex architecture of a vertebrate neuron. The neuron shown is from the retina of a monkey. The arrows indicate the direction of travel of the electrical signal along the axon. The longest and largest neurons in the human body extend for a distance (more...)
Summary
Whole-cell movements and the large-scale shaping and structuring of cells require the coordinated activities of all three basic filament systems along with a large variety of cytoskeletal accessory proteins, including motor proteins. Cell crawling—a widespread behavior important in embryonic development and also in wound healing, tissue maintenance, and immune system function in the adult animal—is a prime example of such complex coordinated cytoskeletal action. For a cell to crawl, it must generate and maintain an overall structural polarity, which is influenced by external cues. Interactions between the microtubule and actin cytoskeletons reinforce this polarity. In addition, the cell must coordinate protrusion at the leading edge (by assembly of new actin filaments), adhesion of the newly protruded part of the cell to the substratum, traction via molecular motors to bring the cell body forward, and disassembly of old cell-substratum contacts.
Nowhere is the elaborate potential of eucaryotic cell morphology better illustrated than in the neuron. Neurons may be many millimeters long, with exceedingly complex dendrites and axons. The elaboration and maintenance of these beautiful and astonishingly complex cells requires the coordinated assembly of microtubules, neurofilaments, and actin filaments, as well as the actions of dozens of highly specialized molecular motors to transport subcellular components to their required destinations.
At the other end of the complexity spectrum, the budding yeast Saccharomyces cerevisiae uses its cytoskeletal plasticity to change shape during vegetative growth and during mating. Through genetic analyses, many of the molecular components that are required for these processes were first identified in yeast. Quite surprisingly, it has turned out that most of the same proteins also mediate the polarity and movements of animal cells, including those of humans.
- Mechanisms of Cell Polarization Can Be Readily Analyzed in Yeast Cells
- Specific RNA Molecules Are Localized by the Cytoskeleton
- Many Cells Can Crawl Across A Solid Substratum
- Plasma Membrane Protrusion Is Driven by Actin Polymerization
- Cell Adhesion and Traction Allow Cells to Pull Themselves Forward
- External Signals Can Dictate the Direction of Cell Migration
- The Complex Morphological Specialization of Neurons Depends on The Cytoskeleton
- Summary
- The Cytoskeleton and Cell Behavior - Molecular Biology of the CellThe Cytoskeleton and Cell Behavior - Molecular Biology of the Cell
- How Cells Regulate Their Cytoskeletal Filaments - Molecular Biology of the CellHow Cells Regulate Their Cytoskeletal Filaments - Molecular Biology of the Cell
- rfx4 regulatory factor X, 4 [Danio rerio]rfx4 regulatory factor X, 4 [Danio rerio]Gene ID:403016Gene
Your browsing activity is empty.
Activity recording is turned off.
See more...