By agreement with the publisher, this book is accessible by the search feature, but cannot be browsed.
NCBI Bookshelf. A service of the National Library of Medicine, National Institutes of Health.
Alberts B, Johnson A, Lewis J, et al. Molecular Biology of the Cell. 4th edition. New York: Garland Science; 2002.
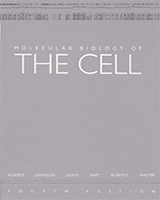
Molecular Biology of the Cell. 4th edition.
Show detailsMicrotubules, actin filaments, and intermediate filaments are much more dynamic in cells than they are in the test tube. The cell regulates the length and stability of its cytoskeletal filaments, as well as their number and the geometry. It does so largely by regulating their attachments to one another and to other components of the cell, so that the filaments can form a wide variety of higher-order structures. Some filament properties are regulated by direct covalent modification of the filament subunits, but most of the regulation is performed by accessory proteins that bind to either the filaments or their free subunits.
This section focuses on how these accessory proteins modify the dynamics and structure of cytoskeletal filaments. We begin with a discussion of how microtubules and actin filaments are nucleated in cells, as this plays a major part in determining the overall organization of the cell's interior.
Microtubules Are Nucleated by a Protein Complex Containing γ-tubulin
While α- and β-tubulins are the regular building blocks of microtubules, another type of tubulin, called γ-tubulin, has a more specialized role. Present in much smaller amounts than α- and β-tubulin, this protein is involved in the nucleation of microtubule growth in organisms ranging from yeasts to humans. Microtubules are generally nucleated from a specific intracellular location known as a microtubule-organizing center (MTOC). Antibodies against γ-tubulin stain the MTOC in virtually all species and cell types thus far examined.
Microtubules are nucleated at their minus end, with the plus end growing outward from each MTOC to create various types of microtubule arrays. A γ-tubulin ring complex (γ-TuRC) has been isolated from both insect and vertebrate cells and is an impressively efficient nucleator of microtubule growth in a test tube. Two proteins, conserved from yeasts to humans, bind directly to the γ-tubulin, along with several other proteins that help create the ring that can be seen at the minus ends of the microtubules nucleated by γ-TuRC. This ring of γ-tubulin molecules is therefore thought to serve as a template that nucleates a microtubule with 13 protofilaments (Figure 16-22).

Figure 16-22
Polymerization of tubulin nucleated by γ-tubulin ring complexes. (A) Model for the nucleation of microtubule growth by the γ-TuRC. The red outline indicates a pair of proteins bound to two molecules of γ-tubulin; this group can (more...)
Microtubules Emanate from the Centrosome in Animal Cells
In most animal cells, there is a single, well-defined MTOC called the centrosome, located near the nucleus. From this focal point, the cytoplasmic microtubules emanate in a star-like, “astral” conformation. Microtubules are nucleated at the centrosome at their minus ends, so the plus ends point outward and grow toward the cell periphery. A centrosome is composed of a fibrous centrosome matrix that contains more than fifty copies of γ-TuRC. Most of the proteins that form this matrix, remain to be discovered, and it is not yet known how they recruit and activate the γ-TuRC.
Embedded in the centrosome is a pair of cylindrical structures arranged at right angles to each other in an L-shaped configuration (Figure 16-23). These are the centrioles, which become the basal bodies of cilia and flagella in motile cells (described later). The centrioles organize the centrosome matrix (also called the pericentriolar material), ensuring its duplication during each cell cycle as the centrioles themselves duplicate. As described in Chapter 18, the centrosome duplicates and splits into two equal parts during interphase, each half containing a duplicated centriole pair (see Figure 18-6). These two daughter centrosomes move to opposite sides of the nucleus when mitosis begins, and they form the two poles of the mitotic spindle (see Figure 18-7). A centriole consists of a short cylinder of modified microtubules, plus a large number of accessory proteins (Figure 16-24). The molecular basis for its duplication is not known.

Figure 16-23
The centrosome. (A) The centrosome is the major MTOC of animal cells. Located in the cytoplasm next to the nucleus, it consists of an amorphous matrix of protein containing the γ-tubulin ring complexes that nucleate microtubule growth. This matrix (more...)

Figure 16-24
A centriole in the centrosome. An electron micrograph of a thick section of a centrosome showing an end-on view of a centriole. The ring of modified microtubules of the centriole is visible, surrounded by the fibrous centrosome matrix. (Courtesy of P. (more...)
In fungi and diatoms, microtubules are nucleated at an MTOC that is embedded in the nuclear envelope as a small plaque called the spindle pole body. Higher-plant cells seem to nucleate microtubules at sites distributed all around the nuclear envelope. Neither fungi nor most plant cells contain centrioles. Despite these differences, all these cells contain γ-tubulin and seem to use it to nucleate their microtubules.
In animal cells, the astral configuration of microtubules is very robust, with dynamic plus ends pointing outward toward the cell periphery and stable minus ends collected near the nucleus. The system of microtubules radiating from the centrosome acts as a device to survey the outlying regions of the cell and position the centrosome at its center, and it does this even in artificial enclosures (Figure 16-25). Even in an isolated cell fragment lacking the centrosome, dynamic microtubules interacting with membraneous organelles and motor proteins arrange themselves into a star-shaped array with the microtubule minus ends clustered at the center (Figure 16-26). This ability of the microtubule cytoskeleton to find the center of the cell establishes a general coordinate system, which is then used to position many organelles within the cell.

Figure 16-25
The center-seeking behavior of a centrosome. (A) Small square wells were micromachined into a plastic substrate. A single centrosome was placed into one of these wells, along with tubulin subunits in solution. As the microtubules polymerize, nucleated (more...)

Figure 16-26
A microtubule array can find the center of a cell. After the arm of a fish pigment cell is cut off with a needle, the microtubules in the detached cell fragment reorganize so that their minus ends end up near the center of the fragment, buried in a new (more...)
Actin Filaments Are Often Nucleated at the Plasma Membrane
In contrast to microtubule nucleation, which occurs primarily deep within the cytoplasm near the nucleus, actin filament nucleation most frequently occurs at the plasma membrane (Figure 16-27). Consequently, the highest density of actin filaments in most cells is at the cell periphery. These actin filaments in the layer underlying the plasma membrane, called the cell cortex, determine the shape and movement of the cell surface. For example, depending on their attachments to one another and to the plasma membrane, actin structures can form many strikingly different types of cell surface projections. These include spiky bundles such as microvilli or filopodia, flat protrusive veils called lamellipodia that help move cells over solid substrates, and the phagocytic cups in macrophages.

Figure 16-27
The tip of the leading edge of a cell nucleates actin filaments. Fibroblasts in culture were gently permeabilized using a nonionic detergent and were then incubated with rhodamine-labeled actin molecules to visualize newly formed actin filaments (red). (more...)
The nucleation of actin filaments at the plasma membrane is frequently regulated by external signals, allowing the cell to change its shape and stiffness rapidly in response to changes in its external environment. This nucleation is catalyzed by a complex of proteins that includes two actin-related proteins, or ARPs, each of which is about 45% identical to actin. Analogous to the function of the γ-TuRC, the ARP complex (also known as the Arp 2/3 complex) nucleates actin filament growth from the minus end, allowing rapid elongation at the plus end. However, the complex can also attach to the side of another actin filament while remaining bound to the minus end of the filament that it has nucleated, thereby building individual filaments into a treelike web (Figure 16-28). The ARP complex is localized in regions of rapid actin filament growth such as lamellipodia, and its nucleating activity is regulated by intracellular signaling molecules and components at the cytosolic face of the plasma membrane.

Figure 16-28
Nucleation and actin web formation by the ARP complex. (A)The structures of Arp2 and Arp3, compared to the structure of actin. Although the face of the molecule equivalent to the plus end (top) in both Arp2 and Arp3 is very similar to the plus end of (more...)
Both γ-tubulin and ARPs are evolutionarily ancient, and they are conserved among a wide variety of eucaryotic species. Their genes seem to have arisen by early duplication of the gene for the microtubule or actin filament subunit, respectively, followed by divergence and specialization of the gene copies so that they encode proteins with a special nucleating function. That a similar strategy has evolved for two separate cytoskeletal systems underlines the central importance of regulated filament nucleation as a general organizing principle in cells.
Filament Elongation Is Modified by Proteins That Bind to the Free Subunits
Once cytoskeletal filaments have been nucleated, they generally elongate by the addition of soluble subunits. In most nonmuscle vertebrate cells, approximately 50% of the actin is in filaments and 50% is soluble. The soluble monomer concentration is typically 50–200 μM (2–8 mg/ml), which is surprisingly high, given the low critical concentration observed for pure actin in a test tube (less than 1 μM). Why does the soluble actin not polymerize into filaments? The reason is that the abundant subunit pool contains special proteins that bind to the actin monomers, thereby making polymerization much less favorable (the action is similar to that of latrunculin). A small protein called thymosin is the most abundant of these proteins. Actin monomers bound to thymosin are in a locked state, where they cannot associate with either the plus or minus ends of actin filament and cannot hydrolyze or exchange their bound nucleotide.
How do cells recruit actin monomers from this sequestered pool and use them for polymerization? One might imagine that the thymosin itself could be regulated by signal transduction pathways, but this has not been found to be the case. Instead, recruitment depends on another monomer-binding protein, profilin. Profilin binds to the face of the actin monomer opposite the ATP-binding cleft, blocking the side of the monomer that would normally associate with the filament minus end (Figure 16-29). However, the profilin-actin complex can readily add onto a free plus end. As soon as this addition occurs, a conformational change is induced in the actin that reduces its affinity for profilin, so the profilin falls off, leaving the actin filament one subunit longer. Profilin competes with thymosin in binding to individual actin monomers, and a local activation of profilin molecules moves actin subunits from the sequestered thymosin-bound pool onto filament plus ends (Figure 16-30).

Figure 16-29
Profilin bound to an actin monomer. The profilin protein molecule is shown in blue, and the actin in red. ATP is shown in green. Profilin binds to the face of actin opposite the ATP-binding cleft. This profilin-actin heterodimer can therefore bind to (more...)

Figure 16-30
Effects of thymosin and profilin on actin polymerization. An actin monomer bound to thymosin is sterically prevented from binding to and elongating the plus end of an actin filament. An actin monomer bound to profilin, on the other hand, is capable of (more...)
Several types of intracellular mechanisms regulate the activity of profilin, including profilin phosphorylation and profilin binding to inositol phospholipids. These mechanisms can define the sites where profilin acts. Profilin's ability to move sequestered actin subunits onto the growing ends of filaments is critical for filament assembly at the plasma membrane, for example. Profilin is localized at the cytosolic face of the plasma membrane because it binds to acidic membrane phospholipids there. At this location, extracellular signals can produce explosive local actin polymerization and the extension of actin-rich motile structures such as filopodia and lamellipodia (see below). Besides binding to actin and phospholipids, profilin also binds to various other intracellular proteins that have domains rich in proline; these proteins may also help to localize profilin to sites where rapid actin assembly may be required.
Like actin monomers, unpolymerized tubulin subunits are sequestered in the cell to maintain the subunit pool at a level substantially higher than the critical concentration. One molecule of the small protein stathmin binds to two tubulin heterodimers and prevents their addition onto the ends of microtubules. Stathmin thus decreases the effective concentration of tubulin subunits that are available for polymerization (the action is analogous to that of colchicine). High levels of active stathmin in a cell decrease the elongation rate of microtubules, since the elongation rate is just the product of the concentration of available tubulin subunits and the rate constant kon. The slower elongation rate also has a second remarkable effect. Since the transition from the growing state to the shrinking state for a microtubule undergoing dynamic instability depends on the race between GTP hydrolysis and filament elongation, slowing the elongation rate by sequestering tubulin subunits can increase the frequency of microtubule shrinkage. Thus, a protein that inhibits tubulin polymerization can have the secondary effect of dramatically increasing the dynamic turnover of microtubules in living cells (Figure 16-31).

Figure 16-31
Effects of stathmin on microtubule polymerization. Polymerization of free tubulin subunits to form microtubules is an energetically favorable reaction. As long as abundant free tubulin subunits are available, microtubule elongation continues. Stathmin (more...)
Stathmin's binding to tubulin is inhibited by the phosphorylation of stathmin, and signals that result in stathmin phosphorylation can increase the rate of microtubule elongation and suppress dynamic instability.
Proteins That Bind Along the Sides of Filaments Can Either Stabilize or Destabilize Them
Once a cytoskeletal filament is formed by nucleation and elongated from the subunit pool, its stability and mechanical properties are often altered by a set of proteins that bind along the sides of the polymer. Different filament-associated proteins use their binding energy to either lower or raise the free energy of the polymer state, and they thereby either stabilize or destabilize the polymer, respectively.
Proteins that bind along the sides of microtubules are collectively called microtubule-associated proteins, or MAPs. Like the drug taxol, MAPs can stabilize microtubules against disassembly. A subset of MAPs can also mediate the interaction of microtubules with other cellular components. This subset is prominent in neurons, where stabilized microtubule bundles form the core of the axons and dendrites that extend from the cell body (Figure 16-32). These MAPs have at least one domain that binds to the microtubule surface and another that projects outward. The length of the projecting domain can determine how closely MAP-coated microtubules pack together, as demonstrated in cells engineered to overproduce different MAPs. Cells overexpressing MAP2, which has a long projecting domain, form bundles of stable microtubules that are kept widely spaced, while cells overexpressing tau, a MAP with a much shorter projecting domain, form bundles of more closely packed microtubules (Figure 16-33).

Figure 16-32
Localization of MAPs in axon and dendrites of a neuron. This immunofluorescence micrograph shows the distribution of tau staining (green) and MAP2 staining (orange) in a hippocampal neuron in culture. Whereas tau staining is confined to the axon (long (more...)

Figure 16-33
Organization of microtubule bundles by MAPs. (A) MAP2 binds along the microtubule lattice at one end and extends a long projecting arm with a second microtubule-binding domain at the other end. (B) Tau binds to the microtubule lattice at both its N- and (more...)
The microtubule-binding domain of several MAPs, including tau and MAP2, includes multiple copies of a tubulin-binding motif. When such MAPs are added to a solution of pure unpolymerized tubulin, they greatly accelerate nucleation, presumably because they stabilize the small tubulin oligomers that form early in polymerization. MAPs are the targets of several protein kinases, and the resulting phosphorylation of a MAP can have a primary role in controlling both its activity and localization inside cells.
Whereas MAP2 and tau are confined to selected cell types in vertebrates, there are other MAPs that seem to have a central role in microtubule dynamics in nearly all eucaryotic cells. In particular, a ubiquitous protein called XMAP215 has close homologs in organisms that range from yeast to humans (XMAP stands for Xenopus microtubule-associated protein, and the number refers to its molecular weight). This protein binds along the sides of microtubules, but, as discussed later, it also has a special ability to stabilize free microtubule ends and inhibit their switch from a growing to a shrinking state. The phosphorylation of XMAP215 during mitosis inhibits this activity, making a substantial contribution to the tenfold increase in the dynamic instability of microtubules observed during mitosis (see Figure 18-12).
Actin filaments are likewise strongly affected by the binding of accessory proteins along their sides. Selected actin filaments in most cells are stabilized by the binding of tropomyosin, an elongated protein that binds simultaneously to seven adjacent actin subunits in one protofilament. The binding of tropomyosin along an actin filament can prevent the filament from interacting with other proteins; for this reason, the regulation of tropomyosin binding is an important step in muscle contraction, as we discuss later (see Figure 16-74).
Another important actin-filament binding protein present in all eucaryotic cells is cofilin, which destabilizes actin filaments. Also called actin depolymerizing factor, cofilin is unusual in that it binds to actin in both the filament and free subunit forms. Cofilin binds along the length of the actin filament, forcing the filament to twist a little more tightly (Figure 16-34). This mechanical stress weakens the contacts between actin subunits in the filament, making the filament brittle and more easily severed. In addition, it makes it easier for an ADP-actin subunit to dissociate from the minus end of the filament. Because the rate of actin filament treadmilling is normally limited by the slow dissociation rate at the minus end, cofilin binding causes a large increase in the rate of actin filament treadmilling. As a result, most of the actin filaments inside cells are much shorter-lived than are filaments formed from pure actin in a test tube.

Figure 16-34
Twisting of an actin filament induced by cofilin. (A) Three-dimensional reconstruction from cryo-electron micrographs of filaments made of pure actin. The bracket shows the span of two turns of the actin helix. (B) Reconstruction of an actin filament (more...)
Cofilin binds preferentially to ADP-containing actin filaments rather than to ATP-containing filaments. Since ATP hydrolysis is usually slower than filament assembly, the newest actin filaments in the cell still contain mostly ATP and are resistant to depolymerization by cofilin. Cofilin therefore efficiently dismantles the older filaments in the cell, ensuring that all actin filaments turn over rapidly.
Proteins That Interact with Filament Ends Can Dramatically Change Filament Dynamics
As we have just seen, proteins that bind along the side of a filament can change the filament's dynamic behavior. For maximum effect, however, these proteins often need to coat the filament completely, and this means they have to be present at fairly high stoichiometries (for example, about one tropomyosin for every seven actin subunits, one tau for every four tubulin subunits, or one cofilin for every actin subunit). In contrast, proteins that bind preferentially to the ends of filaments can have dramatic effects on filament dynamics even when they are present at very low levels. Since subunit addition and loss occur primarily at filament ends, one molecule of such a protein per actin filament (typically one per about 200–500 actin monomers) can be enough to transform the architecture of an actin filament network.
As previously discussed, an actin filament that ceases elongation and is not specifically stabilized by the cell can depolymerize rapidly: it can lose subunits from either its plus or its minus end, once the actin molecules at that end have hydrolyzed their ATP to convert to the D form. The most rapid changes, however, occur at the plus end. An actin filament can be stabilized at its plus end by the binding of a plus end capping protein, which greatly slows the rates of both filament growth and filament depolymerization by making the plus end inactive (Figure 16-35). Indeed, most of the actin filaments in a cell are capped at their plus end by proteins such as CapZ (named for its location in the muscle Z band, see below; it is also called Capping Protein). At the minus end, an actin filament may be capped by remaining bound to the ARP complex that was responsible for its nucleation, although it is possible that many of the actin filament minus ends in typical cells are released from the ARP complex and are uncapped.

Figure 16-35
Filament capping and its effects on filament dynamics. A population of uncapped filaments adds and loses subunits at both the plus and minus ends, resulting in rapid growth or shrinkage, depending on the concentration of available free monomers (green (more...)
A local regulation of the level of capping helps cells to assemble and dismantle specific parts of their actin cytoskeleton. The association of capping proteins with actin filament ends is regulated by various localized intracellular signals. The regulation of plus end capping proteins by the inositol phospholipid PIP2 is especially important: an increase in PIP2 in the cytosolic leaflet of the plasma membrane caused by activation of an appropriate cell surface receptor (discussed in Chapter 15) uncaps plus ends. The uncapping makes the plus ends available for elongation, thereby promoting actin filament polymerization at the cell surface.
In muscle cells, where actin filaments are exceptionally long-lived, the filaments are known to be specially capped at both ends—by CapZ at the plus end and by tropomodulin at the minus end. Tropomodulin binds only to the minus end of actin filaments that have been coated by tropomyosin and have thereby already been somewhat stabilized.
The end of a microtubule, with thirteen protofilaments in a hollow ring (see Figure 16-6), is a much larger and more complex structure than the end of an actin filament, with many more possibilities for accessory protein action. We have already discussed an important microtubule capper: the γ-tubulin ring complex (γ-TuRC), which both nucleates the growth of microtubules at an organizing center and caps their minus ends. Another true capping protein for microtubules is the special protein complex found at the ends of the microtubules in cilia (discussed later), where microtubules are both stable and uniform in length.
Some proteins that act at the ends of microtubules have crucial roles beyond those expected for a simple capping protein. In particular, they can have dramatic effects on the dynamic instability of microtubules (see Figure 16-11). They can influence the rate at which a microtubule switches from a growing to a shrinking state (the frequency of catastrophes) or from a shrinking to a growing state (the frequency of rescues). For example, a family of kinesin-related proteins known as catastrophins induce catastrophes. They bind specifically to ends and seem to pry protofilaments apart, lowering the normal activation energy barrier that prevents a microtubule from springing apart into the curved protofilament characteristic of the shrinking state (see Figure 16-11C). Opposing their actions are MAPs that bind preferentially to ends to favor continued microtubule growth (Figure 16-36A). Both groups of proteins are regulated, and a greatly altered balance between their activities causes the major increase in microtubule turnover rates observed during mitosis (see Figure 18-12).

Figure 16-36
The effects of proteins that bind to microtubule ends. (A) The transition between microtubule growth and microtubule shrinking is controlled in cells by special proteins. A MAP such as XMAP215 stabilizes the end of a growing microtubule by its preferential (more...)
Proteins that bind to the ends of microtubules also serve to control microtubule positioning. In addition to γ-TuRC at the minus ends, there are several groups of plus-end-binding proteins that help localize the growing microtubule end to specific target proteins in the cell cortex. An end-binding protein present in both yeasts and humans, for example, is essential for yeast mitotic spindle positioning, directing the growing plus ends of yeast spindle microtubules to a specific docking region in the yeast bud and then helping to anchor them there (Figure 16-36B).
Filaments Are Organized into Higher-order Structures in Cells
So far, we have described how cells use accessory proteins to regulate the location and dynamic behavior of cytoskeletal filaments. These proteins can nucleate filament assembly, bind to the ends or sides of the filaments, or bind to the free subunits of filaments. But in order for the cytoskeletal filaments to form a useful intracellular scaffold that gives the cell mechanical integrity and determines its shape, the individual filaments must be organized and tied together into larger-scale structures. The centrosome is one example of such a cytoskeletal organizer; in addition to nucleating the growth of microtubules, it holds them together in a defined geometry, with all of the minus ends buried in the centrosome and the plus ends pointing outward. In this way, the centrosome creates the astral array of microtubules that is able to find the center of each cell (see Figure 16-25).
Another mechanism that is used to organize filaments into large structures is filament cross-linking. Any tendency of filaments to stick to one another causes them to align in parallel arrays so as to maximize interfilament contacts. As described earlier, some MAPs can bundle microtubules together: they have two domains—one that binds along the microtubule side (and thereby stabilizes the filament) and another that projects outward to contact other MAP-coated microtubules. In the actin cytoskeleton, the stabilizing and cross-linking functions are separated. Tropomyosin binds along the sides of actin filaments, but it does not have an outward projecting domain. Filament cross-linking is instead mediated by a second group of actin-binding proteins that have only this function. Intermediate filaments are different yet again; they are organized both by a lateral self-association of the filaments themselves and by the cross-linking activity of accessory proteins.
Intermediate Filaments Are Cross-linked and Bundled Into Strong Arrays
Each individual intermediate filament forms as a long bundle of tetrameric subunits (see Figure 16-16). Many intermediate filaments further bundle themselves by self-association; for example, the neurofilament proteins NF-M and NF-H contain a C-terminal domain that extends outward from the surface of the assembled intermediate filament and binds to a neighboring filament. Thus groups of neurofilaments form robust parallel arrays that are held together by multiple lateral contacts, giving strength and stability to the long cell processes of neurons (see Figure 16-20).
Other types of intermediate filament bundles are held together by accessory proteins, such as filaggrin, which bundles keratin filaments in differentiating cells of the epidermis to give the outermost layers of the skin their special toughness. Plectin, which makes bundles of vimentin, is a particularly interesting cross-linking protein. Besides bundling intermediate filaments, it also links the intermediate filaments to microtubules, actin filament bundles, and filaments of the motor protein myosin II (discussed below), as well as helping to attach intermediate filament bundles to adhesive structures at the plasma membrane (Figure 16-37). Mutations in the gene for plectin cause a devastating human disease that combines epidermolysis bullosa (caused by disruption of skin keratin filaments), muscular dystrophy (caused by disruption of desmin filaments), and neurodegeneration (caused by disruption of neurofilaments). Mice lacking a functional plectin gene die within a few days of birth, with blistered skin and abnormal skeletal and heart muscles. Thus, although plectin may not be necessary for the initial formation and assembly of intermediate filaments, its cross-linking action is required to provide cells with the strength they need to withstand the mechanical stresses inherent to vertebrate life.

Figure 16-37
Plectin cross-linking of diverse cytoskeletal elements. Plectin (green) makes cross-links from intermediate filaments (blue) to other intermediate filaments, to microtubules (red), and to myosin thick filaments. In this electron micrograph, the dots (more...)
Cross-linking Proteins with Distinct Properties Organize Different Assemblies of Actin Filaments
Actin filaments in animal cells are organized into two types of arrays: bundles and weblike (gel-like) networks (Figure 16-38). Actin filament cross-linking proteins are accordingly divided into two classes, bundling proteins and gel-forming proteins. Bundling proteins cross-link actin filaments into a parallel array, while gel-forming proteins hold two actin filaments together at a large angle to each other, thereby creating a looser meshwork. Both types of cross-linking protein generally have two similar actin-filament-binding sites, which can either be part of a single polypeptide chain or contributed by each of two polypeptide chains held together in a dimer. The spacing and arrangement of these two filament-binding domains determines the type of actin structure that a given cross-linking protein forms (Figure 16-39).

Figure 16-38
Actin arrays in a cell. A crawling cell, drawn to scale, is shown with three areas enlarged to show the arrangement of actin filaments. The actin filaments are shown in red, with arrowheads pointing toward the plus end. Stress fibers are contractile and (more...)

Figure 16-39
The modular structures of four actin-cross-linking proteins. Each of the proteins shown has two actin-binding sites (red) that are related in sequence. Fimbrin has two directly adjacent actin-binding sites, so that it holds its two actin filaments very (more...)
Fimbrin and α-actinin are widely distributed actin-bundling proteins. Fimbrin is a small crosslinker, with two actin-binding domains close together in a single polypeptide chain. It is enriched in the parallel actin filament bundles in filopodia at the leading edge of cells, and it is presumably responsible for the tight association of these actin filaments (Figure 16-40). α-actinin contains two actin-binding domains that are further apart (see Figure 16-40A and B); it is concentrated in stress fibers, where it is responsible for the relatively loose cross-linking of actin filaments in these contractile bundles. It also helps form the structure that holds stress fiber ends in focal contacts at the plasma membrane (see below).

Figure 16-40
The formation of two types of actin filament bundles. (A) α-actinin, which is a homodimer, cross-links actin filaments into loose bundles, which allow the motor protein myosin II (not shown) to participate in the assembly. Fimbrin cross-links (more...)
Each type of bundling protein determines which other molecules can interact with an actin filament. Myosin II (discussed later) is the protein in stress fibers and other contractile arrays that is responsible for their ability to contract. The very close packing of actin filaments caused by fimbrin apparently excludes myosin, and thus filopodia are not contractile; on the other hand, the looser packing caused by α-actinin allows myosin molecules to enter, making stress fibers contractile (see Figure 16-40A). Because of the very different spacing between the actin filaments, bundling by fimbrin automatically discourages bundling by α-actinin, and vice-versa, so that the two types of bundling protein are themselves mutually exclusive.
Villin is another bundling protein that, like fimbrin, has two actin-filament-binding sites very close together in a single polypeptide chain. Villin (together with fimbrin) helps cross-link the 20 to 30 tightly bundled actin filaments found in microvilli, the finger-like extensions of the plasma membrane on the surface of many epithelial cells (Figure 16-41). A single absorptive epithelial cell in the human small intestine, for example, has several thousand microvilli on its apical surface. Each is about 0.08 μm wide and 1 μm long, making the cell's absorptive surface area about 20 times greater than it would be without microvilli. When villin is introduced into cultured fibroblasts, which do not normally contain villin and have only a few small microvilli, the existing microvilli become greatly elongated and stabilized, and new ones are induced. The actin filament core of the microvillus is attached to the plasma membrane along its sides by lateral sidearms made of myosin I (discussed later), which has a binding site for filamentous actin on one end and a domain that binds lipids on the other end. These two types of cross-linkers, one binding actin filaments to each other and the other binding these filaments to the membrane, seem to be sufficient to form microvilli on cells.

Figure 16-41
A microvillus. (A) A bundle of parallel actin filaments cross-linked by the actin-bundling proteins villin and fimbrin forms the core of a microvillus. Lateral sidearms (composed of myosin I and the Ca2+-binding protein calmodulin) connect the sides of (more...)
The various bundling proteins that we have discussed so far have straight, stiff connections between their two actin-filament-binding domains, and they tend to align filaments in parallel bundles. In contrast, those actin cross-linking proteins that have either a flexible or a stiff, bent connection between their two binding domains form actin filament webs or gels, rather than actin bundles.
A well-studied web-forming protein is spectrin, which was first identified in red blood cells. Spectrin is a long, flexible protein made out of four elongated polypeptide chains (two α subunits and two β subunits), arranged so that the two actin-filament-binding sites are about 200 nm apart (compared with 14 nm for fimbrin and about 30 nm for α-actinin, see Figure 16-39). In the red blood cell, spectrin is concentrated just beneath the plasma membrane, where it forms a two-dimensional web held together by short actin filaments; spectrin links this web to the plasma membrane because it has separate binding sites for peripheral membrane proteins, which are themselves positioned near the lipid bilayer by integral membrane proteins (see Figure 10-31). The resulting network creates a stiff cell cortex that provides mechanical support for the overlying plasma membrane, allowing the red blood cell to spring back to its original shape after squeezing through a capillary. Close relatives of spectrin are found in the cortex of most other vertebrate cell types, where they also help to shape and stiffen the surface membrane.
Any cross-linking protein that has its two actin-binding domains joined by a long bent linkage can form three-dimensional actin gels. Filamin (see Figure 16-39) promotes the formation of a loose and highly viscous gel by clamping together two actin filaments roughly at right angles (Figure 16-42). The actin gels formed by filamin are required for cells to extend the thin sheet-like membrane projections called lamellipodia that help them to crawl across solid surfaces. Filamin is lacking in some types of cancer cells, especially some malignant melanomas (pigment-cell cancers). These cells cannot crawl properly, and instead they protrude disorganized membrane blebs (Figure 16-43). Losing filamin is bad news for the melanoma cells but good news for the melanoma patient; because of the cells' inability to crawl, melanoma cells that have lost filamin expression are less invasive than similar melanoma cells that still express filamin, and, as a result, the cancer is much less likely to metastasize.

Figure 16-42
Filamin cross-links actin filaments into a three-dimensional network with the physical properties of a gel. (A) Each filamin homodimer is about 160 nm long when fully extended and forms a flexible, high-angle link between two adjacent actin filaments. (more...)

Figure 16-43
Loss of filamin causes abnormal cell motility. (A) A group of melanoma cells that have an abnormally low level of filamin. These cells are not able to make normal lamellipodia and instead are covered with membrane “blebs.” As a result, (more...)
Severing Proteins Regulate the Length and Kinetic Behavior of Actin Filaments and Microtubules
In some situations, a cell may break an existing long filament into many smaller filaments. This generates a large number of new filament ends: one long filament with just one plus end and one minus end might be broken into dozens of short filaments, each with its own minus end and plus end. Under some intracellular conditions, these newly formed ends nucleate filament elongation, and in this case severing accelerates the assembly of new filament structures. Under other conditions, severing promotes the depolymerization of old filaments, speeding up the depolymerization rate by tenfold or more (Figure 16-44). In addition, severing filaments changes the physical and mechanical properties of the cytoplasm: stiff, large bundles and gels become more fluid when the filaments are severed.

Figure 16-44
Filament severing and its effects on filament dynamics. Normally, filaments are able to gain and lose subunits only at their ends, limiting the maximum rates of growth and shrinkage of the total amount of actin in filamentous form (green line). If the (more...)
To sever a microtubule, thirteen longitudinal bonds must be broken, one for each protofilament. The protein katanin, named after the Japanese word for “sword,” accomplishes this demanding task (Figure 16-45). Katanin is made up of two subunits, a smaller subunit that hydrolyzes ATP and performs the actual severing, and a larger one that directs katanin to the centrosome. Katanin releases microtubules from their attachment to a microtubule organizing center, and it is thought to have a particularly crucial role in the rapid microtubule depolymerization observed at the poles of a mitotic spindle during mitosis. It is also found in proliferating cells in interphase and in postmitotic cells such as neurons, where it may also be involved in microtubule release and depolymerization.

Figure 16-45
Microtubule severing by katanin. Taxol-stabilized, rhodamine-labeled microtubules were adsorbed on the surface of a glass slide, and purified katanin was added along with ATP. (A) There are a few breaks in the microtubules 30 seconds after the addition (more...)
In contrast to microtubule severing by katanin, which requires ATP, the severing of actin filaments does not require an extra energy input. Most actin-severing proteins are members of the gelsolin superfamily, whose severing activity is activated by high levels of cytosolic Ca2+. Gelsolin has subdomains that bind to two different sites on the actin subunit, one exposed on the surface of the filament and one that is normally hidden in the longitudinal bond to the next subunit in the protofilament. According to one model for gelsolin severing, gelsolin binds on the side of an actin filament and waits until a thermal fluctuation happens to create a small gap between neighboring subunits in the protofilament; gelsolin then insinuates its subdomain into the gap, breaking the filament (Figure 16-46). Once gelsolin has severed an actin filament, it remains bound to the plus end and acts as an effective capping protein. However, like several other actin filament capping proteins, it can be removed from the filament end by a local rise in PIP2 concentration.

Figure 16-46
A model for actin filament severing by gelsolin. There are two actin-binding sites in the gelsolin protein. These sites allow gelsolin to bind both along the side and at the end of an actin filament. Filaments that have been severed by gelsolin remain (more...)
The process of platelet activation shows how a cell can regulate its actin accessory proteins that mediate severing, capping, and cross-linking to generate rapid and dramatic morphological changes. Platelets are tiny cells without a nucleus that circulate in the blood and help to form clots at sites of injury. The resting platelet is discoid in shape, and it contains short actin filaments capped by CapZ, surrounded by a large pool of actin monomer bound to profilin. When the platelet is activated by physical contact with the edge of a damaged blood vessel or by a chemical clotting signal such as thrombin, a rapid, intracellular, signal transduction cascade results in a massive influx of Ca2+ into the platelet cytosol. The Ca2+ activates gelsolin, which cleaves the capped filaments into tiny fragments, each now capped by gelsolin. With slower kinetics, the same signaling pathway causes a rise in PIP2 levels, which inactivates both gelsolin and CapZ, removing them from the filament plus ends. The large numbers of free plus ends generated by severing and uncapping are then rapidly elongated by the monomeric actin pool, forming many long filaments. Some of these long actin filaments are cross-linked into a gel by filamin, while others are bundled by α-actinin and fimbrin. This causes the activated platelet to extend lamellipodia and filopodia and to spread itself across the clot (Figure 16-47), attaching to the clot by transmembrane adhesion proteins called integrins. Once the PIP2 signal subsides, the CapZ returns to the ends of the filaments, rendering them stable against depolymerization and locking the platelet into its spread form. Finally, myosin II uses ATP hydrolysis to slide the long actin filaments relative to one another, causing a contraction of the platelet that pulls the edges of the wound together.

Figure 16-47
Platelet activation. (A) Platelet activation is a controlled sequence of actin filament severing, uncapping, elongation, recapping, and cross-linking that creates a dramatic shape change in the platelet. (B) Scanning electron micrograph of platelets prior (more...)
Cytoskeletal Elements Can Attach to the Plasma Membrane
A common function of actin cytoskeletal structures is to stiffen or to change the shape of the plasma membrane. We have already encountered at least two examples: the spectrin-actin web that underlies the red blood cell plasma membrane and the villin-actin bundles in microvilli that enlarge the absorptive surface area of epithelial cells. The effectiveness of these structures depends on both the bundling and cross-linking of actin filaments and the specific attachments between the actin filament structures and proteins or lipids of the plasma membrane. In many cases, the cytoskeletal assemblies have the additional function of helping to connect the internal structure of a cell to its surrounding environment, including other cells and the extracellular matrix. Both actin filaments and intermediate filaments are critical for these connections. Here, we describe several types of specific interactions between cytoskeletal filaments and the transmembrane proteins that mediate cell adhesion. We consider only the intracellular side of these interactions; the extracellular interactions are discussed in Chapter 19.
A widespread family of closely related intracellular proteins, the ERM family (named for its first three members, ezrin, radixin, and moesin), acts to attach actin filaments to the plasma membrane in many cell types. The C-terminal domain of ERM proteins binds directly to the sides of actin filaments. The N-terminal domain binds to the cytoplasmic face of several transmembrane glycoproteins, including CD44, the receptor for the extracellular matrix component hyaluronan. The functional importance of the ERM proteins is indicated by the consequences of mutations that lead to a loss of one of the members of the family, called merlin. This results in one form of the human genetic disease called neurofibromatosis, in which multiple benign tumors develop in the auditory nerves and certain other parts of the nervous system.
Unlike the attachments between actin and the plasma membrane mediated by spectrin or myosin I, those mediated by ERM proteins are regulated by both intracellular and extracellular signals. ERM proteins can exist in two conformations, an active extended conformation that oligomerizes and binds actin and CD44, and an inactive folded conformation, in which the N- and C-termini are held together by an intramolecular interaction. Switching between the two conformations can be triggered by phosphorylation or binding to PIP2, either of which can occur, for example, in response to extracellular signals. Thus, the strength of ERM-mediated contacts between the actin cytoskeleton and the extracellular matrix is sensitive to a variety of signals received by the cell (Figure 16-48).

Figure 16-48
The role of ERM-family proteins in attaching actin filaments to the plasma membrane. Regulated unfolding of an ERM-family protein, caused by phosphorylation or by binding to PIP2, exposes two binding sites, one for an actin filament and one for a transmembrane (more...)
Special Bundles of Cytoskeletal Filaments Form Strong Attachments Across the Plasma Membrane: Focal Contacts, Adhesion Belts, and Desmosomes
Focal contacts are a highly specialized type of attachment between actin filaments and the extracellular matrix that allows cells to pull on the substratum to which they are bound. Focal contacts are particularly easy to see in cultured fibroblasts, because they create spots where the normal 50 nm gap between the bottom of the cell and the substratum is reduced to only 10–15 nm (Figure 16-49). At these sites, stress fibers, consisting of contractile bundles of actin and myosin II filaments, terminate at the plasma membrane, where clusters of transmembrane adhesion proteins, called integrins, are located. Integrins are a large family of heterodimeric proteins that bind to various components of the extracellular matrix (discussed in Chapter 19), and their linkage to the bundle of actin filaments is indirect, being mediated by an elaborate complex composed of multiple intracellular anchor proteins.

Figure 16-49
Focal contacts and stress fibers in a cultured fibroblast. (A) Focal contacts are best seen in living cells by reflection-interference microscopy. In this technique, light is reflected from the lower surface of a cell attached to a glass slide, and the (more...)
Besides serving as anchors for the cell, focal contacts can also relay signals from the extracellular matrix to the inside of the cell. Thus, the clustering of integrins at focal contacts activates a tyrosine kinase, focal adhesion kinase (FAK) (discussed in Chapter 19). Its activity is sensitive to the type of substratum on which the cell rests, and it is also regulated by the amount of tension at the cell attachment site. How this occurs is uncertain, but FAK is a target of the Src cytoplasmic tyrosine kinase (discussed in Chapter 5), which is also enriched at focal contacts. Once activated, FAK phosphorylates numerous targets, including many components of the focal contact complex itself, and it thereby helps to regulate the survival, growth, proliferation, morphology, movement, and differentiation of cells in response to the extracellular environment. Although we know few of the details, it is possible that the growth and development of a cell are influenced as much by signals received by means of its adhesion proteins as by the more extensively studied receptors for soluble, extracellular signal molecules described in Chapter 15.
Besides forming mechanically strong attachments to the extracellular matrix, cytoskeletal elements anchored to the plasma membrane also provide attachments to other cells. For example, the cell-cell contacts formed in epithelia are mediated largely by the interactions of transmembrane cadherin proteins (see Figure 19-24). The cytoplasmic tail of a cadherin molecule binds a complex of proteins called catenins, which in turn bind to actin filaments (see Figure 19-29). Clusters of these actin-reinforced, cadherin-mediated cell-cell contacts form the adherens junctions described in Chapter 19.
The general principle that we see in focal contacts and adherens junctions, in which bundles of cytoskeletal filaments are indirectly attached through elaborate multiprotein complexes to transmembrane adhesion proteins, is recapitulated in the attachment of intermediate filaments to the plasma membrane. Intermediate filaments are anchored to the plasma membrane at structures called desmosomes (at cell-cell junctions) and hemidesmosomes (at cell-extracellular matrix junctions). These cell junctions are especially important in maintaining the strength of epithelial tissues (discussed in Chapter 19).
Extracellular Signals Can Induce Major Cytoskeletal Rearrangements
In the preceding sections, we have seen how accessory proteins associated with cytoskeletal filament systems can regulate filament length, location, organization, and dynamic behavior. Extracellular signals can alter the activity of these accessory proteins, thereby changing the cytoskeleton and cell behavior.
For the actin cytoskeleton, global structural rearrangements in response to external signals are triggered through diverse cell-surface receptors. But all of these signals seem to converge inside the cell on a group of closely related monomeric GTPases that are members of the Rho protein family—Cdc42, Rac, and Rho. Like other members of the Ras superfamily, these Rho proteins act as molecular switches to control cellular processes by cycling between an active, GTP-bound state and an inactive, GDP-bound state (see Figure 3-70). Activation of Cdc42 triggers actin polymerization and bundling to form either filopodia or shorter cell protrusions called microspikes. Activation of Rac promotes actin polymerization at the cell periphery leading to the formation of sheet-like lamellipodial extensions and membrane ruffles. Activation of Rho promotes both the bundling of actin filaments with myosin II filaments into stress fibers and the clustering of integrins and associated proteins to form focal contacts (Figure 16-50). These dramatic and complex structural changes occur because each of these three molecular switches has numerous downstream target proteins that affect actin organization and dynamics (at least eight have been found for each).Other target proteins affect gene transcription.

Figure 16-50
The dramatic effects of Rac, Rho, and Cdc42 on actin organization in fibroblasts. In each case, the actin filaments have been labeled with fluorescent phalloidin, and focal contacts have been located with an antibody against vinculin. (A) Serum-starved (more...)
Some key targets of activated Cdc42 are members of the WASp protein family. WASp proteins, like ERM family proteins, can exist in an inactive folded conformation and an activated open conformation. Association with Cdc42-GTP stabilizes the open form of WASp, enabling it to bind to the ARP complex. This strongly enhances the actin-nucleating activity of the ARP complex (see Figure 16-28). Thus, activation of Cdc42 causes an increase in actin nucleation. Rac-GTP also activates WASp family members, but in addition it activates PI(4)P-5 kinase, which generates PI(4,5)P2 (a form of PIP2). As we have seen previously, PIP2 can cause the uncapping of filaments whose plus end is bound by gelsolin or CapZ, thereby providing even more sites for actin assembly near the plasma membrane, resulting in the formation of large lamellipodia and ruffles. Rho-GTP activates a protein kinase that inhibits a phosphatase acting on myosin light chains (see Figure 16-67). The consequent increase in the net amount of myosin light chain phosphorylation increases the amount of contractile myosin activity in the cell, enhancing the formation of tension-dependent structures such as stress fibers.
The mechanisms that turn on the three Rho protein family members are similarly complex. Their activation, through an exchange of GTP for GDP, is promoted by guanine nucleotide exchange factors (GEFs), of which more than 20 have been identified. Some are specific for an individual Rho family GTPase, whereas others seem to act on all three. Upstream from the GEFs are various cell-surface receptors. In most cases, the mechanisms that couple the receptors to GEF activation are unknown.
Summary
The varied forms and functions of cytoskeletal filament structures in eucaryotic cells depend on a versatile repertoire of accessory proteins. Each of the three major filament classes (microtubules, intermediate filaments, and actin filaments) has a large dedicated subset of such accessory proteins. A primary determinant of the sites of cytoskeletal structures is the regulation of the processes that initiate the nucleation of new filaments. In most animal cells, microtubules are nucleated at the centrosome, a complex assembly located near the center of the cell. In contrast, most actin filaments are nucleated near the plasma membrane.
The kinetics of filament assembly and disassembly can be either slowed or accelerated by accessory proteins that bind to either the free subunits or the filaments themselves. Some of these proteins alter filament dynamics by binding to the ends of filaments or by severing the filaments into smaller fragments. Another class of accessory proteins assembles the filaments into larger ordered structures by cross-linking them to one another in geometrically defined ways. Yet other accessory proteins determine the shape and adhesive properties of cells by attaching filaments to the plasma membrane. Cytoskeletal rearrangements are mediated by controlling the activities of various accessory proteins.
- Microtubules Are Nucleated by a Protein Complex Containing γ-tubulin
- Microtubules Emanate from the Centrosome in Animal Cells
- Actin Filaments Are Often Nucleated at the Plasma Membrane
- Filament Elongation Is Modified by Proteins That Bind to the Free Subunits
- Proteins That Bind Along the Sides of Filaments Can Either Stabilize or Destabilize Them
- Proteins That Interact with Filament Ends Can Dramatically Change Filament Dynamics
- Filaments Are Organized into Higher-order Structures in Cells
- Intermediate Filaments Are Cross-linked and Bundled Into Strong Arrays
- Cross-linking Proteins with Distinct Properties Organize Different Assemblies of Actin Filaments
- Severing Proteins Regulate the Length and Kinetic Behavior of Actin Filaments and Microtubules
- Cytoskeletal Elements Can Attach to the Plasma Membrane
- Special Bundles of Cytoskeletal Filaments Form Strong Attachments Across the Plasma Membrane: Focal Contacts, Adhesion Belts, and Desmosomes
- Extracellular Signals Can Induce Major Cytoskeletal Rearrangements
- Summary
- How Cells Regulate Their Cytoskeletal Filaments - Molecular Biology of the CellHow Cells Regulate Their Cytoskeletal Filaments - Molecular Biology of the Cell
- rfx4 regulatory factor X, 4 [Danio rerio]rfx4 regulatory factor X, 4 [Danio rerio]Gene ID:403016Gene
Your browsing activity is empty.
Activity recording is turned off.
See more...