By agreement with the publisher, this book is accessible by the search feature, but cannot be browsed.
NCBI Bookshelf. A service of the National Library of Medicine, National Institutes of Health.
Purves D, Augustine GJ, Fitzpatrick D, et al., editors. Neuroscience. 2nd edition. Sunderland (MA): Sinauer Associates; 2001.
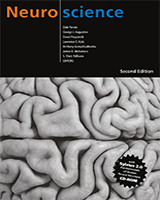
Neuroscience. 2nd edition.
Show detailsAlthough Hodgkin and Huxley had no knowledge of the physical nature of the conductance mechanisms underlying action potentials, they nonetheless proposed that nerve cell membranes have channels that allow ions to pass selectively from one side of the membrane to the other (see Chapter 3). Based on the ionic conductances and currents measured in voltage clamp experiments, the postulated channels had to have several properties. First, because the ionic currents are quite large, the channels had to be capable of allowing ions to move across the membrane at high rates. Second, because the ionic currents depend on the electrochemical gradient across the membrane, the channels had to make use of these gradients. Third, because Na+ and K+ flow across the membrane independently of each other, different channel types had to be capable of discriminating between Na+ and K+, allowing only one of these ions to flow across the membrane under the relevant conditions. Finally, given that the conductances are voltage-dependent, the channels had to be able to sense the voltage drop across the membrane, opening only when the voltage reached appropriate levels. While this concept of channels was highly speculative in the 1950s, later experimental work established beyond any doubt that transmembrane proteins called voltage-sensitive ion channels indeed exist and are responsible for all of the ionic conductance phenomena described in Chapter 3.
The first direct evidence for the presence of voltage-sensitive, ion-selective channels in nerve cell membranes came from measurements of the ionic currents flowing through individual ion channels. The voltage-clamp apparatus used by Hodgkin and Huxley could only resolve the aggregate current resulting from the flow of ions through many thousands of channels. A technique capable of measuring the currents flowing through single channels was devised in 1976 by Erwin Neher and Bert Sakmann at the Max Planck Institute in Goettingen. This remarkable approach, called patch clamping (Box A), revolutionized the study of membrane currents. In particular, the patch clamp method provided the means to test directly Hodgkin and Huxley's proposals about the characteristics of ion channels.
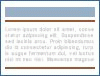
Box A
The Patch Clamp Method.
Currents flowing through Na+ channels are best examined in experimental circumstances that prevent the flow of current through other types of channels that are present in the membrane (e.g., K+ channels). Under such conditions, depolarizing a patch of membrane from a squid giant axon causes tiny inward currents to flow, but only occasionally (Figure 4.1). The size of these currents is minuscule—approximately l-2 pA (i.e., 10-12 ampere), which is orders of magnitude smaller than the Na+ currents measured by voltage clamping the entire axon. The currents flowing through single channels are called microscopic currents to distinguish them from the macroscopic currents flowing through a large number of channels distributed over a much more extensive region of surface membrane. Although microscopic currents are certainly small, a current of 1 pA nonetheless reflects the flow of thousands of ions per millisecond. Thus, as predicted, a single channel can let many ions pass through the membrane in a very short time.

Figure 4.1
Patch clamp measurements of ionic currents flowing through single Na+ channels in a squid giant axon. In these experiments, Cs+ was applied to the axon to block voltage-gated K+ channels. Depolarizing voltage pulses (A) applied to a patch of membrane (more...)
Several observations further proved that the microscopic currents in Figure 4.1B are due to the opening of single, voltage-activated Na+ channels. First, the currents are carried by Na+; thus, they are directed inward at potentials more negative than ENa, reverse their polarity at ENa, are outward at more positive potentials, and are reduced in size when the Na+ concentration of the external medium is decreased. This behavior exactly parallels that of the macroscopic Na+ currents described in Chapter 3. Second, the channels have a time course of opening, closing, and inactivating that matches the kinetics of macroscopic Na+ currents. This correspondence is difficult to appreciate in the measurement of microscopic currents flowing through a single open channel, because individual channels open and close in a stochastic (random) manner, as can be seen by examining the individual traces in Figure 4.1B. However, repeated depolarization of the membrane potential causes each Na+ channel to open and close many times. When the current responses to a large number of such stimuli are averaged together, the collective response has a time course that looks much like the macroscopic Na+ current (Figure 4.1C). In particular, the channels open mostly at the beginning of a prolonged depolarization, showing that they subsequently inactivate, as predicted from the macroscopic Na+ current (compare Figures 4.1C and 4.1D). Third, both the opening and closing of the channels are voltage-dependent; thus, the channels are closed at -80 mV but open when the membrane potential is depolarized. In fact, the probability that any given channel will be open varies with membrane potential (Figure 4.1E), again as predicted from the macroscopic Na+ conductance (see Figure 3.7). Finally, tetrodotoxin, which blocks the macroscopic Na+ current (see Box C), also blocks microscopic Na+ currents. Taken together, these results show that the macroscopic Na+ current measured by Hodgkin and Huxley does indeed arise from the aggregate effect of many thousands of microscopic Na+ currents, each representing the opening of a single voltage-sensitive Na+ channel.
Patch clamp experiments have also revealed the properties of the channels responsible for the macroscopic K+ currents associated with action potentials. When the membrane potential is depolarized (Figure 4.2A), microscopic outward currents (Figure 4.2B) can be observed under conditions that block Na+ channels. The microscopic outward currents exhibit all the features expected for currents flowing through action-potential-related K+ channels. Thus, the microscopic currents (Figure 4.2C), like their macroscopic counterparts (Figure 4.2D), fail to inactivate during brief depolarizations. Moreover, these single-channel currents are sensitive to ionic manipulations and drugs that affect the macroscopic K+ currents and, like the macroscopic K+ currents, are voltage-dependent (Figure 4.2E). This and other evidence shows that macroscopic K+ currents associated with action potentials arise from the opening of many voltage-sensitive K+ channels.

Figure 4.2
Patch clamp measurements of ionic currents flowing through single K+ channels in a squid giant axon. In these experiments, tetrodotoxin was applied to the axon to block voltage-gated Na+ channels. Depolarizing voltage pulses (A) applied to a patch of (more...)
In summary, patch clamping has allowed direct observation of microscopic ionic currents flowing through single ion channels, confirming that voltage sensitive Na+ and K+ channels are responsible for the macroscopic conductances and currents that underlie the action potential. Measurements of the behavior of single ion channels also indicate many of the molecular attributes of these channels. For example, they show that the membrane of the squid axon contains at least two types of channels—one selectively permeable to Na+ and a second selectively permeable to K+. Both channel types are voltage-gated, meaning that their opening is influenced by membrane potential (see Figures 4.1 and 4.2). For each channel, depolarization increases the probability of channel opening, whereas hyperpolarization closes them. Thus, both channel types have a voltage sensor that detects the membrane potential (Figure 4.3). However, these channels differ in important respects. In addition to their different ion selectivities, depolarization also inactivates the Na+ channel but not the K+ channel, causing Na+ channels to pass into a nonconducting state. The Na+ channel must therefore have an additional molecular mechanism responsible for inactivation. And, as expected from the macroscopic behavior of the Na+ and K+ currents described in Chapter 3, the kinetic properties of the gating of the two channels differs. This detailed information about the physiology of single channels set the stage for subsequent studies of the molecular characteristics of ion channels.

Figure 4.3
Functional states of voltage-gated Na+ and K+ channels. The gates of both channels are closed when the membrane potential is hyperpolarized. When the potential is depolarized, voltage sensors (indicated by +) allow the channel gates to open—first (more...)
- PubMedLinks to PubMed
- Ion Channels Underlying Action Potentials - NeuroscienceIon Channels Underlying Action Potentials - Neuroscience
Your browsing activity is empty.
Activity recording is turned off.
See more...